DOI:
10.1039/C8QM00488A
(Research Article)
Mater. Chem. Front., 2019,
3, 420-428
Development and reactive oxygen-species scavenging activity of a new chemical hydrogen-generating system, CaMg2-hydroxypropyl cellulose-citric acid, prepared using Laves-phase CaMg2 and its relationship to chemical hardness
Received
25th September 2018
, Accepted 26th December 2018
First published on 27th December 2018
Abstract
We developed a new chemical hydrogen-generating CaMg2-hydroxypropyl cellulose-citric acid (CAMGCC) system from Laves-phase CaMg2 by using an arc melting method. The CAMGCC generated hydrogen gas (H2) rapidly for 2–3 min on the addition of water. Moreover, the CAMGCC system could scavenge reactive oxygen species (ROS), such as toxic hydroxyl radicals (˙OH) and superoxides (O2−˙) effectively. To develop a new chemical hydrogen-generating system that generates H2 efficiently, it is essential to calculate the quantity (ΔQ) of electron transfer from metal and alloy to H2O by using the absolute hardness (η) and absolute electronegativity (χ) based on chemical hardness. Metals and alloys with a large amount of calculated ΔQ can be used as excellent materials to develop a chemical hydrogen-generating system. A larger ΔQ of electron transfer from the metal to H2O results in a greater antioxidant activity of the system. The results were supported by using the calculation results for clusters of CaMg2 and Mg3, instead of crystalline CaMg2 and Mg. These studies are important in the development of chemical hydrogen-generating systems and antioxidants.
Introduction
Chemical hydrogen-storage systems or hydrogen-absorbing alloys have attracted a great deal of attention as new technologies for generating molecular hydrogen (H2). As an antioxidant, H2 has high potential for use in the prevention and medical treatment of diseases and it is a known alternative energy source.1–5 At the laboratory level, investigations have been conducted to determine a method for the efficient generation of H2 under mild (i.e., physiological) conditions. Magnesium (Mg) has attracted the attention of chemists from various fields for use in chemical hydrogen-storage alloys because Mg alloys exhibit an oxidation resistance and burning resistance6 and can absorb 3–6% of their mass of H2.7–9 However, temperatures of 200–500 °C are required to desorb H2 from hydrogen-storage alloys, and therefore, such hydrogen-storage alloys cannot be used to develop antioxidants or to reduce oxidative stress at the living-cell level, which is the aim of our investigation. Therefore, we have focused on developing a chemical hydrogen-generating system that operates in H2O rather than a Mg-based hydrogen-storage alloy or system.10 It is difficult to use a Mg/H2O system as an antioxidant to scavenge toxic hydroxyl radicals (˙OH) and as a chemical hydrogen-generating system because the production of H2 in the reaction Mg + 2H2O → Mg2+ + 2OH− + H2 is very slow in H2O at room temperature.
In our most recent study, we developed a powder Mg–hydroxypropyl cellulose-citric acid (MGCC) as a chemical hydrogen-generating system using powdered Mg coated with hydroxypropyl cellulose (HPC), together with citric acid.10 The production of H2 from MGCC in H2O is far superior to that by Mg in H2O because of the solid-acid effect of citric acid on the Mg surface. We proposed a mechanism for H2 production from the MGCC system. We also explored the development of a chemical hydrogen-generating system using soft Ca, but found it difficult and inefficient. Here, we focused on the use of Laves-phase Ca–Mg (CaMg2) alloys because Laves-phase alloys are a crystal family that is known to exhibit a wide variety of physical, chemical, and magnetic properties, including hydrogen absorption into these materials. It has been reported that CaMg2 alloys absorb H2 under 10 MPa of H2 at 240 °C. However, hydrogenated CaMg2 only shows the desorption of H2 gases above 480 °C.9 Therefore, hydrogenated CaMg2 alloys are impractical for use as antioxidants to reduce the oxidative stress of living cells.
We developed a new hydrogen-generating CaMg2-hydroxypropyl cellulose-citric acid (CAMGCC) system by using Laves-phase CaMg2 with a method similar to that described in our previous paper.10 The CAMGCC equivalent to MGCC generated approximately three times the volume of H2 by the addition of H2O than that by MGCC. The antioxidant activities of CAMGCC were determined from the IC50 values that were obtained by a scavenging assay of superoxide anion radicals (O2−˙) and hydroxyl radicals (˙OH) that were generated by the xanthine oxidase (XOD) method11 and H2O2 photolysis,12–14 respectively. The antioxidant activities of CAMGCC were stronger than those of MGCC. We found that the antioxidant potentials of CAMGCC and MGCC are correlated to the quantity (ΔQ) of electron transfer from CaMg2 and Mg to H2O by using the chemical hardness theory. The large ΔQ of electron transfer from Laves-phase AB2 to H2O has a strong antioxidant activity. These results were also supported by calculated data based on the chemical hardness by using the density functional theory (DFT) method with clusters of CaMg2 and Mg3.15 We report that it is possible to develop a new chemical hydrogen-generating system by using chemical hardness.
Results and discussion
Preparation of Laves-phase CaMg2 and chemical hydrogen-generating system CAMGCC
The chemical hydrogen-generating system, CAMGCC, which generated hydrogen gas (H2) efficiently, was prepared by using Laves-phase CaMg2 alloy that was prepared by the arc melting of Mg with Ca. After arc melting, the Ca–Mg alloy was made into a fine powder by crushing with a pestle in a porcelain mortar. Fig. 1A and B show the XRD bands of anhydrous citric acid (▲) and powder Mg (●). Fig. 1C shows the powder X-ray diffraction (XRD) bands of the Ca–Mg alloy that was produced by using arc melting. It is thought that a Ca–Mg alloy of uniform composition was produced because the XRD band that was derived from Mg of 2θ = 36.5° was not observed in Fig. 1C. Moreover, the bands were identified as the diffraction peaks (○) of the CaMg2 alloy that was indexed to the AB2 Laves phase by comparison with the XRD profiles of the Laves-phase CaMg2.8,9 It was suggested that the single nanoparticles of the Ca–Mg alloy that were obtained from the chamber by the arc plasma method had broad XRD bands that were not identical to the XRD bands of the Laves-phase CaMg2. This means that the fine particles were mixtures that contained Laves-phase and other phase structures.
