DOI:
10.1039/D4NH00171K
(Review Article)
Nanoscale Horiz., 2024, Advance Article
Oxidative stress modulating nanomaterials and their biochemical roles in nanomedicine
Received
22nd April 2024
, Accepted 8th July 2024
First published on 10th July 2024
Abstract
Many pathological conditions are predominantly associated with oxidative stress, arising from reactive oxygen species (ROS); therefore, the modulation of redox activities has been a key strategy to restore normal tissue functions. Current approaches involve establishing a favorable cellular redox environment through the administration of therapeutic drugs and redox-active nanomaterials (RANs). In particular, RANs not only provide a stable and reliable means of therapeutic delivery but also possess the capacity to finely tune various interconnected components, including radicals, enzymes, proteins, transcription factors, and metabolites. Here, we discuss the roles that engineered RANs play in a spectrum of pathological conditions, such as cancer, neurodegenerative diseases, infections, and inflammation. We visualize the dual functions of RANs as both generator and scavenger of ROS, emphasizing their profound impact on diverse cellular functions. The focus of this review is solely on inorganic redox-active nanomaterials (inorganic RANs). Additionally, we deliberate on the challenges associated with current RANs-based approaches and propose potential research directions for their future clinical translation.
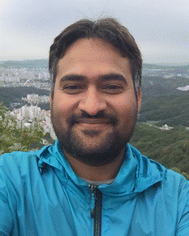 Kapil D. Patel | Kapil D. Patel received his MSc in Physics from the Indian Institute of Technology (IIT) Guwahati, India, in 2010, and PhD in Nanobiomedical Science with a major in Tissue Regeneration Engineering from Dankook University, South Korea, in 2015. He continued his research as a Postdoctoral Research Fellow at the Institute of Tissue Regeneration Engineering (ITREN), Dankook University (2016–2019). He was promoted to Research Professor at Dankook University and worked at University College London (UCL), UK as visiting Research Fellow (2019–2020). He moved to the Korea University, South Korea (2020–2021) as a Research Professor, and then to the University of Bristol, UK as a Senior Research Associate (2021–2023). Currently, he is Research Fellow (Level B) at the Australian National University, Australia. His research interests include the development of functional nano-biomaterials for tissue repair and regeneration, 3D bioprinting, redox-active nanomaterials, and cancer theranostics. |
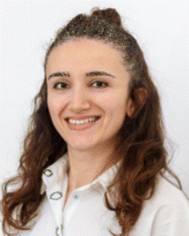 Zalike Keskin-Erdogan | Zalike Keskin Erdogan completed her BEng (2013) and MSc (2015) degree in Bioengineering, specialized in Biomaterials, at Ege University, Izmir, Turkiye. She was awarded a prestigious fully funded scholarship by the National Ministry of Education to pursue her studies abroad, leading her to complete her PhD (2022) at University College London (UCL), UK in the Medical Sciences Faculty, focusing on Biomaterials and Tissue Engineering. Currently, she is a postdoctoral research fellow at Imperial College London (ICL), UK in the Department of Chemical Engineering. Her research expertise spans multiple disciplines, including materials science, biomaterials, cell biology, and tissue engineering, and utilization of biomaterials and hydrogels for 3D cell cultures and cell encapsulation, and microfluidics. |
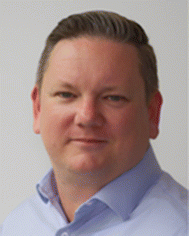 Adam W. Perriman | Adam Perriman is a Professor of Bioengineering at the Australian National University and holds a joint appointments with the School of Cellular and Molecular Medicine, University of Bristol, UK. His position at the ANU is held across the Research School of Chemistry (RSC), and the John Curtin School of Medical Research (JCSMR). He is internationally distinguished for his pioneering research on the construction of novel synthetic biomolecular systems, and his research interests spam the field of biomaterials, biophysics, and synthetic biology. |
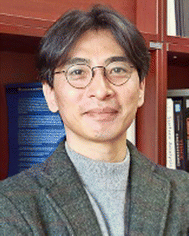 Hae-Won Kim | Hae-Won Kim is Director and Professor of Institute of Tissue Regeneration Engineering at Dankook University. He received his degrees from Seoul National University (BS in 1997, PhD in 2002). Prof. Kim has authored 510 peer-reviewed papers, with 39 000 citations and h-index of 102. He's also written 12 books and holds 165 patents. He has led various prestigious national and international research programs, including Priority Research Center (2009), Global Research Lab (GRL, 2015), UCL Eastman-Korea Joint Center (2017), and Medical Research Center (MRC, 2021). The fundings gained are in total of ∼50 M USD. Currently, he is Editor-in-Chief of Journal of Tissue Engineering (IF 8.2), Associate Editor of Med-X and Frontiers in Bioeng. and Biotech., and editorial board member of many other journals (Biomaterials, Bioactive Materials, etc.). Prof. Kim's research includes therapeutic biomaterials for tissue regeneration and mechanobiological studies on cell-matrix interactions. |
1. Introduction
In the past few decades, nanomaterials with redox-activities (oxidant and antioxidant) have gained massive attention for their therapeutic purposes in deadly diseases such as cancer, strokes, osteoarthritis, and other neurodegenerative disorders such as Alzheimer's, Huntington's, and Parkinson's disease. The ability of nanomaterials to generate reactive oxygen species (ROS) is oxidant-activity, and scavenging of ROS is antioxidant-activity. Moreover, ROS are generated in normal biological processes and can decrease or increase pathological conditions.1–3 Therefore, the dual actions of redox-active foreign particles/nanoparticles as ROS generators and scavengers, can act like a double-edged sworsd to control the ROS in various pathological conditions as presented in schematic Fig. 1. The generation and scavenging of ROS are two important properties of redox-active nanoparticles which have tremendous potential for application in biomedicine as ROS scavengers to aid ischemia-reperfusion injury, stroke, skeletal conditions, myocardial infarction, neurodegeneration, and diabetes.4–7 However, controlling the properties of redox-active nanomaterials (RANs) in vivo and under pathological conditions is challenging.
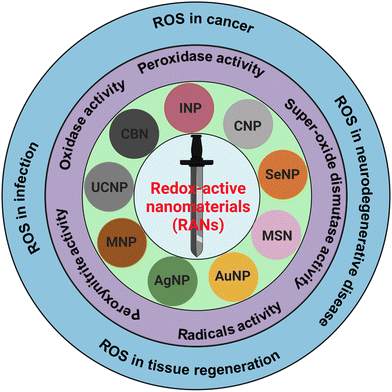 |
| Fig. 1 RANs and their diverse redox-activities and applications in diseases and regenerative medicine. Various nanoparticles including silver nanoparticles (AgNPs) gold nanoparticles (AuNPs), magnetic nanoparticles (MNPs), mesoporous silica-based nanoparticles (MSNs), selenium nanoparticles (SeNPs), cerium oxide nanoparticles (CNPs), iron oxide nanoparticles (INPs), carbon-based nanoparticles (CBNs), up-conversion nanoparticles (UCNPs), and several other nanoparticles are summarized for its redox-activities in biomedicine. | |
Reactive oxygen species (ROS) include free oxygen radicals and other molecules with at least one oxygen atom and one or more unpaired electrons that can exist independently.8 The presence of an unpaired electron in these species makes them extremely unstable and reactive. Radicals can act as oxidants or reductants, depending on whether they give or take an electron. Several common biological functions, such as aerobic metabolism and pathogenic defense mechanism, produce radicals. Furthermore, external exposures including radiation, pollution, dust particles, and smoke from cigarettes can also cause free radical production. Antioxidants are compounds that inhibit oxidation and work in two different ways to combat radicals.9 Enzymatic antioxidants function by oxidizing damaging ROS to produce H2O2, which is then converted to water. Superoxide dismutase (SOD) catalyzes the formation of oxygen and H2O2 from two superoxide anions. Furthermore, vitamin E, vitamin C, and glutathione are examples of non-enzymatic antioxidants that function by directly interacting with radical. For instance, glutathione has a free sulfhydryl group, which makes it a desirable target for radical attacks. The enzyme glutathione reductase then squelches the free radical and recycles the oxidized glutathione.
Redox-active nanomaterials are mainly classified as inorganic, organic, or composite based on their chemical composition. Inorganic redox-responsive nanosystems offer unique physicochemical properties such as robustness, cost-effectiveness, stability, and ease of synthesis and modification.10 These systems can achieve controlled drug release by incorporating reduction- or oxidation-responsive bonds and are generally easier to prepare and modify compared to organic ones.11 Organic redox nanomaterials, on the other hand, are typically more biodegradable and biocompatible.12 Composite redox-responsive nanomaterials, which integrate both inorganic and organic components, combine the strengths of each type, enhancing their physicochemical properties and often exhibiting synergistic effects.13 In this study, our focus will mainly be on inorganic redox-active nanomaterials.
Various types of nanoparticles with a ROS generating or scavenging propensity have recently been synthesized and are currently at different stages of manufacturing. These are also being scrutinized for potential clinical translational application in nanomedicine. These nanomaterials are defined as RANs that include metallic/metallic oxide nanoparticles, carbon-based nanomaterials (CBNs) and some other sources of nanomaterials. Metallic nanoparticles of silver, gold, iron, zinc, palladium, platinum, and ruthenium, as well as nonmetal selenium nanoparticles are known to have intrinsic antioxidant properties, thus, these nanoparticles do not require any functional modification for additional antioxidant function. To enhance the antioxidant activities of these metallic nanoparticles, strategies such as oxygen moiety grafting,14–16 peptide coating,17,18 ligand-exchange,19–21 and chemical conjugation of functional groups have been applied.14,22 Metallic nanoparticles such as magnesium oxide (MgO), titanium dioxide (TiO2), vanadium oxide (V2O5), manganese oxide (MnO2), iron oxide (Fe3O4), copper oxide (CuO), zinc oxide (ZnO), gadolinium oxide (Gd2O3), and cerium oxide (CeO2) have been tested for better antioxidant and catalytic activities. CBNs including fullerenes, carbon nanotubes (CNTs), carbon nanodots and metal-doped carbon nanodots, graphene oxide (GO), reduced graphene oxide (rGO), graphene quantum dots (GQDs) and few layer graphene (FLG), and metal nanoparticles conjugated GO have also been investigated for their antioxidant and redox-activities.23–26 Lately, up-conversion nanoparticles (UCNPs) have also gained significant attention for antioxidant and redox-active applications in nanomedicine.
