DOI:
10.1039/C7RA09100A
(Paper)
RSC Adv., 2017,
7, 52694-52701
Value of mir-247 in warning of graphene oxide toxicity in nematode Caenorhabditis elegans†
Received
17th August 2017
, Accepted 3rd November 2017
First published on 14th November 2017
Abstract
Graphene oxide (GO) induces some dysregulated microRNAs (miRNAs), such as mir-247, in Caenorhabditis elegans. We here further investigated the role and value of mir-247 in detecting GO toxicity in nematodes after prolonged exposure. In nematodes, mir-247 acted in neurons to regulate GO toxicity, and neuronal overexpression of mir-247 induced a susceptibility to GO toxicity. We detected the significant increase in mir-247 in wild-type nematodes exposed to GO at concentrations of more than 10 μg L−1. Moreover, we found the GO toxicity in a transgenic strain overexpressing neuronal mir-247 after exposure to GO at concentrations of more than 10 μg L−1. Therefore, our results imply the important potential of mir-247 in warning of the formation of GO toxicity in the range of μg L−1 in nematodes.
Introduction
Graphenic nanomaterials, such as graphene oxide (GO), are one of the most fascinating nanostructures with unique physical and chemical properties, and are potentially used in biosensors, bio-imaging, cancer therapy, and drug delivery.1–3 Meanwhile, both in vitro and in vivo studies have suggested the potential pulmonary toxicity, reproductive toxicity, and toxic effect on immune response of GO in human cell lines or mammals.4–7Caenorhabditis elegans, a classic model animal, can offer an in vivo assay system for elucidating the toxicological mechanisms of toxicants, including engineered nanomaterials.8 In nematodes, it was reported that GO exposure could cause toxic effects on the functions of both primary (such as intestine) and secondary (such as neuron and reproductive organs) targeted organs.9–12
For the cellular mechanism of GO toxicity in nematodes, it has been shown that activation of oxidative stress, bioavailability, intestinal permeability, defecation behavior, and innate immune response may contribute greatly to the formation of GO toxicity.9,10,13 For the underlying molecular mechanisms of GO toxicity in nematodes, insulin, p38 mitogen-activated protein kinase (MAPK), cell death and DNA damage, and oxidative stress or response associated signaling pathways have been shown to be involved in the control of GO toxicity.12,14–16 Moreover, the potential toxic effects from GO exposure can be inhibited by the treatment with glycyrrhizic acid, an active component from Glycyrrhizae radix, or lactic acid bacteria.11,17
The short noncoding microRNAs (miRNAs) have been shown to exist in many organisms, including C. elegans.18 miRNAs usually act to suppress the expression of certain targeted genes post-transcriptionally.19 So far, the dysregulated miRNAs have already been identified in GO exposed nematodes, and the potential function of some dysregulated miRNAs have been further confirmed using the corresponding mutants.20 Among these candidate miRNAs, our previous study has suggested that GO exposure could increase the expression of mir-247.20 Meanwhile, the mir-247/797 mutant showed the resistant property to GO toxicity on lifespan and aging related phenotypes,20 implying that the activated mir-247 may mediate the molecular mechanism for the GO toxicity formation. However, the environmental implication of mir-247 in the safety assessment of GO exposure is still unclear. Thus, we here further employed in vivo assay system of C. elegans to investigate the potential value of mir-247 expression in the safety assessment of environmental GO exposure. In C. elegans, mir-247 is expressed in several tissues, including pharynx and neurons.21,22 We also examined the tissue-specific activity of mir-247 in affecting the induction of GO toxicity. Our results suggest the potential value of alteration in mir-247 expression in the assessment or prediction of toxicity induced by environmental exposure to GO.
Experimental
Preparation and characterization of GO
GO was prepared from natural graphite powder using the modified Hummer's method.23 Graphite (2 g) and sodium nitrate (1 g) were added into a 250 mL flask. Concentrated H2SO4 (50 mL) was added on ice, and KMnO4 (7 g) was further added. After the mixture temperature reached to 35 °C, H2O (90 mL) was slowly dripped into the paste to dilute it. After stirring the diluted suspension at 70 °C for 15 min, the suspension was treated with a mixture of 7 mL of 30% H2O2 and 55 mL of H2O. The resulting warm suspension was filtered to obtain a yellow-brown filter cake, which was washed with 3% HCl, followed by drying at 40 °C for 24 h. GO would be obtained after ultrasonication of as-made graphite oxide in water for 1 h.