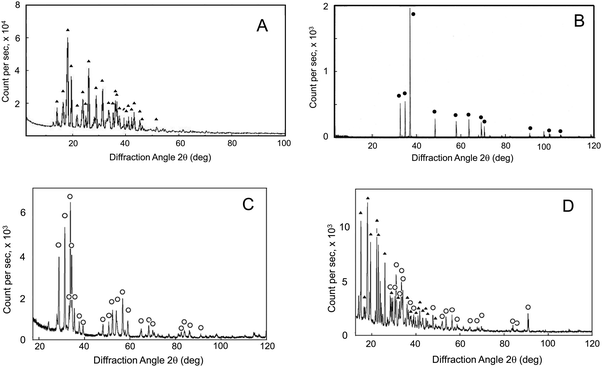 |
| Fig. 1 X-ray powder diffraction (XRD) profiles for citric acid, CaMg2, and CAMGCC systems. The XRD patterns of A, B, C, and D are for citric acid, Mg, CaMg2, and CAMGCC, respectively. CaMg2 (open circles) and citric acid (closed triangles). | |
The new chemical hydrogen-generating system CAMGCC was prepared with hydroxypropyl cellulose (HPC)-coated Laves-phase CaMg2 (powder) with anhydrous citric acid according to the method described in the experimental section. The XRD bands of the powder CAMGCC system were identified as the diffraction peaks of CaMg2 (○) and citric acid (▲) as shown in Fig. 1D.
Generation of H2 with chemical hydrogen-generating system, CAMGCC
The generation of H2 from the CAMGCC system was identified by using a 6890N network model gas-chromatography system that was connected to a JMS-700 model mass spectrometer. Fig. 2 shows the gas chromatography–mass spectrometry (GC–MS) profiles of gases that were generated from the CAMGCC system by using a gas-over-water technique in degassed distilled H2O. The product ratio of the generated compounds was determined with high sensitivity from the height and area of the peaks measured using SIM (Selected Ion Monitoring) mode. In Fig. 2, the x-axis represents the retention time (min), and the vertical axis represents the intensity. The data show that H2 was a major product and hydrogen deuteride (HD) was generated from the CAMGCC system at a volume ratio of 1.22% against H2 because the peak areas of H2 (m/z 2.0157) and HD (m/z 3.0219) were 219.15 and 2.68, respectively. Gaseous D2 (m/z 4.02804) was generated simultaneously and the volume ratio was 0.00456%.
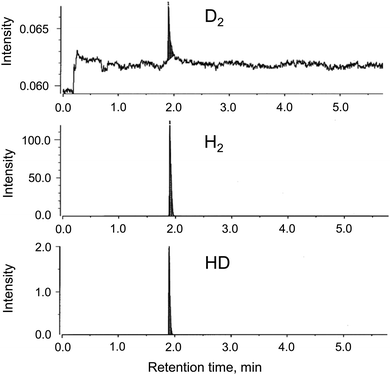 |
| Fig. 2 Identification by gas chromatography–mass spectrometry (GC–MS) analysis of HD, H2, and D2 generated from a CAMGCC system in H2O. GC–MS of gases generated from CAMGCC powders (0.5 g) by the gas-over-water technique. Peak intensity of each compound was measured using SIM mode. | |
Although the reaction between Mg and H2O occurs as shown by Mg + 2H2O → Mg2+ + 2OH− + H2, H2 is not generated easily at room temperature in H2O. To compare the H2 generation by the CAMGCC system with that of other materials, the volumes of H2 generated from powder Mg, powder Laves-phase CaMg2, MGCC, and CAMGCC in H2O were quantified by using the gas-over-water technique. The results are shown in Fig. 3. When 20 mL of H2O was added to 0.024 g (0.27 mmol) of Laves-phase CaMg2, 0.1 mL of H2 was generated in 3 min. However, when 20 mL of H2O was added to 0.21 g (0.27 mmol) of CAMGCC, about 3.8 mL of gaseous H2 was generated as fine bubbles in 3 min. The CAMGCC equivalent to MGCC generated about 3 times the volume of H2 by the addition of H2O as shown in Fig. 3d. The volumes of H2 generation in the reaction of CaMg2 (in CAMGCC) with H2O are related to the stoichiometry.
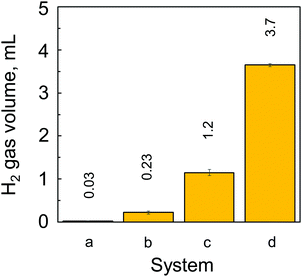 |
| Fig. 3 Measured volume of H2 generated from Mg (a), CaMg2 (b), MGCC (c), and CAMGCC (d) systems by using the gas-over-water technique. Equimolar (0.27 mmol) amounts of Mg (a), CaMg2 (b), MGCC (c), and CAMGCC (d). Equivalent amounts (0.210 g) of CAMGCC and MGCC. Data are expressed as means ± SEs (standard errors, n = 3). | |
The results show that the volume of H2 generated in 3 min by an equivalent chemical hydrogen-generating system increases as: Mg < CaMg2 ≪ MGCC < CAMGCC.
Antioxidant activity of chemical hydrogen-generating system, CAMGCC
Hydroxyl radicals.
˙OH radicals tend to be generated by well-known methods, such as the Fenton system (Fe(II) or Cu(I)/H2O2),16 H2O radiation,17 and H2O2 photolysis.13,14 In the photolysis of H2O2, 1 mol of H2O2 readily forms 2 mol of ˙OH radicals (eqn (1)).18 Therefore, we obtained dose–response curves by the scavenging of CAMGCC with ˙OH from H2O2 by irradiation for 60 s at λ 280–315 nm in the dark. The half-maximal (50%) inhibitory concentrations determined from the dose–response curves were used as the antioxidant activity (IC50) of the CAMGCC system. | H2O2 + UV ⇄ [˙OH + ˙OH]solvent → 2˙OH | (1) |
The hydroxyphenyl fluorescein (HPF) that was oxidized by ˙OH fluoresces strong signals that absorb an excitation light of λ 490 nm. Importantly, the stock solution of CAMGCC cannot be prepared in advance because CAMGCC generates H2 in water solution. Therefore, each emission intensity of HPF was measured by adding CAMGCC in a mass range between 0 and 13 mg (in 400 μL) in five-surface transparent quartz cells. Fig. 4A shows the dose–response curves of the HPF responses that were obtained by the scavenging reaction of CAMGCC with ˙OH. The x-axis shows the concentration of CAMGCC and the y-axis shows the relative fluorescence intensity (F). The IC50 represents the 50% fluorescence intensity point of the dose–response curve of the F response for various concentrations of CAMGCC. The antioxidant activities of MGCC and Trolox (6-hydroxy-2,5,7,8-tetramethylchroman-2-carboxylic acid) were compared with those of CAMGCC under similar conditions because Trolox has been used as an antioxidant standard.19 The results are shown in Fig. 4B and C. The ˙OH scavenging activities of CAMGCC, MGCC, and Trolox were 4.2 (±0.262), 5.0 (±0.225) (±SE), and 1.25 × 10−4 mg mL−1, respectively. The values of IC50 (means) are summarized in Fig. 4D. The small bars represent standard errors (SE). The strength of the antioxidant activity increased as: MGCC < CAMGCC ≪ Trolox. Excess ˙OH that was generated by H2O2 photolysis is scavenged by H2 that is generated from CAMGCC in H2O (Fig. 4E). The reaction mechanism is given by the reaction H2 + 2˙OH → 2H2O.