This review paper highlights oxidative stress in physiological and pathological conditions, and the roles of RANs-associated ROS linked to cellular and molecular mechanisms. The redox-active mechanism of RANs and their roles in oxidase, peroxidase, SOD, radical, and peroxynitrile activities have thoroughly been explored and summarized. Finally, the roles of ROS generated by RANs in various diseases such as cancer, neurodegeneration, infection, and other conditions, along with their role in tissue engineering, have also been discussed in detail.
In recent years, the therapeutic applications of RANs to target ROS have been intensively studied. Current research has emphasized the roles of ROS in some pathological conditions and suggested ROS-based nanomedicine.27–30 However, the review papers mainly focused on ROS's roles in certain diseases’ pathological condition. In this review, we discuss the broad range of nanomaterials and their ROS generating or scavenging properties and underlying mechanisms of ROS in vitro and in vivo disease models. We have also listed nanomaterials with their application in various diseases and regenerative medicine. The family of RANs, various redox-activities and their roles in diseases and regenerative medicine are depicted in Fig. 1.
2. Oxidative stress in biology and medicine
The impact of oxidative stress has been intensively studied in the field of biomedicine over the last few decades.27,31,32 An imbalance between ROS and antioxidants is known as oxidative stress, this can be caused by any form of free radical, oxygen-containing molecules such as superoxide ion/radical, hydrogen peroxide and peroxynitrate. These radicals contain an uneven number of electrons that easily react with proteins/lipids disrupting redox reaction/signalling and eventually causing molecular damage in the body.33 Oxidative stress plays a vital role in normal physiology and the pathophysiology of many life-threatening diseases. Essentially all complex organisms are affected by oxidative stress and free radicals. Although oxidative stress in biology and medicine has been studied for decades, its functional and mechanistic diversity in varying microenvironments has attracted huge attention in the last few years.34–37 Oxidative stress in biology and medicine is part of research of human and animal biology at both the cellular and molecular level.36 Redox homeostasis-based strategy to control the ROS in production has gained great attention due to controllable scale. Lin et al. have developed a radiotherapy-mediated redox homeostasis-controllable nanomedicine for amplifying ferroptosis sensitivity in tumor therapy.38 This strategy can achieve high efficacy of ROS production and modulate the tumor cell microenvironment antioxidant to amplify ferroptosis. Moreover, many of the biological consequences of vitamin and selenium deficiency or excess radiation exposure are thought to be the result of oxidative damages.39 Several reports have also highlighted the role of oxidative damage in human diseases such as cancer, osteoarthritis, chronic inflammatory diseases, neurodegenerative diseases, and retinopathy of prematurity.
2.1. The oxygen paradox
A variety of biological processes, including cell viability/death, cell signalling, differentiation, and the creation of inflammation-related factors, are naturally triggered by ROS during aerobic respiration in the cell. Radicals and non-radicals are two subcategories of biologically significant ROS. A list of free radicals in human biology, its origin, roles, and applications are summarized in Table 1. In a living organism, ROS are created as a result of regular cellular metabolism and external influences including metal toxicity, cigarette smoke, air pollutant, salinity and drought.40
Table 1 Summary of free radicals involved in biological processes
Name of free radicals |
Chemical formula |
Origin and role |
ROS concentration level and disease |
Ref. |
Superoxide radical |
O2˙− |
• Superoxide radical generated as by product of cellular respiration (mitochondrial respiratory chain) and can lead to the formation of other types of ROS. |
• Diffusion-limited rate 2 × 109 M−1 s−1. |
41 and 42 |
• It plays a dual role, at physiological balance level, by product of O2 reduction for the cellular signalling. |
• Superoxide radical-based ROS level in normal cell is in nanomolar (nM) range, while in cancer cell range is in micromolar (μM) |
• At pathological level, induces cellular apoptosis, necrosis, ferroptosis, pyroptosis, and cell death. |
|
Hydroxyl radical |
˙OH |
• Hydroxyl radical is formed from water during various biochemical reactions. |
• Diffusion limit rate for hydroxyl radical is 1.9 × 1010 M−1 s−1. |
43–45 |
• In presence of hydrogen peroxide and iron ions produced hydroxyl radical via Haber–Weiss reaction. |
• Hydroxyl radical concentration range in normal cell is very low nanomolar. |
• It is highly reactive, and can damage the DNA, proteins, and lipids. |
• However, in cancer, inflammation, and neurodegenerative diseases it is in mid high nanomolar to micromolar range. |
• Hydroxyl radical induces polymerization of human fibrinogen. |
• In infection, high nanomolar to low micromolar range. |
Peroxyl radical |
ROO˙ |
• Peroxyl radical commonly formed during oxidation of lipids. |
• Diffusion limit rate for hydroxyl radical is 1.9 × 1010 M−1 s−1. |
46–49 |
• They form as a natural byproduct during the various cellular events including metabolism of lipids, and chain reaction of lipid peroxidation. |
• Peroxyl radical concentration range in normal cell is very low nanomolar. |
• It plays main role in lipid peroxidation, cellular damage, oxidative stress, antioxidant defense, and cell signalling. |
• However, in cancer, inflammation, and neurodegenerative diseases it is in mid high nanomolar to micromolar range. |
|
• In infection, high nanomolar to low micromolar range. |
Hydroperoxyl radical |
HO2˙ |
• Hydroperoxyl radical generate in biological systems during the dismutation of superoxide. |
• Diffusion limit rate for hydroperoxyl radical is 2.3 × 108 M−1 s−1. |
45 and 50–52 |
• Excessive level of hydroperoxyl radicals can leads to oxidative damage to biomolecules including lipids in cell membranes. |
• Hydroperoxyl radical concentration for normal cell is low nanomolar range. |
• Hydroperoxyl radical is associated with various pathological conditions and contribute to the aging process. |
• However for cancer cell, inflammation, are mid to high nanomolar to low micromolar. |
• Hydroperoxyl radicals are also associated with the immune's system defense against pathogens. |
• Neurodegenerative diseases mid to high nanomolar to micromolar range. |
Alkoxyl radical |
RO˙ |
• Alkoxyl radicals are generated during the breakdown of peroxides. |
• Diffusion limit rate for hydroperoxyl radical is 1 × 109 M−1 s−1. |
53–55 |
• Alkoxyl radicals are reactive and participate in redox reactions. |
• Alkoxyl radical concentration range for normal cell is low nanomolar. |
• They involved in the lipid peroxidation process, free radicals damage lipids in cell membranes. |
• However, for cancer and inflammation range is mid to high nanomolar to low micromolar. |
|
• For infection, high nanomolar to low micromolar. |
|
• Neurodegenerative diseases, mid to high nanomolar to micromolar range. |
Carbonate radical |
CO3˙− |
• The carbonate radicals can be formed through different pathways including reaction involving peroxides and other ROS. |
• Diffusion limit rate for carbonate radical is 5.27–7.89 × 105 M−1 s−1. |
56–60 |
• It can generate in presence of hydrogen peroxide and bicarbonate ions. |
• Carbonate radical concentration for normal cell is low picomolar range. |
• Carbonate radicals are highly reactive and can oxidized organic and inorganic molecules and leads to the modification of biomolecular and cellular structure. |
• For cancer, it is low to mid nanomolar. |
• Potentially contribute the oxidative stress in the biological system, |
• For inflammation, it is mid to high nanomolar range. |
|
• Infection, it is high nanomolar range. |
|
• Neurodegenerative diseases, it is mid to high nanomolar to low micromolar range. |
Nitric oxide radical |
NO˙ |
• Nitric oxide radicals are synthesized endogenously by various cell types, primarily through the action of enzyme called nitric oxide synthases (NOS). |
• Diffusion limit rate for nitric oxide radical is 6.7 × 109 M−1 s−1. |
61–64 |
• Nitric oxide radicals act as a cell signalling molecules in various physiological process. |
• Nitric oxide radical for normal cells is 1 to 100 nanomolar range. |
• It is involved in blood vessel dilation in cardiovascular system, and relaxation in smooth muscle cells. |
• For cancer, it is from 20 to 500 nanomolar range. |
• Act as neurotransmission in nervous system, body defense system against pathogens, anti-inflammatory. |
• For inflammation, it is 100 nanomolar to 1 micromolar range. |
|
• Infection, it is 100 nanomolar to 1 micromolar range. |
|
• Neurodegenerative diseases, it is 50 nanomolar to 1 micromolar range. |
Thiyl radical |
RS˙− |
• Thiyl radicals are formed through the homolytic cleavage of sulphur–hydrogen bond. |
• Diffusion limit rate for thiyl radical is 1 × 108 M−1 s−1. |
65–68 |
• In biological system, thiyl radicals are generated during the oxidative stress, and redox reactions involving thiol-containing biomolecules. |
• Thiyl radical concentration range for normal cells is picomolar to low nanomolar range. |
• Thiyl radicals serve as intermediates in the transfer of electrons during various cellular processes including antioxidant defense, cellular signalling, and redox balance in cellular process. |
• Cancer cell, low to mid nanomolar range. |
• Dysregulation of thiyl radicals and thiol redox balance are associated with various diseases including neurodegenerative disorder, and cardiovascular diseases. |
• Inflammation condition, mid to high nanomolar. |
|
• Infection, it is high nanomolar to low micromolar range. |
|
• Neurodegenerative diseases, it is mid to high nanomolar to micromolar range. |
ROS are highly reactive, thus excessively produced ROS can rapidly bind to cell membrane proteins or lipids, nucleic acids, and carbohydrates leading to irreversible structural alteration. Thus, controlling the reducing and oxidizing (redox) states of ROS is critical for cellular activation, viability, proliferation and, ultimately, organ function. Endogenous antioxidant systems exist, in that, enzymatic and non-enzymatic mechanisms normally operate to chelate ROS in healthy normal organisms. Nevertheless, overproduction of ROS in the pathological conditions mentioned above often leads to an imbalance of oxidant and antioxidant levels, resulting in an oxidative stress condition. Highly reactive oxygen radicals can also affect gene expression by up-regulation of redox-sensitive transcription factors and chromatic remodeling through modulation of histone acetylation and deacetylation.69,70
In healthy physiology, the simultaneous oxidation and reduction of O2−˙, and ˙OH form H2O2 which is then broken down by the enzyme glutathione peroxidase in the presence of metals in the reduced state. For instance, mitochondria produce about one-third of the liver's total glutathione peroxidase activity.71 O2−˙, the mediator in an oxidative chain reaction and the product of the one electron reduction of oxygen (from 1O2) molecules, is the precursor to the majority of ROS. Additionally, superoxide, which can be reduced to H2O or ˙OH, catalyzes the dismutation of O2 to produce H2O2.72 Among normal biological processes, most ROS are produced as by-products of the interaction of oxygen with the leaking electrons from the electron transport chain (ETC), in particular protein complexes CI and CIII of the mitochondrial respiratory chain.73 Additionally, metal-catalyzed oxidation reactions generate ROS as intermediates. In the outer shell of the oxygen atom, there are two unpaired electrons. The sequential reduction of oxygen by adding electrons results in the formation of excessive ROS summarized in Fig. 2(a). The breadth of ROS generated by oxygen reduction is demonstrated by the application of photosensitive gold nanoparticles in photodynamic cancer therapy. Fig. 2(b) highlights that the stepwise oxidation and reduction processes triggered by photocatalyst absorption can produce ROS from H2O2 or O2, respectively.