GO was characterized by atomic force microscopy (AFM, SPM-9600, Shimadzu, Japan), Raman spectroscopy using a 632 nm wavelength excitation (Renishaw Invia Plus laser Raman spectrometer, Renishaw, UK), and zeta potential determined by dynamic light scattering (DLS) using a Nano Zetasizer (Malvern Instrument Ltd., UK). Based on the AFM assay, the thickness of GO was approximately 1.0 nm in topographic height, corresponding to the property of one layer (Fig. 1a). Sizes of GO in K-medium after sonication (40 kHz, 100 W, 30 min) were mainly in the range of 40–50 nm (Fig. 1b). Raman spectroscopy assay indicated the existence of G band at 1592 cm−1 and D band at 1326 cm−1 (Fig. 1c). The zeta potential of GO (10 mg L−1) in K-medium was −22.3 ± 2.7 mV.
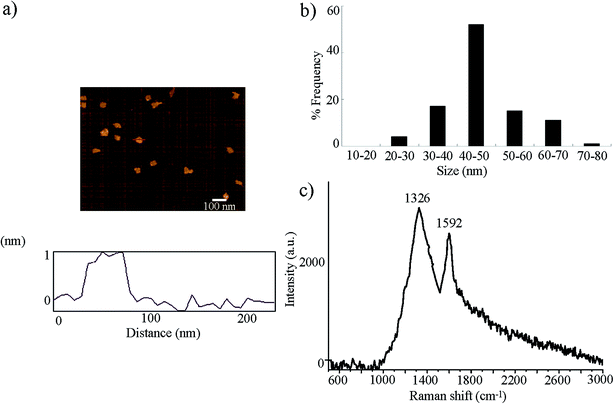 |
| Fig. 1 Physiochemical properties of GO. (a) AFM analysis of GO after sonication. (b) Size distribution of GO after sonication. (c) Raman spectrum of GO. | |
C. elegans strains and exposure
Nematodes used were wild-type N2, mutant of mir-247/797(n4505), and transgenic strains of mir-247/797(n4505)Ex(Pmir-247-mir-247), mir-247/797(n4505)Ex(Pmir-797-mir-797), mir-247/797(n4505)Ex(Punc-14-mir-247), mir-247/797(n4505)Ex(Pmyo-2-mir-247), and Ex(Punc-14-mir-247). Some of the used strains were from Caenorhabditis Genetics Center (funded by NIH Office of Research Infrastructure Programs (P40 OD010440)). Nematodes were maintained on nematode growth medium (NGM) plates seeded with Escherichia coli OP50 at 20 °C as described.24 The nematodes were lysed with a bleaching mixture (0.45 mol L−1 NaOH, 2% HOCl) after washing off the plates into centrifuge tubes, and age synchronous L1-larvae populations were prepared as described.25
Exposure and toxicity assessment
After sonication for 30 min (40 kHz, 100 W), GO was dispersed in K medium to prepare the stock solution (1 mg mL−1). GO at working concentrations were prepared by diluting the stock solution with K medium. Prolonged exposure to GO was performed from L1-larvae to adult day-1 (approximately 4.5 days) in liquid K medium in 12-well sterile tissue culture plates at 20 °C in the presence of food (OP50). After prolonged exposure to GO, the examined nematodes were used for toxicity assessment using lifespan and intestinal reactive oxygen species (ROS) production as the endpoints.
Lifespan assay was preformed at 20 °C basically as described.26,27 During the lifespan assay, hermaphrodite nematodes were transferred daily for the first 7 days of adulthood. Nematodes were checked every day, and scored as dead if they did not move even after repeated taps with a pick. Sixty nematodes were examined per treatment, and three replicates were performed.
Intestinal ROS production assay was performed as described previously.28,29 The endpoint of intestinal ROS production was used to reflect the induction of oxidative stress. After prolonged exposure to GO, the examined nematodes were transferred to 1 μmol L−1 5′,6′-chloromethyl-2′,7′-dichlorodihydro-fluorescein diacetate (CM-H2DCFDA; Molecular Probes) to incubate for 3 h in the dark. The nematodes were examined under a laser scanning confocal microscope (Leica, TCS SP2, Bensheim, Germany) at 488 nm of excitation wavelength and at 510 nm of emission filter. Relative fluorescence intensity of ROS signals in intestine was semi-quantified, and expressed as relative fluorescence units (RFU). Sixty nematodes were examined per treatment.