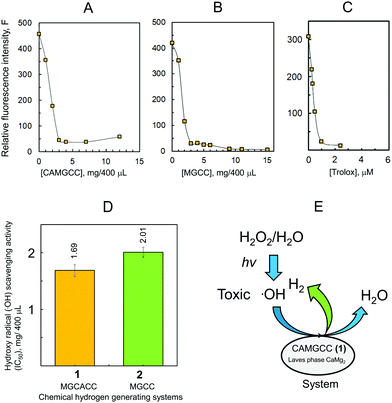 |
| Fig. 4 Dose–response curves of CAMGCC (A) and MGCC (B) for hydroxyl-radical (˙OH) scavenging activity and the IC50 diagram (D). Responses are expressed as the intensity of fluorescence for the concentration (mg/400 μL) of CAMGCC (A), MGCC (B), and Trolox (C) in 10 mM phosphate buffer (pH 7.4). (D) IC50 values are converted to values for 1 mL. Data are expressed as means ± SEs (n = 3). (E) Model of scavenging reaction by the reaction of toxic ˙OH formed by photolysis with H2 generated from CAMGCC in H2O. | |
Superoxide anion radicals.
The scavenging activity of the superoxide anion radical (O2−˙) was determined from the change in chemiluminescence (FCL) response by the reaction of MPEC with the O2−˙ produced by the XOD-HPX method.20 In the O2−˙ scavenging reaction, the control value (FCL) was 5–7 × 106 (RLU). Fig. 5 shows the dose–response curves for O2−˙ scavenging measured at 6–7 points of a mass range between 0 and 14 mg (in 400 μL) of CAMGCC (A) and MGCC (B). In the series of measurements, the O2−˙ scavenging activities of CAMGCC and MGCC were 1.68 (±0.089) and 2.97 (±0.88) (±SE) mg mL−1, respectively. The results are summarized in Fig. 5C. In comparison with CAMGCC, Trolox was 0.0166 (±0.00375) mg mL−1 (= 6.63 × 10−5 ± 1.5 × 10−5 (±SE) mol L−1). The antioxidant activity strength increased as: MGCC < CAMGCC < Trolox. When using Mg3(citric acid)2 instead of CAMGCC, the O2−˙ scavenging reaction did not proceed. Therefore, we propose that the active species in the CAMGCC system is H2 that is generated from CAMGCC in H2O (Fig. 5D).
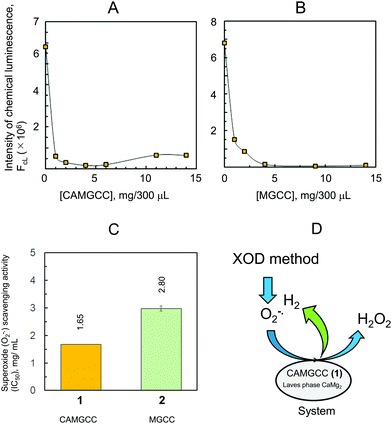 |
| Fig. 5 Dose–response curves for superoxide anion-radical (O2−˙) scavenging of CAMGCC (A) and MGCC (B) and the IC50 diagram (C). Responses are expressed as the intensity of chemiluminescence for the concentration (mg/300 μL) of CAMGCC and MGCC in 10 mM phosphate buffer (pH 7.4). MPEC is used as a chemiluminescent reagent. Data are expressed as means ± SEs (n = 4). (D) Model of ROS scavenging by the reaction of O2−˙ (formed by XOD/HPX method) with H2 (generated from CAMGCC in 0.1 M phosphate buffer). | |
Crystal structure of Laves-phase CaMg2 and structural model of the CaMg2 cluster
The experimental lattice parameters and space groups of the Laves-phase CaMg2 (3) and Mg (4) that were used to prepare the CAMGCC and MGCC systems were taken from the literature,21–23 and the CaMg2 and Mg structures were prepared by using VESTA and Spartan’16 as shown in Fig. 6. Fig. 7A and B show the energy levels and the highest occupied molecular orbital (HOMO) and lowest unoccupied molecular orbital (LUMO) phases of Laves-phase 3 calculated using the B3LYP method with 6-311+G(2df,2p) as the basis set. The energy, εhomo, εlumo, absolute hardness (η), and absolute electronegativity (χ) of 3 and 4 are listed in Table 1. The εhomo (−3.37 eV) of Laves-phase 3 is higher than that (−3.41 eV) of crystal Mg 4. Crystal 3 from eqn (5) is a harder crystal chemically than crystal 4, and the absolute electronegativity (χ = 3.020) of 3 is lower than that (χ = 3.170) of 4. That is, the Laves phase of 3 is more easily oxidized than crystal 4. Fig. 7C shows the energy levels and the HOMO and LUMO phases of crystal Mg 4 that were computed by using the B3LYP/and M06/6-311+G(2df,2p) methods. The HOMO and LUMO phases of CaMg23 are distributed on the Ca and two Mg atoms that are represented by ▲ in the crystal. The HOMO phase of Mg 4 is distributed on the three Mg atoms represented by ▲ in the crystal. The HOMO phase of Laves-phase 3 is distributed in the Ca of ▲ as shown in Fig. 6 and 7A and B.