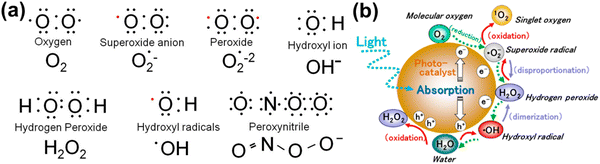 |
| Fig. 2 (a) Electronic structure of some common ROS. The structure of chemical formula and corresponding name are provided, and unpaired electron are designated as a red dot ( ). (b) Reactive oxygen species generated in the photocatalytic redox-reactions, and steps into O2 and H2O2. ROS in free-radical form such as superoxide radicals, hydrogen peroxide, and hydroxyl radicals may be produced sequentially from molecular oxygen (O2) via a stepwise reduction mechanism, and reversibly from hydroxyl radicals, hydrogen peroxide, superoxide radicals, and singlet oxygen as well from water (H2O) via a stepwise oxidation mechanism. Reproduced with permission from Nosaka et al.29 [Copyright 2019, American Chemical Society]. | |
Ranking ROS in terms of their toxicity in mammalian systems involves considering their reactivity, stability, and potential to cause cellular damage. Table 2 is the general ranking of ROS based on their toxicity from most to least harmful:
Table 2 Summary of free radicals with ranking of ROS level toxicity in mammalian cells
ROS species |
Toxicity level |
Description |
Ref. |
Hydroxyl radical (˙OH) |
Extremely high |
• It is one of most reactive ROS, can cause significant damage to DNA, proteins, and lipid due to high reactivity and lac of selectivity. |
74 and 75 |
• Even at very low concentration, can induce severe oxidative damage. |
Peroxynitrite (ONOO−) |
Very high |
• Peroxynitrite is a potent oxidant formed from the reaction of nitric oxide (NO˙) with superoxide (O2˙−). |
76 and 77 |
• It can nitrate tyrosine residues in proteins, leading to functional alteration and damage. |
• Highly damaging to the cells and tissue. |
Superoxide anion (O2˙−). |
High |
• Superoxide is a primarily ROS that can lead to the formation of other, or more reactive species like hydrogen peroxide, hydroxyl radicals. |
78 and 79 |
• It is less reactive than hydroxyl radicals with significant toxicity. |
• It can disrupt the cellular functions and contribute to the formation of other toxic ROS. |
Alkoxyl radical (RO˙) |
Moderate |
• Alkoxy radicals are intermediate in reactivity. |
80 and 81 |
• It is generated from decomposition of organic peroxides and can propagate lipid peroxidation. |
Hydroperoxyl radical (HO2˙) |
Moderate |
• Hydroperoxyl radicals are in equilibrium with superoxide and involved in lipid peroxidation. |
50 and 82 |
• It is less reactive than hydroxyl radicals but still contribute to oxidative stress. |
Carbonate radical (CO3˙−) |
Moderate |
• Carbonate radicals can oxidize biomolecules but are generally less reactive than hydroxyl and peroxynitrite radicals. |
83 and 84 |
• It is formed during the reactions involved peroxynitrite and bicarbonate. |
Nitric oxide radical (NO˙−) |
Low to moderate |
• Nitric oxide radical is less reactive than many other ROS. |
61 and 85 |
• It has physiological importance such as vasodilation, and cell signalling. |
• However, in high concentration or in combination with superoxide, it forms peroxynitrite, which is highly toxic. |
In summary, superoxide and peroxynitrite both are harmful to mammalian cells. Peroxynitrite is generally considered as more toxic due to its reactivity and potential to cause significant damage to the cell membrane. The impact of upregulated ROS levels should be evaluated in the context of the overall redox balance and the cell's ability to neutralize the ROS with antioxidants.
2.2. Molecular switches in oxidative stress
Eukaryotic cells have evolved to harness energy in the form of adenosine triphosphate (ATP). Enzymatic reduction of ATP (catabolic) enables the generation of macromolecular precursor nucleotides, and amino acids (anabolic). ROS are generated within the electron transport chain (ETC) in mitochondria which facilitates this energy utilization, with about 0.1–0.2% of the total O2 consumed through ETC type I and III complexes generating ROS.86,87 Additionally, in the process of energy metabolism, the signalling molecule known as the mammalian target of rapamycin complex 1 (mTORC1) receives signals from both amino acids and glucose.88 Eukaryotic cells frequently include mTORC1 downstream signalling, serving as an important signalling node that connects nutrition sensing and metabolic control. Since cellular metabolism and cell survival are tightly related, signalling pathways for metabolic activity and autophagy may interact despite being functionally independent.89 According to a study by Dibble et al., mTORC1 may serve as a link between the anabolic process and the circumstances that promote cellular development.90 Moreover, mTORC1 signals are integrated with systemic signals, including secreted growth factors, as well as intracellular signals, such as amino acids, glucose, oxygen, and ATP.
Autophagy, a catabolic process responsible for clearing out damaged organelles and unnecessary dysfunctional components in cells, occurs in normal and stressed conditions including viral infection, nutrient deprivation, and genotoxic effects. Recent studies have reported that the oxidative stress created by ROS and reactive nitrogen species (RNS) might converge to trigger sustained autophagy.91 Furthermore, autophagy is closely linked to redox homeostasis and metabolic networks, which involve both nitrosative and oxidative stress.
Thiol-containing proteins can undergo reversible post-translational changes, which are known to be damaging to both biological biomolecules and signal mediators.92 Protein activity is regulated by these thiol-based redox switches, which are also essential for cellular ROS response and adaptation to local and global changes. First responder proteins control redox levels via ROS-specific transcription factors, chaperones, or metabolic enzymes to protect cells from increasing amounts of oxidants, repair the damage, and restore redox homeostasis. In addition, phosphatases and kinases are regulated by redox-regulation, resulting in ROS generation that is considered to be a crucial second messenger in growth, development and differentiation.93 ROS are essential for cell growth and differentiation, and excessive ROS production in the cell causes apoptosis.94 Several studies have reported the roles of ROS during the differentiation of embryonic stem cells (ESC).95 For example, ROS are transiently elevated during the G2/M period of the cell cycle, differing from other differentiated mature cells. It is of interest to highlight that ESCs produce little ATP due to their immature mitochondria, leading ESCs to be presumably resistant to the oxidative stress condition.5 To meet energy demand, ESCs mainly utilize glycolysis instead of mitochondrial oxidative phosphorylation to avoid ROS production. Thus, the produced nicotinamide adenine dinucleotide phosphate (NADPH) from glycolysis can maintain thioredoxin and glutathione to support scavenging ROS.96,97 ROS also plays a crucial role in the differentiation of embryonic hematopoietic stem cells and cardiomyocytes.95,98 Adult stem cells (ASC) have the capability to regenerate in injured tissues throughout their whole life span, retaining a propensity to differentiate into specific lineages. ASC such as neural and mesenchymal stem cells also maintain low levels of ROS by utilizing glycolysis with suppression of mitochondrial oxidative phosphorylation.99–101 The mechanism of ROS scavenging changes under hypoxic conditions; hypoxia-inducible factor-1α (HIF-1α) is produced through oxidative phosphorylation in the presence of elevated ROS. In ASC, Meis Homeobox 1 (MEIS1) regulates HIF-1α; Kocabas et al. have demonstrated that MEIS1 knockout in mice is entirely mediated through ROS and treatment of MEIS1 with the scavenger N-acetylcysteine maintains ASC function.102 However, HIF-1α enhances glycolytic metabolism from oxidative phosphorylation to glycolysis by upregulating gene expression with pyruvate dehydrogenase kinase (PDK1), glucose transporter 1 (GLUT1) and acetate dehydrogenase A (LDHA).103,104 These all factors suggest that the glycolytic metabolism and hypoxia signalling are crucial for ROS regulation.
2.3. Cellular mechanisms of oxidative stress in human health
Oxidative stress plays a significant part in a wide range of diseases, including cancer, inflammation, wound healing, and neurological disorders. An imbalance in the generation and clearance of ROS can lead to oxidative stress, thus directly affecting cellular functions. If the imbalance is severe enough and not controlled by redox homeostasis, then it can lead to serious injuries in the cell and possibly cell death, either by apoptosis or necrosis.105,106 This condition may involve the initiation and progression of certain disease pathologies. In response to tissue injury, cells develop various responses induced by ROS and activate repair mechanisms via modulation of downstream signalling molecules. In this section, we will discuss the mechanisms of oxidative stress within cancer pathology, inflammation, wound healing, and neurodegenerative disease.