Reverse-transcription and quantitative real-time polymerase chain reaction (PCR)
Total RNA was isolated from the nematodes using Trizol reagent (Invitrogen, UK) according to manufacturer's protocol. The extracted RNA was used for the further cDNA synthesis. Relative expression levels of the mir-247 were measured by real-time PCR (RT-PCR) in an ABI 7500 with Evagreen (Biotium, USA). Three biological replicates were performed. Expression of mir-247 or mir-797 is presented as relative expression ratio between mir-247 or mir-797 and F35C11.9, which encodes a small nuclear RNA U6. The related primer information is shown in Table S1.†
DNA constructs and germline transformation
To generate entry vector carrying promoter sequence, promoter region for unc-14 gene specially expressed in neurons, myo-2 gene specially expressed in pharynx, mir-247, or mir-797 was amplified by PCR from wild-type C. elegans genomic DNA. These promoter fragments were inserted into pPD95_77 vector in the sense orientation. mir-247 or mir-797 was amplified by PCR, and inserted into corresponding entry vector carrying the unc-14, myo-2, mir-247, or mir-797 promoter sequence. Transformation was performed by coinjection testing DNA at the concentration of 10–40 μg mL−1 and marker DNA of Pdop-1::rfp at the concentration of 60 μg mL−1 into the gonad of nematodes as described.30 The related primer information for DNA constructs is shown in Table S2.†
Statistical analysis
Data in this article were expressed as means ± standard deviation (SD). Statistical analysis was performed using SPSS 12.0 software (SPSS Inc., Chicago, USA). Differences between groups were determined using analysis of variance (ANOVA), and probability levels of 0.05 and 0.01 were considered statistically significant. The lifespan data were analyzed using a 2-tailed 2 sample t-test (Minitab Ltd, Coventry, UK).
Results
Confirmation of the contribution of mir-247 in the regulation of GO toxicity
mir-247/797(n4505) mutant contains both the mutation of mir-247 and the mutation of mir-797, and was resistant to GO toxicity in reducing lifespan and in inducing ROS production (Fig. 2).20 To determine whether the resistance of mir-247/797(n4505) mutant to GO toxicity is due to the functional deficit in mir-247, mir-247 or mir-797 was expressed in mir-247/797(n4505) mutant. The expression of mir-247 or mir-797 in mir-247/797(n4505) mutant was confirmed by qRT-PCR (Fig. S1†). It was reported that prolonged exposure (from L1-larvae to young adults) to GO at the concentrations more than 1000 μg L−1 could decrease the locomotion behavior and induce the significant induction of intestinal ROS production in nematodes.9 We selected 10
000 μg L−1 as the working concentration for GO to confirm the contribution of mir-247 in the regulation of GO toxicity. After GO exposure, expression of mir-797 did not significantly affect the lifespan and the induction of ROS production in mir-247/797(n4505) mutant (Fig. 2). In contrast, after GO exposure, expression of mir-247 significantly suppressed the resistance of mir-247/797(n4505) mutant to GO toxicity in reducing lifespan and in inducing ROS production, and the phenotypes of lifespan and induction of ROS production in GO exposed mir-247/797(n4505)Ex(Pmir-247-mir-247) were similar to those in GO exposed wild-type nematodes (Fig. 2). Therefore, the formation of resistance in mir-247/797(n4505) mutant to GO toxicity may be due to the mutation of mir-247.