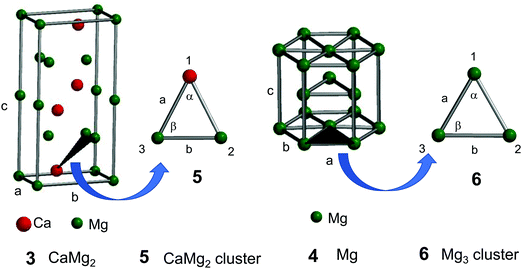 |
| Fig. 6 Crystal structures of Laves-phase CaMg2 (3) and Mg (4) and structural models of the CaMg2 cluster (5) and Mg3 cluster (6). For a detailed description of 3 and 4, see Fig. 7A and B. Models of clusters 5 and 6 are represented by ▲ in the structures of 3 and 4. | |
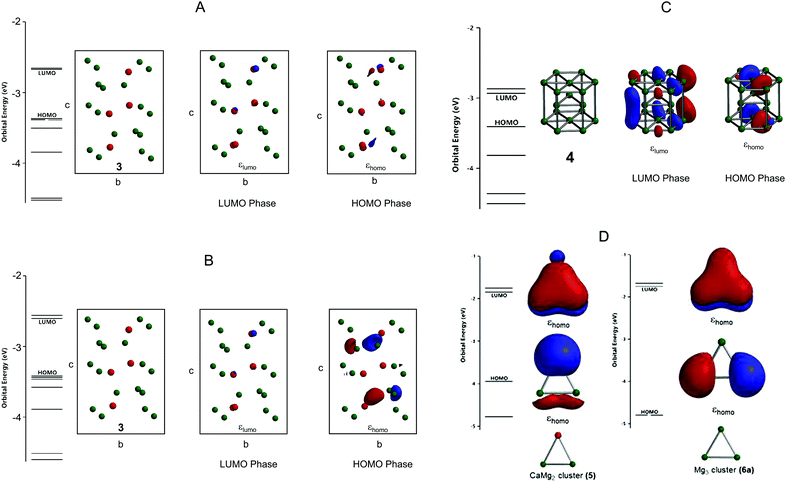 |
| Fig. 7 Orbital energy diagram and the HOMO and LUMO phases of Laves-phase CaMg2 (A and B), crystal Mg (C), CaMg2, and Mg3 clusters (D). (A and B) Single-point orbital energy, εhomo, and εlumo of CaMg2 computed at the B3LYP/6-311+G(2df,2p) and M06/6-311+G(2df,2p) level, respectively. The structural parameters of CaMg2 are a = b = 6.220 Å, c = 10.100 Å, α = β = 90°, and γ = 120°. (C) Orbital energy, εhomo, and εlumo of crystal Mg computed at the B3LYP/6-311+G(2df,2p) level. (D) Orbital energy, εhomo, and εlumo for clusters of CaMg2 and Mg3 optimized using the B3LYP/6-311+G(2df,2p) method. | |
Table 1 Energy, εhomo, εlumo, absolute hardness and electronegativity of Laves phase CaMg2 and Mg computed using the DFT method
|
Distancea |
Energyb (eV) |
ε
homo (eV) |
ε
lumo (eV) |
Absolute hardness (η, eV) |
Absolute electronegativity (χ, eV) |
a (Å) |
b (Å) |
c (Å) |
a, b, and c present crystal parameters.
Single-point calculation.
Optimized energy.
|
CaMg2 (3) |
6.220 |
6.220 |
10.100 |
|
|
|
|
|
B3LYP/6-311+G(2df,2p) |
|
|
|
−171764.59 |
−3.37 |
−2.67 |
0.3505 |
3.020 |
6-311+G(d,p) |
|
|
|
−171764.22 |
−3.38 |
−2.69 |
0.345 |
3.035 |
M06/6-311+G(2df,2p) |
|
|
|
−171747.93 |
−3.42 |
−2.60 |
0.410 |
3.010 |
|
Mg (4) |
3.209 |
3.209 |
5.211 |
|
|
|
|
|
B3LYP/6-311+G(2df,2p) |
|
|
|
−92550.01 |
−3.41 |
−2.93 |
0.240 |
3.170 |
6-311+G(d,p) |
|
|
|
−92549.63 |
−3.44 |
−2.74 |
0.233 |
3.090 |
M06/6-311+G(2df,2p) |
|
|
|
−92541.90 |
−3.51 |
−2.67 |
0.420 |
3.090 |
|
H2O |
6-311+G(2df,2p) |
|
|
|
−2080.67c |
−8.82 |
0.56 |
4.69 |
4.13 |
We present the cluster of CaMg2 (5) and Mg3 (6) shown as ▲ in crystals 3 and 4 in Fig. 6. Fig. 7D shows the optimized structures, the HOMO and LUMO phases, and energy levels of clusters 5 and 6. The calculated data are listed in Table 2. The results show that 6 has two energetically stable structures, 6a and 6b. The difference of the energies of 6a and 6b calculated by B3LYP/6-311+G(2df,2p) is small; the energies are −16334.72 (6a) and −16334.58 eV (6b). The bond lengths, Mg–Mg, of 6a and 6b are 3.44 and 6.74 Å, respectively. The binding energy of 5 is 0.216 eV, a value that is obtained by subtracting the basis set superposition error (BSSE24). The HOMO is distributed in the Ca of the CaMg2 cluster 5 (Fig. 7B). From Table 2, the absolute electronegativity of the CaMg2 cluster 5 is lower than that of Mg cluster 6a; the electron-withdrawing property of the Mg cluster 6a is stronger than that of the CaMg2 cluster 5. Furthermore, cluster 5 (η = 1.060) with a small HOMO–LUMO gap has a higher reactivity than 6a (η = 1.515) with a large hardness.