Oxidative stress is more prevalent in cancer cells than normal cells.107,108 As mechanisms for normal cell repair and division fail during tumorigenic progression, the cycle of cancer cells speeds up resulting in higher energy demands for fast growth, uncontrolled cell division and cellular migration. The high cellular metabolism and oxygen consumption required to keep these energetic demands result in a higher accumulation of ROS leading to oxidative stress being more prevalent in cancer cells compared to normal cells.109 The radicals and ROS produced in cancer cells induce several effects in the body, such that low concentrations of ROS mediate cell proliferation and tumor progression to the metastatic stage resulting in aggregation of tumor cells. On the other hand, high level of ROS induce a more contradictory outcome, activating cell death pathways in cancers as well as mediating cancer recurrence.110 In the case of cell death initiation, the antioxidant system in cancer cells fail to control excessive ROS generation during oxidative stress resulting in cell death within the tumor. Thus, deciding how to implement ROS to affect tumors is challenging. Their use as part of combination therapies has been suggested, for example, using ROS modulating antioxidants together with chemotherapy could be more effective to deal with the different stages of tumor progression.111
In cancer cells, oxidative stress can be induced by oncogenic signalling, mitochondrial activation, metabolic activity, and increased enzyme activity.112,113 Additionally, cytokines and growth factors such as insulin, platelet-derived growth factor (PDGF), transforming growth factor (TGF), tumor necrosis factor (TNF), and epidermal growth factor (EGF) can drive cancer cells to create intracellular ROS.114–116 Under the hypoxic conditions observed during the intermediate stage of tumor development due to inadequate vascularization, ROS-induced transcription of HIF-1α, a fundamental member of hypoxia-inducing factors, stabilizes the encoded protein, which should be hydroxylated within five minutes by iron-dependent prolyl 4-hydroxylase (PDH), a HIF-1α degrading enzyme.117 As a consequence of HIF-1α activation, several essential genes in cancer progression, such as vascular endothelial growth factor (VEGF) and VEGF-receptors, are induced.118 It has been shown that epidermal growth factor receptors (EGF-receptors) and PDGF-receptors, along with activating mutations in K-ras, can initiate Akt signalling mediated by oxidative stress. In addition, hydrogen peroxide activates Akt either directly or via ROS-induced activation of phosphoinositide-dependent kinase 1 (PDK1), its upstream kinase.119 Additionally, mutations of downstream growth factor receptors, like Kars-RAS 77, 78, can also result in an increase in superoxide generation.120,121 Another downstream effector of numerous growth factor receptors, including EGF receptors and c-Mets, is the tiny Rho GTPase Rac-1.122 Chavda et al. have recently summarized the role of ROS and the molecular mechanism of oxidative stress in cancer and brain stroke.123 It has been well established that oxidative stress plays an important role in tumorigenesis via inflammation, immune evasion, autophagy and apoptosis control through signalling pathway regulation, angiogenesis, and drug resistance. The mitochondrial ROS cause of cell apoptosis and the oxidative-stress-mediated molecular mechanism of cancer progression are presented in Fig. 3.
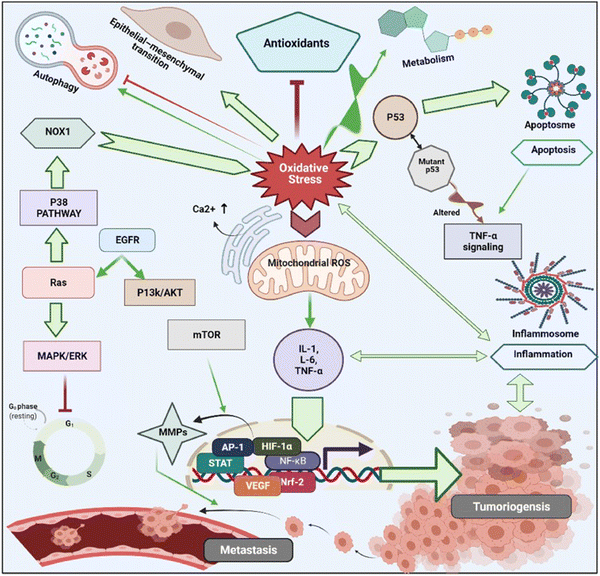 |
| Fig. 3 Schematic illustration of various cell signalling pathways associated to oxidative stress mediated progression of tumorigenesis and metastasis. Under oxidative stress, intracellular ROS activates cancer cell surviving signalling cascades such as MAPK/ERK1/2, p38, JNK, PI3K/Akt, which leads to activation of transcription factors such as NF-κB, MMPs, AP-1, HIF-1α, STAT, Nrf2, VEGF. These transcriptional factors cause imperative pathophysiologies aggravating carcinogenesis, and cancer metastasis. Reproduced with permission from Chavda et al.123 [Copyright 2022, Elsevier]. | |
The primary function of inflammation is to protect the body from infectious pathogens. It is usually not a disease condition itself but is commonly observed in various pathological conditions such as hepatitis B & C, malaria, dengue, and tuberculosis (TB) infections, autoimmune diseases, radiation, or toxic chemical damage, and even in obesity. Inflammation also occurs in non-pathological processes, including tissue rearrangement, elimination of cellular waste, and tissue regeneration. Recent investigations have shown that the progression of many chronic diseases is closely related to the situation of oxidative stress, where the resulting protein oxidation accelerates inflammatory responses.124,125 Protein-oxidation induces the release of inflammatory signalling molecules including peroxiredoxins 2 (PRDX2).126 During this response, proinflammatory mediators like tumor necrosis factor-α (TNF-α) are released through activation of disintegrin, metalloproteinase-17 (ADAM-17), and PRDX2, a ubiquitous redox-active intracellular enzyme which also acts as a redox-dependent inflammatory mediator to activate macrophages after the release of TNF-α. It is of note that chronic inflammatory responses are commonly observed in insulin resistance, type 2 diabetes mellitus (T2DM), and cardiovascular diseases.
Wound healing is another redox-regulated biological process involving continuous and extending phases of homeostasis, inflammatory related events, cell proliferation, and new tissue formation.127 Immediately after blood vessel injury, platelet aggregation and activation are initiated, forming blood clots that temporarily seal the wound site. The subsequent inflammatory response involves different immune cells such as neutrophils and monocytes that are recruited into the wound. These immune cells secrete proteolytic enzymes and proinflammatory cytokines together with excessive ROS, which are essential to kill invading bacteria and other microorganisms. During normal aging or oxidative-stress-related pathological conditions such as diabetes, alcohol abuse, smoking, or infectious disease, the normal inflammatory response can be delayed or impaired.128 Some interesting studies have suggested that ROS might be crucial regulators involved in all stages of wound healing process. Although ROS function as important regulators during wound healing, over production of ROS could cause molecular damage, disrupting the wound healing process resulting in the formation of chronic wounds. In fact, many studies have suggested that antioxidant strategies are effective and beneficial during the wound healing inflammatory response. Antioxidant strategies such as mitochondrial-targeted peptides like elamipretide, can protect against mitochondrial dysfunction and inflammation by activating NOD-like family receptors, including the pyrin domain 3 (NLRP3) inflammasome and inhibiting the nuclear factor-kappa B (NF-κB) signalling pathway, and the nuclear factor (erythroid derived 2)-like 2 (Nrf2).129 Furthermore, sustained oxidative stress accelerates the inflammatory response in the chronic period of wound healing via ROS-stimulated chemotaxis and migration of neutrophil and macrophage cells to the wound area by expressing adhesion molecules in blood vessels. ROS can directly affect cell migration, proliferation, and extracellular matrix (ECM) production in fibroblast and keratinocytes.130
In neurodegenerative disorders including AD, PD, HD, and ALS, the ROS-induced oxidative stress is extremely high with comparatively low levels of antioxidants. Particularly in AD among other neurodegenerative disorders mentioned above, ROS-induced oxidative stress plays a critical role in the accumulation and deposition of β-amyloid peptide (Aβ peptide). The aggregation of Aβ peptides can lead to mitochondrial dysfunction and energy failure prior to plaque formation in the brain via impairing the electron transport system complex I and IV.131,132 Similarly, α-synuclein, a presynaptic protein in PD that forms Lewy bodies (LBs), is misfolded and aggregated causing a decrease of mitochondrial functions.133,134 Importantly, the PD-related genes such as PINK1, DJ-1, LRRK2, and PARK2 (Parkin), are all involved in homeostasis of mitochondrial ROS.135,136 Moreover, the induction of mitochondrial recruitment of Parkin by mitochondrial ROS plays an important role in the PINK1/Parkin-related mitophagy, as well as mutations or deficiency of PINK1.137 HD is caused by a mutation in the Huntingtin (HTT) gene located on chromosome 4 (4p63).138 This mutation leads to the expansion of CAG trinucleotide repeats, causing the aggregation of Huntingtin proteins and eventually neuronal death in the brain at the early stages of HD. Oxidative damage is suggested as one of the major pathological mechanisms due to the higher lipid concentrations and high energy requirement in the HD brain.139 Mutant HTT proteins serve as of the initiator of ROS, due to oxidized proteins in partially purified mHTT aggregates.140 Thus, oxidative damage has been suggested as one of the major pathological mechanisms in the early stages of HD progression.141 The roles of oxidative stress in neurodegenerative diseases such as AD, PD, and HD and corresponding cellular and molecular details are summarized in Fig. 4.
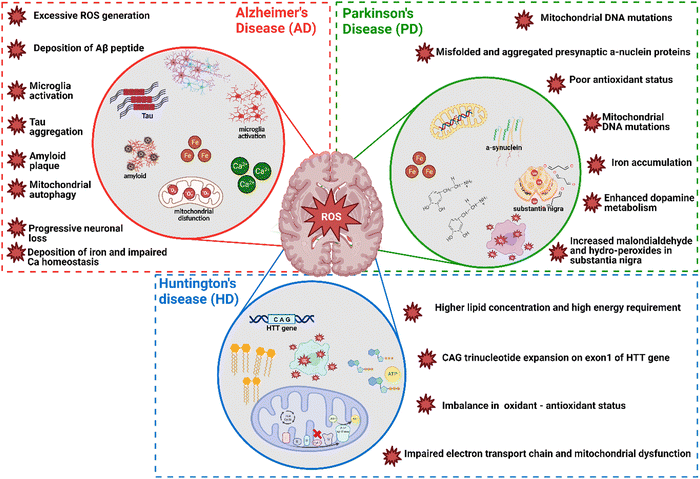 |
| Fig. 4 The role of oxidative stress in neurodegenerative disease namely AD, PD and HD; three circles in the figure represent three main neurodegenerative diseases, red star ROS symbols provide the detailed role of oxidative stress and associated cellular and molecular effects. Figure created by authors using BioRender.com. | |
Reactive oxygen species play a crucial role in the ageing process as pathogenic components. These extremely reactive chemicals, such as superoxide (a free radical) and hydrogen peroxide (a non-radical molecule), are naturally produced during cellular metabolism, especially in the mitochondria. Under normal physiological conditions cells have a homeostatic equilibrium in between ROS and presence of antioxidant mechanisms. As we age this equilibrium shifts towards higher levels of ROS as a result of a decrease in mitochondrial activity and antioxidant capability.