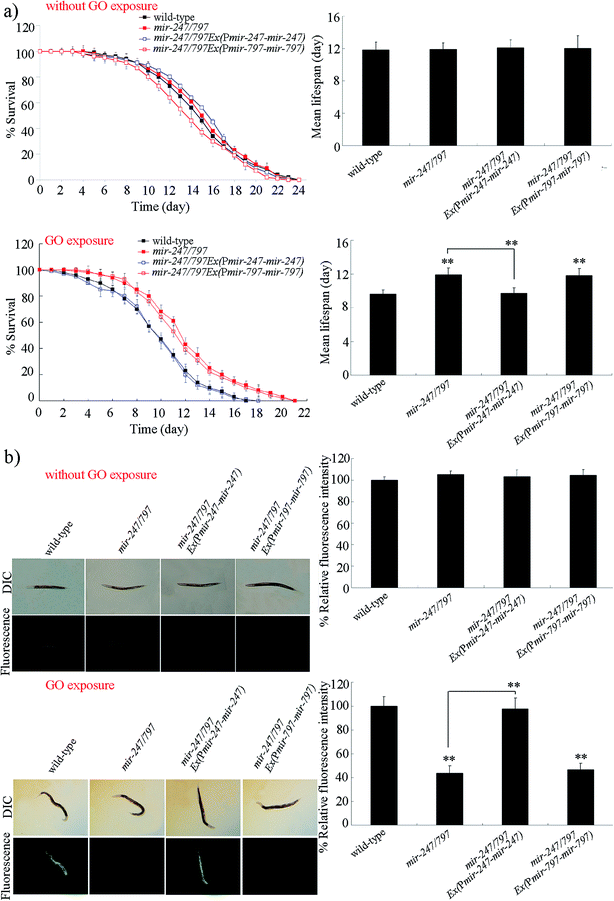 |
| Fig. 2 Rescue assay of GO toxicity in mir-247/797 mutant nematodes. (a) Rescue assay of GO toxicity on lifespan in mir-247/797 mutant nematodes. (b) Rescue assay of GO toxicity in inducing intestinal ROS production in mir-247/797 mutant nematodes. GO exposure concentration was 10 000 μg L−1. Prolonged exposure was performed from L1-larvae to adult day-1. Bars represent means ± SD. **P < 0.01 vs. wild-type (if not specially indicated). | |
Tissue-specific activity of mir-247 in the regulation of GO toxicity
Using tissue-specific promoters, we further examined the tissue-specific activity of mir-247 in the regulation of GO toxicity. Expression of mir-247 in neurons or pharynx in mir-247/797(n4505) mutant was confirmed by qRT-PCR (Fig. S2†). Rescue assay by expression of mir-247 in the pharynx did not significantly affect the resistance of mir-247/797(n4505) mutant to GO (10
000 μg L−1) toxicity in reducing lifespan and in inducing ROS production (Fig. 3). In contrast, expression of mir-247 in the neurons significantly inhibited the resistance of mir-247/797(n4505) mutant to GO (10
000 μg L−1) toxicity in reducing lifespan and in inducing ROS production (Fig. 3). Therefore, mir-247 may act in the neurons to regulate the GO toxicity.
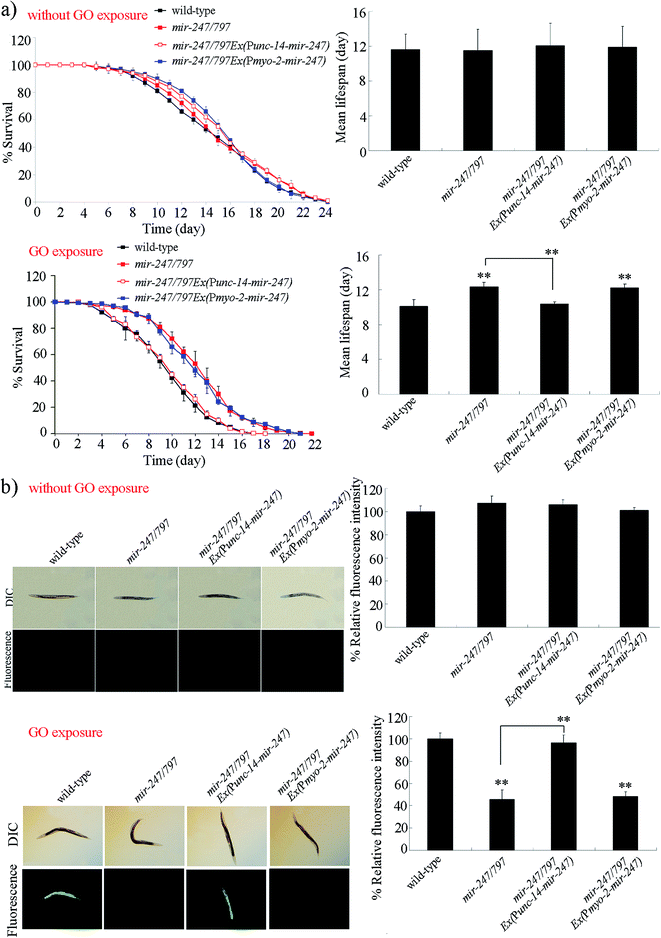 |
| Fig. 3 Tissue-specific activity of mir-247 in the regulation of GO toxicity. (a) Tissue-specific activity of mir-247 in the regulation of GO toxicity on lifespan. (b) Tissue-specific activity of mir-247 in the regulation of GO toxicity in inducing intestinal ROS production. GO exposure concentration was 10 000 μg L−1. Prolonged exposure was performed from L1-larvae to adult day-1. Bars represent means ± SD. **P < 0.01 vs. wild-type (if not specially indicated). | |
Neuronal overexpression of mir-247 enhanced the GO toxicity
To further investigate the function of neuronal mir-247 in the regulation of GO toxicity, we generated the transgenic strain overexpressing neuronal mir-247. Overexpression of mir-247 in Ex(Punc-14-mir-247) was confirmed by qRT-PCR (Fig. S3†). Under normal conditions, the transgenic strain of Ex(Punc-14-mir-247) exhibits the similar lifespan to wild-type nematodes, and do not show the obvious induction of ROS production (Fig. 4). However, after GO (10
000 μg L−1) exposure, the transgenic strain of Ex(Punc-14-mir-247) showed the more decreased lifespan and the more severe induction of ROS production than those in wild-type nematodes (Fig. 4). Therefore, overexpression of neuronal mir-247 may induce the enhancement of GO toxicity in nematodes.