Table 2 Energy, εhomo, εlumo, absolute hardness and electronegativity of CaMg2 and Mg3 clusters computed using the DFT method
|
Distance |
Bond angle |
Energya (eV) |
ε
homo (eV) |
ε
lumo (eV) |
Absolute hardness (η, eV) |
Absolute electronegativity (χ, eV) |
a (Å) |
b (Å) |
(α) |
(β) |
|
(degree) |
Optimized energy.
|
CaMg2 cluster (5) |
B3LYP/6-311+G(2df,2p) |
3.667 |
3.349 |
54.3 |
62.8 |
−29327.93 |
−3.96 |
−1.84 |
1.060 |
2.900 |
M06/6-311+G(2df,2p) |
3.510 |
3.201 |
54.2 |
62.9 |
−29325.14 |
−4.02 |
−1.79 |
1.115 |
2.905 |
|
Mg3 cluster (6a) |
B3LYP/6-311+G(2df,2p) |
3.44 |
3.44 |
60.0 |
60.0 |
−16334.72 |
−4.79 |
−1.76 |
1.515 |
3.280 |
M06/6-311+G(2df,2p) |
3.237 |
3.237 |
60.0 |
60.0 |
−16332.15 |
−4.76 |
−1.74 |
1.510 |
3.250 |
|
Mg3 cluster (6b) |
B3LYP/6-311+G(2df,2p) |
6.74 |
6.74 |
60.0 |
60.0 |
−16334.58 |
−5.26 |
−1.17 |
2.045 |
3.215 |
Electron transfer to H2O from CaMg2 and Mg
The CAMGCC scavenges ˙OH radicals, and we found that the antioxidant activity of CAMGCC is stronger than that of MGCC as shown in Fig. 4 and 5. We explored why the antioxidant activity of CAMGCC is stronger than that of MGCC. The difference in reactivity of CaMg23 and Mg 4 is derived from the quantity of electron transfer (ΔQ) based on chemical hardness as shown in eqn (6), because H2 generation by the reaction of CAMGCC with H2O is a redox reaction. Tables 1 and 2 show the values of the absolute hardness (η) and absolute electronegativity (χ) of the crystal models 3 and 4.
An electron is transferred from Laves-phase CaMg23 to H2O because the ΔQ value is negative (−0.11) according to the approximation of eqn (6). Similarly, the ΔQ of crystal Mg 4 is −0.097. The magnitude of ΔQ is greater for 3 than for 4. The results suggest that the reductive power of 3 is stronger than 4. The difference in ΔQ values of 3 > 4 for electron transfer also suggests that the antioxidant activity of the CAMGCC system is stronger than that of the MGCC system. The ΔQ of the electrons from crystals 3 (and 4) to H2O is equivalent to systems 1 (and 2). To test the above results, the ΔQ of the electrons from clusters 5 and 6a to H2O were calculated and the results are listed in Table 3. The magnitude of ΔQ is in the following order: 5 (ΔQ = −0.107) > 6a (ΔQ = −0.069) at the B3LYP/6-311+G(2df,2p) level. Part of the reason that the chemical hydrogen-generating system CAMGCC was prepared by using Laves-phase CaMg2 as a superior antioxidant to MGCC prepared by using crystal Mg is related to its chemical hardness (Fig. 8).
Table 3 Calculated quantity of electron transfer (ΔQ) from CaMg2 and Mg to H2O by redox reaction
System |
Method |
Metal |
Medium |
Quantity of electron transfer (ΔQ) |
CAMGCC |
|
B3LYP/6-311+G(2df,2p) |
CaMg2 (3) |
H2O |
−0.11 |
|
M06/6-311+G(2df,2p) |
3
|
H2O |
−0.11 |
|
|
B3LYP/6-311+G(2df,2p) |
CaMg2 cluster (5) |
H2O |
−0.107 |
|
M06/6-311+G(2df,2p) |
5
|
H2O |
−0.107 |
|
MGCC |
|
B3LYP/6-311+G(2df,2p) |
Mg (4) |
H2O |
−0.097 |
|
M06/6-311+G(2df,2p) |
4
|
H2O |
−0.096 |
|
|
B3LYP/6-311+G(2df,2p) |
Mg3 cluster (6a) |
H2O |
−0.069 |
|
M06/6-311+G(2df,2p) |
6a
|
H2O |
−0.074 |
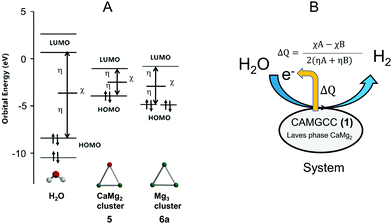 |
| Fig. 8 Orbital energy diagram, HOMO, and LUMO phases for optimized models of CaMg2 and Mg clusters, and hardness (η) and electronegativity (χ) of H2O, CaMg2, and Mg3 clusters (A) and H2 generation by the reaction of CAMGCC with H2O (B). (A) The orbital energy, εhomo, and εlumo for clusters of CaMg2 and Mg3 optimized by using the B3LYP/6-311+G(2df,2p) method. The η and χ are shown in the diagram of the H2O, CaMg2, and Mg3 clusters. The values of 2η and χ are equal to Ip − Ea ≒ εhomo − εlumo and Ip − η ≒ εhomo − η, respectively. (B) Model of H2 generation by the reaction of CAMGCC with H2O on the CAMGCC surface. | |
We report for the first time the chemical properties, reactivity, and antioxidant activity of a new chemical hydrogen-generating system, CAMGCC, prepared using Laves-phase CaMg2. The CAMGCC generated gaseous H2 by the effect of solid citric acid on the CAMGCC's surface in water, and showed the selective scavenging activity of ˙OH generated by the ultraviolet (UV) photolysis of H2O2. CaMg2 is classified as Laves-phase AB2, and is well known for its chemical storage of H2. Zirconium-based and NiMg2 alloys have been developed for the reversible storage of H2.25,26 However, the CAMGCC system reported in this study was not prepared for use as a prospective hydrogen-storage material but rather for use as a hydrogen-generating system that can generate H2 rapidly in water. CAMGCC has different chemical properties for the oxidation-resistance of CaMg2 alloys because the CAMGCC by the effect of the citric acid on the CaMg2 surface is oxidized easily in H2O. The amount of H2 that is generated from the CAMGCC system was 4 ml (0.27 mmol of CaMg2) for 3 min, which is about 3 times the amount of H2 produced from an equimolar amount (0.27 mmol of Mg) of MGCC (eqn (2)). Therefore, the stoichiometry of the reaction of CaMg2 (CAMGCC) with H2O is best expressed by eqn (3).
| Mg (MGCC) + 2H2O → Mg(OH)2 + H2 | (2) |
| CaMg2 (CAMGCC) + 6H2O → Ca(OH)2 + 2Mg(OH)2 + 3H2 | (3) |
Although the gaseous H
2, D
2, and HD that were generated from CAMGCC in H
2O were identified by using a GC–MS technique (
Fig. 2), the bands of D
2 and HD were derived from D
2O, including in water. The equilibrium constant (
K) of H
2O and D
2O is 4, and it is expressed in the following equilibrium, H
2O + D
2O ⇄ 2HDO (
K = 4).