Elevated levels of ROS are detrimental and can result in significant harm to cellular and organelle membranes, DNA, and proteins.142 Gradual oxidation over a period of time and decline in ATP production cause damage to cells and tissues which is one of the causes of aging. ROS are involved in the development of other age-related illnesses, including neurological conditions like Alzheimer's and Parkinson's diseases, cardiovascular diseases, and malignancies.143,144 These molecules have the ability to initiate and alter various cellular signalling pathways that result in apoptosis, inflammation and cellular senescence, hence affecting the process of ageing and the progression of diseases.145
ROS have a crucial impact on the ageing process and the emergence of age-related ailments through the initiation of oxidative harm and the disturbance of cellular equilibrium. Gaining a comprehensive understanding of the processes involved in the generation and reduction of ROS is essential for the development of effective treatment approaches to address the effects of ageing and its related diseases.
3. Nanomaterials possessing antioxidant and redox-activities
Antioxidants are molecules/compounds/materials that can react with radicals by donating an electron,8 radical addition,46 H-atom donation,146 as well as regeneration by other reducing agents,147 preventing unfavorable biochemical chain reactions by converting them to nontoxic metabolites. In other words, antioxidants can bind to oxidizable molecules, protecting cells by delaying or inhibiting their autoxidation.148 Antioxidants can therefore reduce oxidative stress, playing a key role in the mediation and control of ROS induced deadly diseases. They can be categorized as preventive antioxidants and chain breaking antioxidants. Preventative antioxidants are a heterogeneous class of molecules/compounds including metal chelators,149 hydroperoxide-decomposing agents,150 and glutathione peroxidase;151 their main role is to interrupt the initiation rate of ROS generation.152 On the other hand, the main role of chain-breaking antioxidants is to slow down (or inhibit) the autooxidation. The antioxidants that break chains are also known as radical-trapping antioxidants. For example, phenols are the best-known chain-breaking antioxidant as they can rapidly trap 2-preoxyl radicals per molecule. Tocopherols (vitamin E), ascorbate (Vitamin C) flavonoids, and stilbenes are some other examples of chain-breaking antioxidants.153
Some best practices to measure oxidative-stress induced by nanoparticles, it is important to have strong and sensitive assays to detect reactive oxygen species and related oxidative damage.8,145 There are several potential assays that can be used, including the dichloro-dihydro-fluorescein diacetate (DCFH-DA) assay, the thiobarbituric acid reactive substances (TBARS) assay, and the glutathione (GSH/GSSG) assay. The DCFH-DA assay is commonly used to measure oxidative stress and assess ROS generation.154–156 The TBARS assay measures malondialdehyde (MDA), a byproduct of lipid peroxidation caused by nanoparticles.157,158 Additionally, the GSH/GSSG assay measures reduced (GSH) and oxidized (GSSG) glutathione levels, which can give insight into the cellular redox state.159,160 According to the previous report, it is important to include both positive (e.g., H2O2 treatment) and negative controls (untreated cells) when conducting these assays.155
In the last several decades, numerous nanomaterials have been developed and evaluated for their antioxidant properties to see if they can provide defense against oxidative damage.153 Nanomaterials such as metals/nonmetals (Cu, Ag, Au, Pt, and Se), metal oxides (TiO2, ZnO, Fe3O4, CeO2), carbon-based nanomaterials (fullerenes, CNTs, GO, GQDs, nanodiamonds), nanomaterials developed as delivery tools (Ce@SiO2, Ce@GO), and UCNP have shown intrinsic redox-activities like superoxide dismutase (SOD) or catalase-like activities often associated in radicals trapping. Grafting or modifying nanomaterials with low molecular weight antioxidants can sometimes make them antioxidants. In this section, we have categorized the above nanomaterials and discussed their antioxidant/redox-activities and mechanism of action in different physiological conditions.
3.1. Metallic nanomaterials
Metallic nanoparticles (silver, gold, palladium, platinum, and ruthenium) and nonmetal (selenium) mostly possess intrinsic antioxidant properties and these nanoparticles do not require any functional modification for having antioxidant properties. These nanoparticles do not need to be functionally modified in order to exhibit antioxidant activities and they exhibit oxidase-like activity under acidic conditions and in the presence of oxidizing agents like 2,2-azinobis(3-ethylbenzothizoline-6-sulfonic acid) and 3,3′,5,5′-tetramethylbenzidine (TMB).161–165 Unlike antioxidant activity, which only occurs at neutral or basic pH levels, this redox-activity occurs at acidic pH levels, similar to that of peroxidase enzymes. A Fenton-like reaction on the surface releases OH radicals, resulting in the peroxidase activity.29
3.1.1. Silver nanoparticles. Silver nanoparticles (AgNPs) are well known antibacterial and antioxidant materials used for biomedicine. Keshari et al. have demonstrated the antioxidant property of AgNPs by employing 2,2-diphenyl-1-picrylhydrazyl, hydrogen peroxide, hydroxyl radical and superoxide radical scavenging methods.166 They suggest that the antioxidant activity of AgNPs is caused by the presence of bioactive-compound (molecules/functional) groups on their surface. The mechanism underlying the antioxidant activity of AgNPs is complicated and seems to vary depending on synthesis protocols, physical parameters, and particle surface chemistry. In a study by Prasad et al., a complex antioxidant enzyme network was proposed as a possible mechanism of biosynthesis for AgNPs.167 Moreover, internalization of AgNPs into cells by endocytosis is followed by release of the compounds from the surface of particles, particle aggregation, and surface oxidation, resulting in the release of silver ions.168 The interaction of AgNPs with membrane proteins activates signalling pathways and inhibits the cell proliferation in cancer cells.169,170 AgNPs and surface oxidized silver oxide exhibit great affinity for sulfur containing functional groups and are thus able to bind with proteins, enabling direct modulation of antioxidant enzymes. Moreover, AgNPs themselves and other nanoparticles doped with AgNPs have been proven as effective antibacterial agents, acting as an antineoplastic drug with anti-apoptotic activity. ROS production depends on the size of AgNPs, with the smaller size of nanoparticles exhibiting higher ROS production. In line with this finding, Onodera et al. have recently found that AgNPs-induced ROS production occurs in a size dependent manner.171 They found that the ROS were rapidly generated right after treatment with 1 nm and 70 nm AgNPs for 5 and 60 min; but there was no ROS production by AgNO3. They also detected ROS from whole cell lysate and mitochondria at 5 and 60 min after AgNPs exposure; this was the first study reporting the local production of ROS induced by AgNPs. Similarly, Carlson et al. also reported a significantly higher ROS production together with a decrease to undetectable levels of the reduced state of glutathione (GSH), an important antioxidant, in macrophages exposed to smaller (15 nm) AgNPs as opposed to their larger counterparts.172 This interaction of AgNPs with cellular proteins via thiol groups has been suggested to interfering with thiol based redox switches that regulate ROS production, such as ROS-specific transcriptional regulators, and GSH antioxidant defense mechanisms by interacting with GSH reductase.173 The higher surface area of smaller AgNPs relative to their volume have been suggested as one of the characteristics making these particles more reactive for interactions with thiol groups. A significant reduction of both protein and non-protein sulfhydryl (thiol) groups has also been reported in vivo studies upon exposure to AgNPs.174 Moreover, smaller size AgNP were reported to cause larger loss of the mitochondrial membrane potential exacerbating the already known inactivation of the enzymatic complexes in the ETC and resulting in higher ROS production.175Several studies have further reported AgNPs-induced ROS generation in mouse fibroblasts and human hepatocytes, showing reduced membrane potential of mitochondria with subsequent release of cytochrome C into the cytosol followed by JNK activation and Bax translocation.176,177 Contrary to this result antiapoptotic protein Bcl2 is highly expressed in HCT116 cells (human colon cancer cells) that are resistant to AgNPs.176 Ag+ from AgNPs directly mediates the synthesis of ROS, such as superoxide, hydroxyl radicals and hydrogen peroxide, in free-cell environments.178,179 This report has been further supported by Mendis et al. showing ROS generated from AgNPs can lead to cell membrane disruption, mitochondrial dysfunction, and DNA damage.180 Another study by Chang et al. has suggested the antibacterial properties of AgNPs result from the formation of multiple forms of ROS, observing a reduction in antibacterial activity on addition of the ROS scavenging enzymes super oxide dismutase and catalase.181 Inoue et al. have found that the bacterial activity induced by the introduction of Ag+ into zeolite is mediated by four forms of ROS under aerated conditions, the activity of which can again specifically be decreased by scavenger addition.182
Despite evidence for ROS generation from AgNPs and its antibacterial effect, the potential toxicity of Ag+, the structure of AgNPs, and their combinatorial effect with other factors confounds a clear understanding of AgNPs functional mechanisms. Jones et al. have investigated the possible reactions involved in ROS generation by AgNPs and the potential interaction between Ag+ and ROS once they have been generated.183 Henglein group has reported a possible reformation of AgNPs by O2˙− as a result of O2˙− mediated charging of AgNPs.184,185 A schematic illustration of AgNPs acting as an electron pool during ROS generation is shown in Fig. 5(a), proposing potential interactions among AgNPs, Ag+, H2O2 and O2˙−. A second source of ROS is the leakage of O2˙− through cell membranes, which can be neutralized by natural antioxidants as shown in Fig. 5(b). Since AgNPs and Ag+ have a strong affinity for thiol groups (–SH) in cysteine residues, it is conceivable for AgNPs to be internalized and disrupt mitochondrial function through altered membrane permeability, disruption of the electron transfer chain, and disruption of mitochondrial membrane proteins.