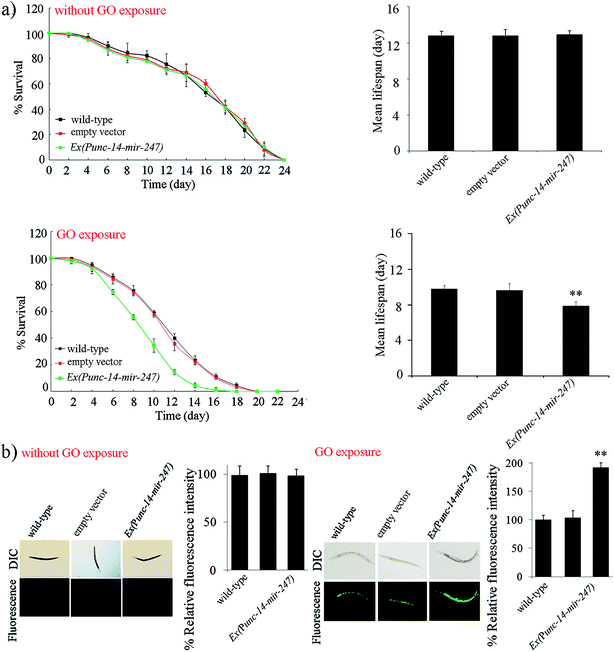 |
| Fig. 4 Effect of neuronal overexpression of mir-247 on GO toxicity. (a) Effect of neuronal overexpression of mir-247 on GO toxicity in reducing lifespan. (b) Effect of neuronal overexpression of mir-247 on GO toxicity in inducing intestinal ROS production. GO exposure concentration was 10 000 μg L−1. Prolonged exposure was performed from L1-larvae to adult day-1. Bars represent means ± SD. **P < 0.01 vs. wild-type. | |
Toxicity assessment of GO in nematodes overexpressing neuronal mir-247
We next performed the toxicity assessment of GO at different concentrations in nematodes overexpressing neuronal mir-247 using intestinal ROS production as the endpoint. After prolonged exposure, we observed that GO at concentrations more than 100 μg L−1 caused the significant induction of intestinal ROS production in wild-type nematodes (Fig. 5). In contrast, we detected the significant induction of intestinal ROS production in nematodes overexpressing neuronal mir-247 exposed to GO at concentrations more than 10 μg L−1 (Fig. 5). Moreover, the induction of intestinal ROS production was more severe in nematodes overexpressing neuronal mir-247 than that in wild-type nematodes after exposure to GO at the concentration of 100 or 1000 μg L−1 (Fig. 5).