27 Therefore, HD observed by GC–MS measurements was produced by the reaction of CAMGCC with HDO.
The CAMGCC system proved a useful antioxidant to scavenge reactive oxygen species, such as O2−˙ and ˙OH. The scavenging of toxic ˙OH translates biologically into a reduction of the oxidative stress of cells, living tissues, and body systems. We explored the antioxidant activity of CAMGCC by the reaction of CAMGCC with ˙OH generated from the photolysis of H2O2 as a biological model of ˙OH. Hydrogen gas was generated vigorously with fine bubbles in the five-surface transparent quartz cell to which CAMGCC was added. Fine H2 bubbles that were generated from the CAMGCC surface scavenged the ˙OH generated by the UV photolysis of H2O2. The CAMGCC system prepared with Laves-phase CaMg2 has a stronger ˙OH scavenging activity than the MGCC prepared with Mg as shown in Fig. 4. The ˙OH scavenging activity (IC50) of CAMGCC was 1.68 ± 0.105 (mg/400 μL), and the IC50 converted to moles is equal to 5.96 mM. The IC50 is for a molecular weight of CaMg2 (M.W. = 88). The IC50 of Trolox is usually used as a standard for scavenging activity. The scavenging activity of CAMGCC was 1/50 times that of Trolox, as shown in Fig. 4. Although CAMGCC is not as strong an antioxidant as Trolox and other polyphenols, it is thought that the new system, CAMGCC, is an antioxidant that scavenges highly toxic ˙OH selectively.
The CAMGCC and MGCC were prepared using Laves-phase CaMg2 and Mg, respectively, and the lattice structures of CaMg2 and Mg were classified as hexagonal P63/mmc (No. 194) (Fig. 6). Clusters 5 and 6 are considered to be cluster approximations of 3 and 4 because the η and χ of clusters 5 and 6 have a trend that is similar to that of crystals CaMg23 and Mg 4. The calculated 0.216 eV for the binding energy of Ca–Mg of 5 was smaller than the 46.4 kcal mol−1 of cluster Cu–Cu.28 That is, CaMg2 is known to be a brittle alloy. We showed that the chemical hardness of clusters CaMg2 and Mg plays a key role in understanding the mechanism of the antioxidant reaction of CAMGCC 1 and MGCC 2. The ΔQ values of electron transfer between CAMGCC (and MGCC) and H2O are an important quantity for understanding why the ˙OH scavenging activity of CAMGCC is stronger than that of MGCC; namely, the ˙OH scavenging activity by CAMGCC results from a redox reaction of CAMGCC with H2O. To develop an effective hydrogen-generating system, we have to consider that Laves-phase AB2 structures possess a small absolute hardness and a large absolute electronegativity. The theoretical results in Table 3 suggest that the ΔQ of electron transfer from Laves-phases AB2 to H2O can be used to develop ˙OH and O2−˙ scavenging active chemical hydrogen-generating systems. Therefore, the absolute hardness and ΔQ values are useful parameters for developing a chemical hydrogen-generating system with a strong reducing power. It can be predicted that the ΔQ values of electron transfer from metals and alloys to water are a determining factor in the development of an excellent chemical hydrogen-generating system as shown in Fig. 8B.
Conclusions
We developed a new chemical hydrogen-generating system CAMGCC using a Laves-phase CaMg2 alloy prepared by arc melting. The CAMGCC generated H2 readily by H2O addition. Our study revealed for the first time that Laves-phase CaMg2 is superior to Mg to prepare a chemical hydrogen-generating system, because the equimolar H2 gas volume quantified by using the gas-over-water technique was higher in CaMg2 than in Mg (Fig. 3). Our results show that the CAMGCC is an excellent antioxidant (Fig. 4D and 5C). The CAMGCC scavenged highly toxic ˙OH and O2−˙ selectively, which are well known to be the cause of many diseases in the living body. Therefore, the development of antioxidant materials that scavenge ROS is important for materials science and from the viewpoint of medical care. We propose that the ΔQ of electron transfer from Laves-phase AB2 to H2O is an important parameter to develop chemical hydrogen-generating systems. The results were supported by the calculated ΔQ of electron transfer by the reaction of the crystal structures of the Laves-phase CaMg2 and Mg calculated by a DFT method, B3LYP/6-311+G(2df,2p) and M06/6-311+G(2df,2p), with H2O. As a clarification of the results, our datashow that CaMg2 (−0.107, Table 3) has a larger ΔQ of charge transfer to H2O than Mg3 (−0.069, Table 3) as demonstrated by the cluster model. Applications of a material CAMGCC system containing Ca to the prevention of disease by oxidative stress can be expected in the future.
Materials and methods
Materials
Magnesium metal powder, calcium metal shot, and citric acid (anhydride) were obtained from Wako Pure Chemical Industries, Ltd (Osaka, Japan). Mg3(citric acid)2 was obtained from Tokyo Chemical Industry Co. Ltd (Tokyo, Japan). Hydroxyphenyl fluorescein (HPF) was obtained from Sekisui Medical Co. Ltd (Tokyo, Japan). The MGCC system was obtained from Nikko-Kasei Co. Ltd (Tokyo, Japan). Standard hydrogen (H2, 99.99% purity) was obtained from GL Sciences Inc. (Tokyo, Japan). Xanthinoxidase (10 U) and hypoxanthine were obtained from Calbiochem (Darmstadt, Germany). All experimental solutions were prepared with degassed distilled water (DW). All other chemicals used were of the highest grade available.
Procedure of the CaMg2 Laves phase and chemical hydrogen-generating (CAMGCC) system
3.4468 g (0.0860 mol) of sheet Ca and 4.0104 g (0.1717 mol) of metal Mg were arc melted together and the Ca–Mg alloy was homogenized under high-purity argon (Ar) gas after evacuation to 3 Pa in a compact arc melting furnace (Diavac Ltd, Chiba, Japan). Air in the glove box was exchanged in Ar flow (7.0 L min−1 for 60 min, 500 L). The box was turned nine times to homogenize the Ca–Mg alloy during arc melting. After arc melting, the Ca–Mg alloy was converted to a fine powder by crushing with a pestle in a porcelain mortar (MP-90, Niigata Seiki Co. Ltd, Sanjo, Japan).