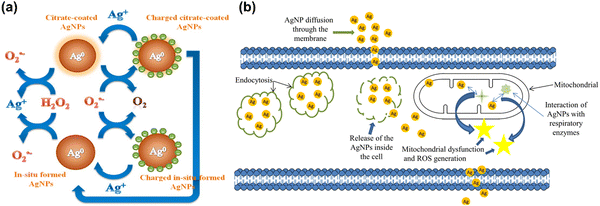 |
| Fig. 5 (a) Schematic illustration of the action of silver nanoparticles in generation of ROS in the form of radical. Reproduced with permission from He et al.179 [Copyright 2012, American Chemical Society]. (b) Interactions of AgNPs with cellular membranes and mitochondria and generation of ROS, causing cellular apoptosis. Reproduced with permission from Flores-López et al.186 [Copyright 2018, Wiley]. | |
Gold nanoparticles (AuNPs) have been employed extensively in nanomedicine applications, for example biosensing,187 drug delivery,188 theranostics,189 biolabeling,190 wound healing,191,192 and medicine.193 The development of plant extract and bio-object derived green synthesized AuNPs with high redox-ability has started to gain interest among researchers. Green synthesized AuNPs are mainly derived from bacteria, virus, yeast, fungi, algae, and plants.194,195 Plant extract-based nanoparticles (also known as phytosynthesized NPs) are more effective compared to microorganism sources, and their preparation method requires fewer additional reagents.196–198 Stozhko et al. have recently introduced a phytosynthetic method to create AuNPs using leaf extract (phyto-AuNPs) and demonstrated their antioxidant activity together with details of the phytosynthesis kinetics, particle size, and dispersibility of the created nanoparticles.199 They found that smaller phyto-AuNPs produced a higher antioxidant activity with an increase of the absolute value of zeta-potential. Nie et al. has developed antioxidant-functionalized AuNPs using self-assembly of thiol ligands derived from Trolox (Vitamin E analogue).19 Surprisingly, the Vitamin E (tocopherol) analogue-functionalized AuNPs has shown strong reactivity towards 2,2-diphenyl-1-picrylhydrazyl radicals (DPPH˙), about eight times higher than AuNPs alone. Hamelian et al. have developed Thyme-derived green AuNPs as a reducing agent, exhibiting antibacterial and antioxidant activity specifically for DPPH˙.200 Quercetin capped AuNPs have also been synthesized using a green route by Milanezi et al. for antioxidant, antibacterial application. The antioxidant activity of quercetin both free and on AuNPs has been proven by free radical scavenging methods using ABTS, DPPH, and nitric oxide. In addition, quercetin-capped AuNPs (IR50 0.37 μg mL−1) demonstrated higher antioxidant activity than free quercetin (IR50 0.57 μg mL−1) in nitric oxide free radical scavenging method.201 In a recent study by Nieves et al. have reviewed silver chalcogenide-based hybrid nanoparticles for its synthesis methodologies, and thorough biomedical applications including bio-imaging, theranostic agents, and biosensors.202 For example, Mantri et al. synthesized iodine-doped silver shell/gold core metal nanorod for measuring the oxidative stress in vivo via photoacoustic imaging.203
3.1.2. Gold nanoparticles. Gold nanoparticles (AuNPs) are attractive and promising nanocarriers in nanomedicine due to their unique size/shape-dependent optical properties, high stability, low cytotoxicity, and easy surface modification.204,205 In addition, AuNPs are very useful in drug delivery for targeting and controlling of drug release and serve as a sensitive imaging tool for early detection of diseases or injury.188,206,207 Of note, AuNPs themselves have no redox-activities, however, they can induce a redox-response by interacting with other molecules via their unique surface properties.27,30 According to recent reports, endocytosis of AuNPs causes intracellular ROS production, which in turn sets off oxidative stress in cells.208,209 AuNPs are suggested as an ideal platform for electrochemical biosensing due to their redox-catalytic properties, which improve electron transport across a wide range of electroactive biological species, primarily redox-proteins, without using mediators.210–212 In fact, Lee et al. have used AuNPs to image intracellular ROS after modifying the surface of AuNPs with fluorescent dye-labeled hyaluronic acid.213 Monodispersed polystyrene (PS) particles of varying sizes and chemical functional groups on their surfaces can be assessed for cellular toxicity by ultrasensitive detection of intracellular ROS. PEGylation of PSNP surfaces was also tested for its detection of intracellular ROS production. Further, PSNPs intracellular ROS levels were well correlated with their cytotoxicity, apoptosis-inducing activity, and cellular uptake. It was found that linear and branched polyethylenimine (PEI) can generate ROS intracellularly and can cause cellular damage. Higashi et al. have developed enzyme-free electrochemiluminescence (ECL) immunosensing detection using approximately 5 nm size AuNPs to generate ROS in an aqueous solution of trishydroxymethylaminomethane (Tris).214 They demonstrated the catalytic pathways of new ECL detection and ROS generation can be varied and chemically regulated with parameters such as shape, size, concentration, and dispersant types of AuNPs. AuNPs were employed to an ECL-based enzyme-free sandwich immunoassay using magnetic beads (MBs), and disposable screen-printed electrode (SPE) chips as shown in Fig. 6(a). It is easy to modify AuNPs with biomolecules such as antibodies, thus they can be used for various biosensing applications through functional immobilization of biomolecules/antibodies.215,216
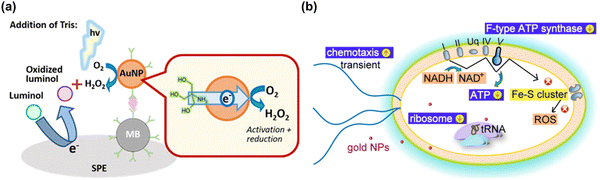 |
| Fig. 6 (a) A schematic illustration of gold nanoparticle-generated ROS controlled on a disposable screen-printed electrode using an enzyme-free electrochemiluminescence-based immunoassay. Reproduced with permission from Higashi et al.214 [Copyright 2018, American Chemical Society]. (b) Schematic diagram illustrating the of cellular mechanism of bactericidal gold nanoparticles on E. coli, and subsequent gold nanoparticles inducing the reduction of oxidative phosphorylation pathway (F-type ATP synthase and ATP level) and ribosome pathways, and the transient up-regulation of chemotaxis. During the process, the gold nanoparticles do not induce any change in ROS-related processes. Reproduced with permission from Cui et al.217 [Copyright 2012, Elsevier]. | |
In addition to the ROS generating effect of AuNPs, many research groups have reported a ROS independent effect of AuNPs. AuNPs have been shown to be capable modulating cell interaction, and to have an apoptotic effect on Escherichia coli via a redox imbalance followed by decreased GSH without ROS generation.35,217 By transcriptomic and proteomic approaches, the Cui group has investigated the molecular mechanism by which AuNPs exert their antibacterial activity against multidrug-resistant Gram-negative bacteria.218 The antibacterial actions by AuNPs could occur through two routes; first, by changing membrane potential and inhibiting ATP synthase causing a subsequent metabolic decline through decreased ATP; second, by inhibiting ribosome binding to tRNA, causing a collapse of biological processes as demonstrated in Fig. 6(b). The antibacterial activity by AuNPs seems to be a bactericidal antibiotic effect that is independent from ROS.219 Mateo et al. have investigated the cytotoxic role of oxidative stress in human tumor cells. which is suggested to involve an AuNP-induced decrease of superoxide dismutase activity.220 Overall, AgNPs and AuNPs have potential for some important biological applications including for antibiotic effect, drug delivery, biosensing, and cancer theranostics, however they are not very effective catalytically due to low coordination numbers and so frequently require complex surface modifications with biologically active molecules.
3.1.3. Platinum and palladium nanoparticles. Platinum and palladium nanoparticles (PtNPs and PdNPs, respectively) have antioxidant properties due to their strong catalytic activity which can quench radical.221,222 In fact, PAPLAL, a mixture of PdNPs and PtNPs, has been used to treat chronic diseases for several decades. Shibuya et al. used a diseased condition in mice related to aging to study the anti-aging effects of PAPLAL.114 For Sod1−/−-deficient (Sod1−/−) mice with thin skin brought on by enhanced lipid peroxidation, PAPLAL transdermal therapy is beneficial. Col1a1, MMP2, IL-6, TNF-α, and p53 gene expression, which is abnormal in the skin of Sod1−/− mice, was restored by PAPLAL. This group has also shown PtNPs with high SOD and catalase activities, while PdNPs were demonstrated to have weak SOD and catalase activity. Compared to the SOD and catalase activity of PtNPs, which decreased right after being oxidized in the air, the mixture of PAPLAL exhibited stronger SOD and catalase activity even after oxidation, suggesting PdNPs possibly inhibit the degradation of PtNPs by oxidation.223 Elhusseiny et al. have demonstrated the antitumor and antimicrobial activity of a complex of PtNPs and PdNPs against human cell lines such as breast carcinoma (MCF7), liver carcinoma (HEPG2), and colon carcinoma (HCT 116) cells.224 In their study, palladium complexes of polyamides containing sulfones were found to be the most potent for antibacterial and antifungal effects, whereas platinum complexes with flexible sulfone and ether flexible linkages and chloro (chloride) groups demonstrated high antitumor and antimicrobial agents. Further, Kora et al. have synthesized environmentally friendly PdNPs using gum ghatti (Anogeissus latifolia), for better application as an antioxidant and catalyst.225 These green synthesized PdNPs showed relatively high antioxidant activity even with a lower dose of nanoparticles, and homogenous catalytic activity was also confirmed using reduction of dyes such as, methylene blue, Coomassie brilliant blue G-250, methyl orange, and 4-nitrophenol with sodium borohydride. The presence of large surfaces and the large proportion of metal atoms on the surfaces of most metallic nanoparticles results in strong catalytic activity in hydrogenation, hydration, and oxidation reactions.226,227 These nanoparticles that possess SOD and catalase activity may be useful for biomedical research and clinical practice, as well as in material science and engineering.228,229
3.1.4. Ruthenium nanoparticles. Ruthenium nanoparticles (RuNPs) and ruthenium-containing nanomaterials (Ru-cNMs) combined with platinum and palladium or with nonmetal phosphorous and oxygen have been tested for antioxidant, anticancer, antimicrobial activities, and a wide range of catalytic applications.230,231 Srivastava et al. have reported multiple metal nanoparticles synthesized by bacterial-extract (Pseudomonas aeruginosa) SM1.232 Green bioextract-derived RuNPs possesses antioxidant and catalytic activity. RuNPs synthesized from Nephorlepis biserrata showed significant antifungal activity via DPPH, ABTS, SORS, and HAS assays. Cao et al. have reported the ROS scavenging activities of RuNPs.233 They found that RuNPs can break down H2O2, scavenge hydroxyl radical, superoxide, singlet oxygen, 2,2′-azino-bis(3-ethylbenzothiazoline-6-sulfonic acid) derived radical (ABTS˙+) and 1,1-diphenyl-2-picrylhydrazyl radicals (˙DPPH), as well as exert cytoprotective effects against H2O2-induced oxidative stress in vitro. In addition, the oxidation of o-phenylenediamine (OPD), and dopamine hydrochloride (DA) by H2O2 to produce the colorimetric products in the aqueous solution can also be catalyzed by RuNPs, which also have inherent HRP-like, oxidase-like mimetic capabilities.