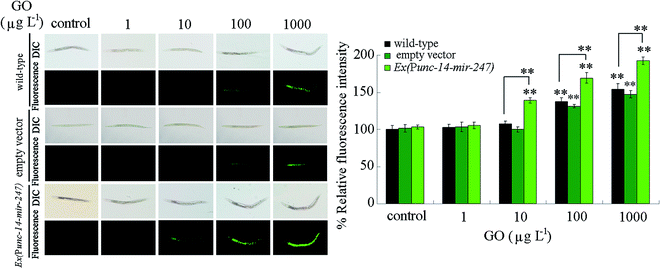 |
| Fig. 5 Toxicity assessment of GO in nematodes overexpressing neuronal mir-247 using intestinal ROS production as the endpoint. Prolonged exposure was performed from L1-larvae to adult day-1. Bars represent means ± SD. **P < 0.01 vs. control (if not specially indicated). | |
Alteration in mir-247 expression in GO exposed wild-type nematodes
We further investigated the expressional alteration of mir-247 in GO exposed wild-type nematodes. After prolonged exposure to 1 μg L−1 of GO, we did not detect the obvious alteration in mir-247 expression (Fig. 6). However, we observed the significant increase in mir-247 expression in wild-type exposed to GO at concentrations more than 10 μg L−1 (Fig. 6).
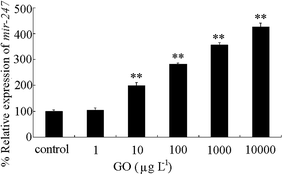 |
| Fig. 6 Expression of mir-247 in wild-type nematodes after prolonged exposure. Prolonged exposure was performed from L1-larvae to adult day-1. Bars represent means ± SD. **P < 0.01 vs. control. | |
Discussion
It has been shown that mir-247 is involved in the control of male tail development, and overexpression of mir-247 induces the abnormalities in mail tail rays.22 In this study, we provide the direct evidence to indicate the involvement of mir-247 in the control of GO toxicity. The rescue assays demonstrated that that the observed resistance of mir-247/797(n4505) mutant to GO toxicity in reducing lifespan and in inducing ROS production was due to the mutation of mir-247 (Fig. 2). In addition, GO exposure could increase the transcriptional expression of mir-247 in nematodes.20 These results suggest that mir-247 may positively regulate the toxicity formation of certain environmental toxicants, such as GO. Under normal conditions, mutation or overexpression of mir-247 did not induce the significant intestinal ROS production (Fig. 2b and 4b). Therefore, although mir-247 may not regulate the oxidative stress under normal conditions, overexpression of mir-247 may induce a susceptibility to oxidative stress or stress response in nematodes. In nematodes, PEG surface modification or fetal bovine serum (FBS) coating could reduce GO toxicity.31 Meanwhile, we observed that PEG surface modification or FBS coating could further suppress the mir-247 expression in GO exposed nematodes (Fig. S4†), which further confirms the potential role of mir-247 in the regulation of GO toxicity. Nevertheless, we did not observe the significant alteration in mir-247 expression in reduced graphene oxide (rGO) or multiwalled carbon nanotubes exposed nematodes (data not shown).32
In C. elegans, insulin and p38 mitogen-activated protein kinase (MAPK) signaling pathways have been shown to act in the intestine to regulate the GO toxicity.15,33,34 Moreover, cell apoptosis and DNA damage signaling pathways may function in the germline to regulate the reproductive toxicity of GO.16 In this study, mir-247 may mediate a certain molecular signaling in the neurons to regulate the GO toxicity, since mir-247 expression in the neurons suppressed the resistance of mir-247/797(n4505) mutant to GO toxicity (Fig. 3). Therefore, so far, at least the intestine, the neurons, and the germline, as well as the molecular signals in these organs, may participate in the control of GO toxicity in nematodes. More recently, the ERK signaling was also identified to act in the neurons to regulate the GO toxicity, and the increased expression of neuronal ERK signaling may mediate a protection mechanism for nematodes against the GO toxicity.35 In nematodes, GO is mainly distributed in intestine, pharynx, and reproductive organs, such as gonad.36
In this study, we further provide evidence to indicate that neuronal overexpression of mir-247 induced a susceptibility to GO toxicity (Fig. 4). Using the transgenic strain overexpressing neuronal mir-247 could even detect the toxicity of GO at the concentration of 10 μg L−1 (Fig. 5). It is normally predicted that the environmentally relevant concentrations of engineered nanomaterials (ENMs) are in the range of ng L−1 or μg L−1.37,38 It was reported that certain genetic mutants can be employed to detect the toxicity of ENMs at environmentally relevant concentrations in nematodes.39 Our results suggest that, besides certain genetic mutants, certain transgenic strains could also be helpful for detecting the possible toxicity of ENMs at environmentally relevant concentrations.