Powder X-ray diffraction method
Powder X-ray diffraction (XRD) measurements were taken on an Ultima IV diffractometer (Rigaku, Tokyo, Japan) using CuKα radiation. The X-ray tube voltage and current were 40 kV and 40 mA, respectively. Data were collected at 2θ from 3° to 120° angle ranges. The detection interval was at a 2θ step of 0.02°.
Chemical hydrogen-generating (CAMGCC) system procedure
The CaMg2-hydroxypropyl cellulose (HPC)–citric acid (CAMGCC) system was prepared by the physical mixing of 1.0 g of CaMg2–HPC (prepared by the physical mixing of 1.0 g of Laves-phase CaMg2 with 0.072 g of HPC) with 7.2 g of citric acid (anhydrous).
Gas chromatography–mass spectrometry
The mass spectra of H2, HD, and D2 gases were collected using a model 6890N network gas chromatography system (Agilent Technologies, Japan) connected to a Model JMS-700 mass spectrometer (JEOL Ltd, Tokyo, Japan).10 The insoluble gases H2, D2, and HD produced from the CAMGCC system in H2O, respectively, were collected and identified by using gas-over-water and injected directly into a helium carrier gas by using a gas-tight instrument syringe (1 mL, Model 1001 RN SYR, Hamilton Co.). The peaks were measured by a rate of temperature increase from 30 to 120 °C. The hydrogen gas peak by GC–MS was identified by using standard hydrogen. RT®-Msieve 5A columns (30 m × 0.32 mm, coated with 30-μm thick molecular sieves) (RESTEK Co., Bellefonte PA, USA) were used for the helium carrier gas. Gas chromatography was used to introduce the generated gases into the mass spectrometer. The injector and detector temperatures were maintained at 30 °C. EI mass spectra were acquired over a mass range of m/z 0 to 40. The ratio of generated D2, H2, and HD ions (m/z) was obtained from the height or area value of each peak of the GC spectrum using the SIM mode.
Crystal structure and chemical hardness of Laves-phase CaMg2
The crystal structures of Laves-phase CaMg2 and Mg comprise a hexagonal close-packed (hcp) system with a space group P63/mmc (No. 194). The experimental parameters of Laves-phase CaMg2 (3)21,22 and Mg (4)23 are a = b = 6.220 Å, c = 10.100 Å, α = β = 90°, and γ = 120° and a = b = 3.209 Å, c = 5.2105 Å (see Fig. 6), respectively. The crystal structures of CaMg2 and Mg were visualized using VESTA (three-dimensional visualization system of crystal structures) and modeled using Spartan’16 (Wavefunction, Inc., Irvine, CA, U.S.A.). The electron energy, the HOMO and LUMO (frontier orbitals) energy levels, and the orbital phases of crystals CaMg23 and Mg 4 were single-point computed using B3LYP and M06 methods by DFT with 6-311+G(2df,2p) and 6-311+G(d,p) as the basis set. To understand the electronic states of crystal CaMg2 and Mg, optimized structures of clusters of CaMg2 (5) and Mg3 (6) were computed using B3LYP and M06 methods with 6-311+G(2df,2p) as the basis set. The calculated values were collected using Spartan’16. The binding energies of Ca–Mg and Mg–Mg in the CaMg2 and Mg3 clusters were corrected for BSSE by using the counterpoise (CP) correction. For each calculation, the BSSE-corrected value was obtained using the keyword “INTERACTIONENERGY = BSSE”.
The absolute hardness (η) and absolute electronegativity (χ) were calculated from eqn (4) and (5) as defined by Parr and Pearson:29,30
| χ = −μ = −(∂E/∂N)v(r) = (Ip + Ea)/2 = −(εLUMO + εHOMO)/2 | (4) |
| η = (∂μ/∂N)v(r)/2 = (∂2E/∂N2)/2 = (εLUMO − εHOMO)/2 | (5) |
where
E is the electronic energy of a molecule,
N is the number of electrons,
v(
r) is the external electrostatic potential, and
Ip and
Ea are the ionization energy and the electron affinity (eV). According to Koopmans’ theorem, the
εHOMO and
εLUMO, which are the energy levels for the frontier orbitals, are roughly equal to
Ip and
Ea, respectively.
The quantity of electron transfer (ΔQ) has been given by eqn (6):29,31
| ΔQ = (χA − χB)/2(ηA + ηB) | (6) |
If ΔQ is positive, the electrons are transferred from A to B. If ΔQ is negative, the electrons are transferred from B to A. The reaction between CAMGCC (and MGCC) (A) and H2O (B) is a redox reaction because the CAMGCC (and MGCC) system is oxidized by H2O.
Generation of hydroxyl radicals (OH˙) by UV photolysis
Hydroxy radicals (˙OH) were generated by ultraviolet (UV) photolysis. The generated ˙OH radicals were detected using hydroxyphenyl fluorescein (HPF)32 as a fluorescent probe, which becomes strongly fluorescent by reaction with ˙OH. HPF (100 μL, 5 μM) in 10 mM phosphate solution (pH 7.4) was added to a five-surface transparent quartz cell (10 mm × 10 mm × 45 mm) that contained 300 μL of 3% H2O2 in 10 mM phosphate (pH 7.4). The solution was irradiated by UV for 60 s using the UV transilluminator (λ = 315 nm, TP-20ME, ATTO Co., Japan) in the dark. After UV irradiation, a total volume of 3 mL solution was prepared to measure its relative fluorescence intensity (F) by adding 10 mM phosphate buffer (pH 7.4) to the quartz cell and by collecting the F values by spectrofluorophotometer (JASCO FP-6200, JASCO Co., Tokyo, Japan). Wavelengths of 490 and 515 nm were used as excitation and emission wavelengths, respectively.
Scavenging of hydroxyl radicals (˙OH) by the CAMGCC system
The ˙OH scavenging reaction was performed by adding 0, 1, 2, 3, 4, 5, 8, 11, and 15 mg each of CAMGCC to 400 μL of the quartz cell solution described above. After mixing by mild pipetting two times and UV irradiation for 60 s, 10 mM phosphate buffer (pH 7.4) was added to prepare a final volume of 3 mL of the UV irradiated solution mix. Wavelengths of 490 and 515 nm were used as excitation and emission wavelengths, respectively.