3.1.5. Selenium nanoparticles. Selenium nanoparticles (SeNPs) are one of the more promising and intensively studied materials in recent years. From the Greek word ‘Selene’, which means moon, selenium was discovered as a byproduct of sulphuric acid synthesis by Jöns Jacob Berzelius in 1817.234 Selenium is a non-metal which is categorized as non-metal, physical appeared as a red/brown colored powder.235 The atomic number of selenium is 34 and its electronic distribution is 34Se = 1s2, 2s2 2p6, 3s2 3p6 3d10, 4s2 4p4. It has various oxidation states like 2−, 0, 2+, 4+, 6+. Because of its varying oxidation states, selenium is very useful for biomedical applications. The dietary form of selenium known as sodium selenite is a common dietary source of selenium for animals and humans, in which it is a trace element.236 Selenium-based compounds such as seleno–amino acids and various synthetic organo-selenium compounds are known to be effective tumorigenesis inhibitors in many animal and cell models.237–239 When selenium is injected to live animals in in vivo conditions, it is primarily found as selenoproteins that play critical roles in cellular redox-reaction, detoxification, and immune-system protection.240 There are 25 selenoproteins discovered in humans and 24 kinds in rodents.241 It is highly expressed in the human brain, functioning as an antioxidant and neuroprotectant for preventing the onset and progression of Alzheimer's disease.242Selenium is known to be involved in various biological processes including the immune response. Some pathological conditions associated with the immune system have also been affected by selenium content level, and its different salt forms. In the past, concerns have been raised about the metabolism of selenium compounds into metabolites and potential hereditary factors influencing their utilization. Due to low levels of selenium in soils and food, selenium deficiency is uncommon in the United States and Canada,243 while it is also common in some regions of China, New Zealand, and portions of Europe and Russia.244 Recent studies have observed selenium deficiency in immune-related diseases and suggested selenium supplementation to solve the health issues associated with selenium deficiency, although the cellular and molecular mechanisms underlying the effects of selenium in immune-related diseases are not fully understood.245 One possible mechanism by selenium is to activate leukocytes in an immune response including adherence, migration, phagocytosis, and cytokine secretion at an optimal dose. The redox-activities of selenoproteins seem very important in modulating cell signalling in these immune responses. Thus, the redox-activities of selenium-based materials could be a promising therapy for immunity-related pathologies including chronic inflammation, by generating reduced forms of thioredoxin to balance the reduced and oxidized molecules within the cell.245–247
The range of oxidation states accessible to selenium (2−, 0, 2+, 4+, 6+) and its electronegativity are the two properties which are important in redox biology. Because selenium possesses different oxidation states, it has interesting redox activities and therefore a capability to generate ROS. In proteins, selenium can be incorporated in the place of sulfur in cysteine residues forming selenocysteine (Se-Cysteine), the electronegativity of which is lower than cysteine (−0.23 V Cysteine, −0.38 V Se-Cysteine). This incorporation occurs by the action of diverse antioxidant enzymes like thioredoxin reductase, glutathione peroxidase, and selenoprotein.248 Selenium acts as a redox center for all of these enzymes which are essential for biochemical activities. Compared to disulfide, diselenide has a much lower binding energy (H–Se–Se–H, 172 kJ mol−1) than disulfide (H–S–S–H, 240 kJ mol−1).249 The lower binding energy of diselenide bond allows having redox-activity companying with visible light radiation in an effective manner.250–252 Diselenide can produce seleninic acid or selenol under redox conditions by the cleavage of the diselenide bond, and selenium radicals under stress conditions such as under irradiation or heating could be generated. Selenium can be reduced by thiol compounds or oxidized by oxygen leading to ROS production in both reactions and subsequently to apoptotic cell death. However, studies have reported that selenium has a narrow therapeutic window for clinical application.253–255
SeNPs made from sodium selenite (Na2SeO3) can be an alternative form to modulate oxidative stress, presenting an advantage for therapeutic purposes. The ionization of sodium selenite to sodium ions and selenite (SeO32−) is the most common fabrication method (eqn (1)). Selenite has a high affinity with glutathione (GSH), producing selenodiglutathione (GS–Se–GS) as reported previously (eqn (2)).256
|
 | (1) |
|
SeO32− + 4GSH + 2H+ → GS–Se–GS + GSSG + 3H2O
| (2) |
|
GS–Se–GS + GSH → GS–SeH + GSSG
| (3) |
In the presence of excessive GSH, selenodiglutathione is further reduced by glutathione into glutathioselenol (GS–SeH/GH–Se
−) as shown in
eqn (3). Subsequently, glutathioselenol is dismutated to selenium and glutathione as shown in
eqn (4). Selenite also reacts with thiols, generating ROS such as O
2−˙, ˙OH and H
2O
2.
256 Therefore, it is crucial to understand the detailed reaction mechanism of selenite/selenium nanoparticle ROS generation and its byproducts, as well as to identify intermediates in these reactions to understand their role in biochemical processes.
Selenium has been tested in several disease models including ischemic cerebral stroke, an acute brain degeneration with a high mortality rate and no appropriate treatment so far.257,258 Amani et al. have developed biodegradable SeNPs to target ischemic brain stroke, demonstrating a dramatic effect. They found that SeNPs reduced brain edema, protected axons and promoted axonal growth, and enhanced remyelination in the hippocampal area.257 The group has also suggested an effective delivery of SeNPs to target a specific area with minimal side effects. SeNPs possibly modulate cellular signalling pathways in inflammatory and metabolic responses including the ubiquitin-proteasome system (ERK5), Tsc1/Tsc2 complex, biquitin-proteasome system (ERK5), FoxO1, and wnt/β-catenine. The activation of JAC2/STAT3 and Adamts1 are important in inflammatory responses. Studies suggest that SeNPs are promising therapeutics for cerebral stroke via its antioxidant and anti-inflammatory properties.
Redox homeostasis is critical in living organisms and excessive ROS damage cellular biomolecules during oxidative stress. GSH is a tripeptide of L-glutamate, glycine, and L-cysteine, which plays a critical role in many biological functions in mammals. GSH is a major nonprotein thiol in organisms that is required for intracellular redox homeostasis. Importantly, GSH and ˙OH are natural counterparts functioning as reducing and oxidizing agents, respectively. Yang et al. have developed selenium-conjugated graphene quantum dots (Se-GQDs) based on an ultrasensitive reversible redox-fluorescent switch for detecting ˙OH and reductive GSH in aqueous solution and living cells.259 They found that the fluorescence of Se-GQDs is statically quenched by ˙OH, causing a condition known as fluorescence OFF condition that is brought on by Se–Se groups right after reduction of Se–Se groups to C–Se groups upon GSH addition, and fluorescence can be turned ON in the reverse process. This fluorescence-based switch can be useful for detecting redox-activity in cells.
Although the cytotoxic effects of selenium against many cancer cells are thought to be due to its ROS producing activities,260–262 the biochemical mechanism involved in tumor suppression by selenium remains to be studied. Wang et al. have developed biodegradable and pH-responsive selenium-doped hydroxyapatite (SeHA) nanoparticles for osteosarcoma treatment, using in vitro and in vivo osteosarcoma models.263 The molecular mechanism involved in suppression of osteosarcoma is presented in Fig. 7(a) and (b). In another study, Li et al. have demonstrated selenium-containing amphiphiles reduced and stabilized AuNPs suggesting that the selenium in the particles possibly induces high levels of ROS, leading to cancer cell death.264 Zheng et al. have further investigated the therapeutic use of SeNPs in a co-delivery system of selenium and small interfering RNAs (siRNAs).265 This approach aims to overcome drug resistance in breast cancer therapy mostly caused by P-glycoprotein (P-gp) and class III β-tubulin. For co-delivery of selenium and siRNAs (anti-P-gp and anti-β-tubulin III), they have designed layered double hydroxide (LDH) nanoparticles and found a more efficient gene-silencing effect than siRNA alone by a significant downregulation of P-gp and β-tubulin III expression. In addition, apoptotic cells undergo morphological change with an increase of intracellular ROS and altered signalling pathways such as Bcl-2/Bax, caspase-3, PI2K/AKT/mTOR and MAPK/ERK pathways. A similar trial was also performed for dual delivery of siRNAs and cisplatin (DDD) to A549/DDP cells, a breast cancer cell line exhibiting multidrug resistance (MRD). The co-delivery of gene and drug (siRNA and DDP) in A549/DDP cells showed a synergistic effect in anti-cancer therapy, leading to a decreased expression of P-gp and MRP.266 Although selenium conjugated nanomaterials have been intensively explored in many disease conditions, the diagnostic or therapeutic applications of these NPs require more investigation. Furthermore, the exact mechanisms of SeNPs in certain pathological conditions remain unclear. Redox-activity and biocompatibility are considered as the main properties of selenium-based NPs that are applicable for clinical applications. The current challenges of SeNP-based therapies in clinical applications are limiting due to a lack of information on dosing accuracy, potential cytotoxicity, and metabolism in the body.