In wild-type nematodes, we further found that prolonged exposure to GO at concentrations more than 10 μg L−1 could significantly increase the mir-247 expression (Fig. 6). Meanwhile, we only detected the toxicity in wild-type nematodes exposed to GO at concentrations more than 100 μg L−1 (Fig. 5). These observations imply that the increase in mir-247 may provide a potential warning for the formation of GO toxicity in 10 μg L−1 of GO exposed nematodes.
In nematodes, using TargetScan version 6.2 (http://www.targetscan.org/worm_52/), a large amount of genes may act as the targets of mir-247 (Table S3†). In the list of potential targeted genes for mir-247, we did not find the genes required for the control of oxidative stress. We detected some genes encoding components in important signaling pathways in this list. For example, cwn-1 encodes a Wnt ligand in the Wnt signaling pathway, pkc-1 encodes a serine/threonine protein kinase in the ERK signaling pathway, and goa-1 encodes a heterotrimeric G protein in the G-protein signaling pathway. It has been reported that at least CWN-1 and PKC-1 are involved in the control of GO toxicity in nematodes.29,40
Conclusions
In this study, we determined the role and value of mir-247 in the detection of GO toxicity using in vivo assay system of C. elegans. In nematodes, mir-247 acted in the neurons to regulate GO toxicity. Neuronal overexpression of mir-247 induced a susceptibility to GO toxicity. In wild-type nematodes, we detected the toxicity of GO at concentrations more than 100 μg L−1. In contrast, we could observe the toxicity in nematodes exposed to GO at concentrations more than 10 μg L−1 in transgenic strain overexpressing neuronal mir-247. More importantly, we found the significant increase in mir-247 in nematodes exposed to GO at concentrations more than 10 μg L−1. Our results suggest the important value of increase in mir-247 in warning the potential of GO toxicity in nematodes.
Conflicts of interest
There are no conflicts to declare.
References
- A. K. Geim, Science, 2009, 324, 1530–1534 CrossRef CAS PubMed.
- D. Bitounis, H. Ali-Boucetta, B. H. Hong, D. Min and K. Kostarelos, Adv. Mater., 2013, 25, 2258–2268 CrossRef CAS.
- K. Yang, S. Zhang, G. Zhang, X. Sun, S. Lee and Z. Liu, Nano Lett., 2010, 10, 3318–3323 CrossRef CAS.
- K. Yang, Y. Li, X. Tan, R. Peng and Z. Liu, Small, 2013, 9, 1492–1503 CrossRef CAS.
- G. Qu, S. Zhang, L. Wang, X. Wang, B. Sun, N. Yin, X. Gao, T. Xia, J. Chen and G. Jiang, ACS Nano, 2013, 7, 5732–5745 CrossRef CAS.
- Y. P. Li, Q. L. Wu, Y. L. Zhao, Y. F. Bai, P. S. Chen, T. Xia and D. Y. Wang, ACS Nano, 2014, 8, 2100–2110 CrossRef CAS.
- S. Liang, S. Xu, D. Zhang, J. He and M. Chu, Biomaterials, 2015, 9, 92–105 CAS.
- Y. L. Zhao, Q. L. Wu, Y. P. Li and D. Y. Wang, RSC Adv., 2013, 3, 5741–5757 RSC.
- Q. L. Wu, L. Yin, X. Li, M. Tang, T. Zhang and D. Y. Wang, Nanoscale, 2013, 5, 9934–9943 RSC.
- Q. L. Wu, Y. L. Zhao, J. P. Fang and D. Y. Wang, Nanoscale, 2014, 6, 5894–5906 RSC.
- Y. L. Zhao, X. M. Yu, R. H. Jia, R. L. Yang, Q. Rui and D. Y. Wang, Sci. Rep., 2015, 5, 17233 CrossRef CAS.
- Y. L. Zhao, Q. L. Wu and D. Y. Wang, RSC Adv., 2015, 5, 92394–92405 RSC.
- W. Zhang, C. Wang, Z. Li, Z. Lu, Y. Li, J. Yin, Y. Zhou, X. Gao, Y. Fang, G. Nie and Y. Zhao, Adv. Mater., 2012, 24, 5391–5397 CrossRef CAS.
- Q. L. Wu, Y. L. Zhao, Y. P. Li and D. Y. Wang, Nanoscale, 2014, 6, 11204–11212 RSC.
- Y. L. Zhao, R. L. Yang, Q. Rui and D. Y. Wang, Sci. Rep., 2016, 6, 24024 CrossRef CAS.