Scavenging of superoxide anion radicals (O2−˙) by the CAMGCC system
Superoxide anion radicals (O2−˙) were generated by using the xanthine oxidase-hypoxanthine (XOD-HPX) system and O2−˙ was emitted to reduce 2-methyl-6-p-methoxyphenylethynyl-imidazopyrazinone (MPEC) (ATTO Co., Tokyo, Japan), which yielded a chemiluminescent product. The chemiluminescence intensity (FCL) was measured with a lumicounter (Lumat LB9507, Berthold). The XOD (0.1 U mL−1) and HPX (1.5 mM prepared from 7.5 mM HPX) were prepared with 0.1 M phosphate buffer (pH 7.5). The O2−˙ scavenging reaction was performed in 300 μL at 25 °C by mixing XOD (60 μL), 0.1 M phosphate buffer (160 μL), and MPEC (10 μL in DW). Test compounds were mixed in test tubes (20 μL) immediately before HPX addition (50 μL), and the final concentrations of the test systems, CAMGCC (1) and MGCC (2), were 0, 1, 2, 4, 6, 9, 11, and 14 mg. The reaction mixtures were incubated at 37 °C in a water bath for 10 s. The reaction solution without the test systems was equilibrated to the desired level of FCL output for 1 min. The half-maximal inhibitory concentration (IC50) was calculated from the dose–FCL curve obtained from measured FCL, and the concentrations of the test systems were as shown in Fig. 5A–C.
Conflicts of interest
There are no conflicts to declare.
Acknowledgements
The authors would like to thank Dr Masahiro Uda for helpful discussions and for the arc melting techniques.
References
- A. F. Dale brook, W. Gan, M. Grasermann, S. Moret and G. Laurenczy, Chem. Commun., 2013, 49, 8735–8751 RSC.
- Y. H. Hu, Int. J. Energy Res., 2013, 37, 683–685 CrossRef CAS.
- I. Ohsawa, M. Ishikawa, K. Takahashi, M. Watanabe, K. Nishimaki, K. Yamagata, K. Katsura, Y. Katayama, S. Asoh and S. Ohta, Nat. Med., 2007, 13, 688–694 CrossRef CAS PubMed.
- K. Ohno, M. Ito, M. Ichihara and M. Ito, Oxid. Med. Cell. Longevity, 2012, 11 Search PubMed.
- S. Ohta, Pharmacol. Ther., 2014, 144, 1–11 CrossRef CAS PubMed.
- J. F. Fan, C. L. Yang, G. Han, S. Fang, W. D. Yang and B. S. Xu, J. Alloys Compd., 2011, 509, 2137–2142 CrossRef CAS.
- J. F. Stampfer, C. E. Holley Jr and J. F. Scttle, J. Am. Chem. Soc., 1960, 82, 3504–3508 CrossRef CAS.
- N. Terashita and E. Akiba, Mater. Trans., 2004, 45, 2594–2597 CrossRef CAS.
- T. Nobuki, M. Chiba and T. Kuji, J. Alloys Compd., 2007, 446, 152–156 CrossRef.
- S. Kobayashi, T. Chikuma, K. Chiba, D. Tsuchiya and T. Hirai, J. Mol. Struct., 2016, 1106, 468–478 CrossRef CAS.
- O. Shimomura, C. Wu, A. Murai and H. Nakamura, Anal. Biochem., 1998, 258, 230–235 CrossRef CAS PubMed.
- P. Attri, Y. H. Kim, D. H. Park, J. H. Park, Y. J. Hong, H. S. Uhm and K.-N. Kim, Nat. Sci. Rep., 2015, 5, 9332–9339 CrossRef CAS PubMed.
- O. Legrini, E. Oliveros and A. M. Braun, Chem. Rev., 1993, 93, 671–698 CrossRef CAS.
- S. Gligorovski, R. Strekowski, S. Barbati and D. Vione, Chem. Rev., 2015, 115, 13051–13092 CrossRef CAS PubMed.
- J. Jellinek and P. H. Acioli, J. Phys. Chem. A, 2002, 106(45), 10919–10925 CrossRef CAS.
- C. Walling, Acc. Chem. Res., 1975, 8, 125–131 CrossRef CAS.
- L. M. Kohan, S. Sanguanmith, J. Meesungnoen, P. Causey, C. R. Stuart and J.-P. Jay-Gerin, RSC Adv., 2013, 3, 19282–19299 RSC.
- H. Herrmann, Phys. Chem. Chem. Phys., 2007, 9, 3935–3964 RSC.
- R. Re, N. Pellegrini, A. Proteggente, A. Pannala, M. Yang and C. Rice-Evans, Free Radical Biol. Med., 1999, 26, 1231–1237 CrossRef CAS PubMed.
- S. Kobayashi and S. Kanai, Molecules, 2013, 18, 6128–6141 CrossRef CAS PubMed.
- K. Aono, S. Orimo and H. Fujii, J. Alloys Compd., 2000, 309, L1–L4 CrossRef CAS.
- J. Fu, W. Lin and Z. Chen, Int. J. Advanced Materials and Production, 2016, 1, 62–69 Search PubMed.
-
M. D. Graef and M. McHerry, Crystal Structure Descriptions, 2nd edn, 2013, http://som.web.cmu.edu/frames2.html. Search PubMed.
- S. F. Boys and F. Bernardi, Mol. Phys., 1970, 19, 553–566 CrossRef CAS.
- D. G. Ivey and D. O. Northwood, Z. Phys. Chem., 1986, 147, 191–209 CrossRef CAS.
- S. B. Gesari, M. E. Pronsato, A. Visintin and A. Juan, J. Phys. Chem. C, 2010, 114, 16832–16836 CrossRef CAS.
- L. Friedman and V. J. Shiner Jr., J. Chem. Phys., 1966, 44, 4639–4640 CrossRef CAS.
- M. D. Morse, Chem. Rev., 1986, 86, 1049–1109 CrossRef CAS.
- R. G. Parr and R. G. Pearson, J. Am. Chem. Soc., 1983, 105, 7512–7516 CrossRef CAS.
- R. G. Pearson, J. Chem. Sci., 2005, 117(5), 369–377 CrossRef CAS.
- W. Kohn, A. D. Becke and R. G. Parr, J. Phys. Chem., 1996, 100, 12974–12980 CrossRef CAS.
- K. Setukinai, Y. Urano, K. Kakinuma, H. J. Majima and T. Nagano, J. Bio. Chem., 2003, 278, 3170–3175 Search PubMed.
|
This journal is © the Partner Organisations 2019 |
Click here to see how this site uses Cookies. View our privacy policy here.