- Y. L. Zhao, Q. L. Wu and D. Y. Wang, Biomaterials, 2016, 79, 15–24 CrossRef CAS.
- Y. L. Zhao, R. H. Jia, Y. Qiao and D. Y. Wang, Nanomedicine, 2016, 12, 735–744 CrossRef CAS PubMed.
- V. Ambros, R. C. Lee, A. Lavanway, P. T. Williams and D. Jewell, Curr. Biol., 2003, 13, 807–818 CrossRef CAS.
- D. P. Bartel, Cell, 2004, 116, 281–297 CrossRef CAS.
- Q. L. Wu, Y. L. Zhao, G. Zhao and D. Y. Wang, Nanomedicine, 2014, 10, 1401–1410 CrossRef CAS.
- N. J. Martinez, M. C. Ow, J. S. Reece-Hoyes, M. I. Barrasa, V. R. Ambros and A. J. Walhout, Genome Res., 2008, 18, 2005–2015 CrossRef CAS.
- H. Zhang and S. W. Emmons, Dev. Dyn., 2009, 238, 595–603 CrossRef CAS.
- N. I. Kovtyukhova, P. J. Olivier, B. R. Martin, T. E. Mallouk, S. A. Chizhik, E. V. Buzaneva and A. D. Gorchinskiy, Chem. Mater., 1999, 11, 771–778 CrossRef CAS.
- S. Brenner, Genetics, 1974, 77, 71–94 CAS.
- S. Donkin and P. L. Williams, Environ. Toxicol. Chem., 1995, 14, 2139–2147 CrossRef CAS.
- S. Shakoor, L. M. Sun and D. Y. Wang, Toxicol. Res., 2016, 5, 492–499 RSC.
- J. N. Yang, Y. L. Zhao, Y. W. Wang, H. F. Wang and D. Y. Wang, Toxicol. Res., 2015, 4, 1498–1510 RSC.
- Q. L. Wu, P. D. Liu, Y. X. Li, M. Du, X. J. Xing and D. Y. Wang, J. Environ. Sci., 2012, 24, 733–742 CrossRef CAS.
- H. Chen, H. R. Li and D. Y. Wang, Sci. Rep., 2017, 7, 41655 CrossRef CAS PubMed.
- C. Mello and A. Fire, Methods Cell Biol., 1995, 48, 451–482 CrossRef CAS.
- Q. L. Wu, X. F. Zhou, X. X. Han, Y. Z. Zhuo, S. T. Zhu, Y. L. Zhao and D. Y. Wang, Biomaterials, 2016, 102, 277–291 CrossRef CAS PubMed.
- Y. L. Zhao, Q. L. Wu, Y.-P. Li, A. Nouara, R. H. Jia and D. Y. Wang, Nanoscale, 2014, 6, 4275–4284 RSC.
- Y. L. Zhao, L. T. Zhi, Q. L. Wu, Y. L. Yu, Q. Q. Sun and D. Y. Wang, Nanotoxicology, 2016, 10, 1469–1479 CrossRef CAS.
- M. X. Ren, L. Zhao, X. Lv and D. Y. Wang, Nanotoxicology, 2017, 11, 578–590 CrossRef CAS.
- M. Qu, Y. H. Li, Q. L. Wu, Y. K. Xia and D. Y. Wang, Nanotoxicology, 2017, 11, 520–533 CrossRef CAS.
- Q. L. Wu, Y. L. Zhao, J. P. Fang and D. Y. Wang, Nanoscale, 2014, 6, 5894–5906 RSC.
- F. Gottschalk, T. Sonderer, R. W. Scholz and B. Nowack, Environ. Sci. Technol., 2009, 43, 9216–9222 CrossRef CAS.
- N. Mueller and B. Nowack, Environ. Sci. Technol., 2008, 42, 4447–4453 CrossRef CAS.
- Q.-L. Wu, Y.-L. Zhao, Y.-P. Li and D.-Y. Wang, Nanomedicine, 2014, 10, 1263–1271 CrossRef.
- L. T. Zhi, M.-X. Ren, M. Qu, H. Y. Zhang and D. Y. Wang, Sci. Rep., 2016, 6, 39261 CrossRef CAS.
Footnote |
† Electronic supplementary information (ESI) available. See DOI: 10.1039/c7ra09100a |
|
This journal is © The Royal Society of Chemistry 2017 |
Click here to see how this site uses Cookies. View our privacy policy here.