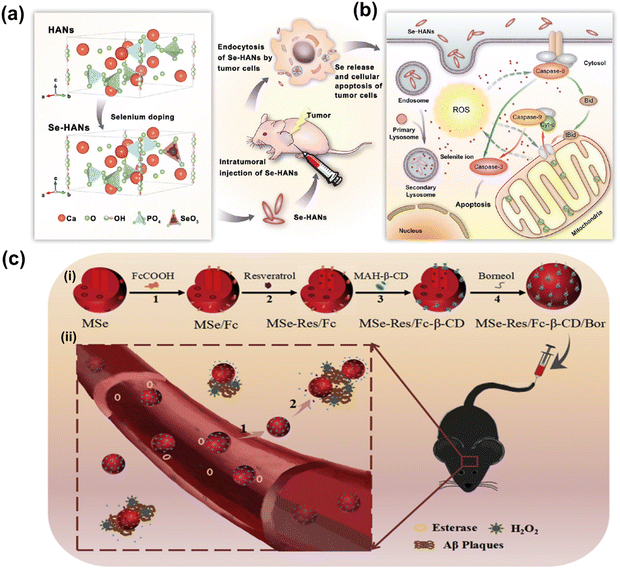 |
| Fig. 7 (a) Schematic chemical structural diagram represents the synthesis and acting mechanism of antitumor nanoparticles (Se-HANs) using selenite to replace phosphate groups in hydroxyapatite nanoparticles (HANs). Next, intratumorally injection of Se-HANs into xenograft osteosarcoma mice model. (b) Further, cellular schematic diagram illustrates the non-specific endocytosis of Se-HANs into tumor and subsequent rapid degradation in lysosome (acidic pH) to release selenium. Finally, selenium-induced, caspase-dependent apoptosis pathway activates the cellular apoptosis together orchestrated with the intracellular ROS generation. Reproduced with permission from Wang et al.263 [Copyright 2016, American Chemical Society]. (c) (i) Step-by-step schematic illustration of synthesis and various surface functionalization of MNSe-Res/Fc-β-CD/Bor; including (1) Ferrocene loading into mesoporous nanoselenium (MNSe/Fc), (2) Further loading with resveratrol to MNSe/Fc. (3) Then surface functionalized with MAH modifiedβ-CD on to the surface of MNSe-Res/Fc. (4) Finally, grafting of borneol onto the NPs surface via ester bond to create drug delivery carrier for AD with capability to penetrate the blood–brain-barrier. (ii) Schematic illustration of in vivo circulation of MNSe-Res/Fc-β-CD/Bor in mice: (1) the release of borneol of from the nanocarrier (MNSe-Res/Fc-β-CD/Bor) and passing the blood–brain barrier (BBB). (2) Targeting the amyloid plaques and controlled release of resveratrol by hydrogen peroxide from MNSe-Res/Fc-β-CD/Bor. Reproduced with permission from Sun et al.267 [Copyright 2019, Elsevier]. | |
Sun et al. have created an innovative drug delivery system that targets Bor and utilizes Fc-β-CD loaded with Res to treat AD through multiple channels.267 The researchers have shown that using MNSe-Res/Fc-β-CD/Bor can effectively prevent the aggregation of Aβ proteins, reduce oxidative stress, and suppress tau hyperphosphorylation. Moreover, this treatment successfully protected neurons and restored impaired memory in APP/PS1 mice. It is interesting to note that the MSe/Fc-CD/Bor loaded with rivastigmine (Riv) displayed a higher pharmacokinetic index than Riv alone. A schematic illustration of synthesized MNSe-Res/Fc-β-CD/Bor for gradual release of drug is presented in Fig. 7(c). The in vivo therapeutic approach is illustrated in Fig. 7(c), where the compound MNSe-Res/Fc-β-CD/Bor was administered via the tail vein of mice with the APP/PS1 model. The Bor present on the nano-system's surface contains an ester bond, which can be broken down by esterases in the bloodstream. This drug delivery system utilizing nanomaterials has been demonstrated to cross the blood–brain barrier and accumulate consistently in the brain. Treatment with MNSe-Res/Fc-β-CD/Bor effectively restored the diminished cognitive function in APP/PS1 mice, accompanied by a decrease in the total levels of Aβ, hyperphosphorylated tau, and the loss of neurons in the brain.
3.2. Metal oxide nanomaterials
Metal oxide nanoparticles such as magnesium oxide (MgO), titanium dioxide (TiO2), vanadium oxide (V2O5), manganese oxide (MnO2), iron oxide (Fe3O4), copper oxide (CuO), zinc oxide (ZnO), gadolinium oxide (Gd2O3), and cerium oxide (CeO2) have antioxidant and catalytic properties. By developing pH-responsive oxide nanomaterials, we can modulate oxidative stress inside the cells through redox-chemistry.268 It is important to note that the reaction mechanisms for these types are not well understood.29,269 The reaction of these metal oxide NPs can be modulated by a given condition, which can be a major factor to favour either antioxidant or redox reaction. Understanding the detailed conditions will provide important information for the use of metal oxide NPs as redox-regulators, however the exact reaction mechanisms of metal oxide NPs need to be thoroughly investigated.29,268,269 In this section, we will discuss and detail the mechanisms of their antioxidant and redox activities on the physiology and pathology of living organisms.
3.2.1. Magnesium oxide nanoparticles. Magnesium oxide nanoparticles (MgONPs) are promising solid-base catalysts that have gained significant attention due to their superior catalytic reaction. The catalytic property of MgONPs is highly dependent on their size, shape, morphology, crystallinity, and surface area. Sutradhar et al. have developed several different forms of MgONPs such as flower shaped nanoflakes, house of card structures, spheres, cubes, and hexagonal plates via calcination of magnesium carbonate hydrates as an intermediate.270 The same group have reported that the amount and distribution of carbonate ions in the crystal is the key factor in the formation of different morphologies of MgONPs using hydrothermal fabrication. Considering that the newly synthesized MgONPs contain many edges and corners, step edges and step corners, as well as many base sites, the surface hydroxyl group is highly coordinated with O2− sites, which are considered to be active basic sites in heterogeneous catalysts. Dobrucka et al. have prepared MgONPs using Artemisia abrotonum herb extract and examined their antioxidant and photocatalytic and antioxidant activities.271 The antioxidant activity of these herb based MgONPs was tested using DPPP radicals by reducing 2,2-diphenyl-1-picrylhydrazyl (DPPH) into stable nitrogen radicals. Any oxygen atom donor can result in reduction of DPPH, which result in the loss of the violet color of the solution. Antioxidant effectiveness in scavenging radical is indicated by the degree of color change in the DPPH solution immediately following the reaction with antioxidants. Similarly, Sushma et al. have investigated the antioxidant activity of MgONPs synthesized from Clitoria ternatea.272 Kiranmai et al. have demonstrated that the exposure of rats to MgONPs decreases the rats’ antioxidant capacity including SOD and catalytic activity in blood samples of the animals in a dose-dependent manner compared to a PBS (with 1% Tween 80) exposed control group.273 The reduction in the antioxidant capacity in MgONPs-exposed rats indicated the antioxidant defense mechanism is triggered by the nanoparticles, suggesting a possibly hazardous effect of nanoparticles in case of chronic exposure. Recently, Kuo et al. have produced surface defect-rich MgONPs to enhance surface oxygen vacancies which exhibited greater antibacterials activity against Escherichia coli, and Staphylococcus aureus after incorporation in cellulose acetate thin film.274 Thus, by increasing the oxygen vacancies on MgONPs surface and thereby releasing a greater number of superoxide anions may increase the antibacterial activity.
3.2.2. Titanium oxide nanoparticles. Titanium oxide nanoparticles (TiO2NPs) with varied size, shape, and morphology have been widely studied for practical applications including cosmetics (including as skin protectors from ultraviolet (UV) light), food components, inks, toothpastes, pharmaceuticals, and antibacterial agents.275,276 Titanium and its related materials are also widely used in biomedical devices and products due to their high Young's modulus and excellent biocompatibility, for example, as replacement materials for hard tissues or replacement for cardiac and cardiovascular tissues.277–280 TiO2NPs have been often used as metal implants for dental and orthopedic implants. However, the biocompatibility of TiO2NPs and their effect on osteoblast cells have not been fully understood.281 Titanium oxide-based nanomaterials have been suggested as a potential photosensitizer, with potential use for photodynamic cancer therapy due to their hydrophobic nature and electron–hole-pair generating propensity under UV radiation. The electron–hole pair being generated from TiO2NP inside or in proximity to tissue or cells under UV exposure reacts with ROS lessening the intracellular oxidative stress.282 The best application of this mechanism is the use of TiO2 nanomaterials to protect skin from sunlight. Moreover, TiO2 nanoparticles can also be used for pollution remediation, using light induced radical generation to remove dyes and phenols from water.283,284 Successful application of ROS generated from TiO2NPs as a cytotoxic reagent has been reported in human cervical adenocarcinoma, leukemia, breast, and lung cancer, and hepatocarcinoma cell lines.285–288 Some other studies have implicated the antioxidant potential of TiO2NPs by generation of ROS.289,290 TiO2NPs may also be suitable for antibacterial application; ROS generated by photocatalysis at the particle surface can cause an imbalance between antioxidant and oxidant, and the ROS themselves can damage proteins, lipids, and DNA in bacterial cells.291 Li et al. have studied the mechanism of TiO2NP-generated ROS toxicity together with a mechanical characterization of the particles using electron spin resonance (ESR).292 Photobleaching of TiO2NPs results in ROS generation on the surface of photoexcited TiO2NPs. In detail, TiO2NPs absorbs light in the ultraviolet A (UVA) (320–400 nm) and ultraviolet B (290–320 nm) ranges of the electromagnetic spectrum. When TiO2NPs absorb photons with energy equal to or higher than their energy band gap (3.0 and 3.2 eV for rutile and anatase phase, respectively), electrons become excited and jump from the valence band to the conduction band resulting in the formation of an electron–hole pair.293 When the holes in the valence band are created, they become highly oxidizing and can produce hydroxyl radicals when in contact with water or hydroxide ions. While, the conduction band electrons can further convert oxygen molecules to form superoxide radical anions.294 This redox-activity of TiO2NPs has a significant impact in various biological systems. Recently, Cai et al. have reported that repeated oral administration of inorganic nanoparticles such as TiO2NPs, Au, and NaYF4 at low concentration can promote lipid degradation and alleviate steatosis in the liver of male mice.295 The low dose of these nanoparticles evokes an unusual antioxidant response in hepatocytes via up-regulation of Ces2h expression and resulted rapid ester hydrolysis. The authors claim that the low dose administration of these nanoparticles may serve as a potential candidate for metabolic regulation treatment.
3.2.3. Vanadium oxide nanoparticles. The outstanding structural versatility of vanadium oxide nanoparticles (V2O5NPs) has led to significant progress in their use as a catalyst in electrochemical devices developments. V2O5NPs, also known as vanadium pentaoxide, are regarded as a promising candidate to serve as cathode materials for lithium-ion batteries. Various types of V2O5 have been developed including one dimensional structures (nanorods,296 nanowires,297 nanotubes,298 nanofibers,299,300 nanobelts301,302), two dimensional nanosheets,303 and three dimensional hollow and porous nanostructures.304–306 Recently, these V2O5NPs structures have been tested for biomedical applications. For example, Natalio et al. have investigated vanadium halo-peroxidase mimicking V2O5NPs to inhibit the formation of bacterial biofilms.307 They found that V2O5 nanowires with vanadium haloperoxidase-like activity are capable of simultaneously producing hypobromous acid (HBrO) and 1O2, with potent antibacterial properties. Furthermore, HBrO may react with other H2O2 molecules being able to produce 1O2 in the absence of an organic acceptor, which has a stronger antibacterial activity adequate for prevention of biofilm formation. Vernekar et al. has also shown that V2O5 nanowires (vanadia nanowires) act like glutathione peroxidase, an antioxidant enzyme that consumes cellular glutathione, suggesting V2O5NPs could be used as potential therapeutics in many oxidative stress associated diseases including aging, cardiovascular disorder, and other neurological disorders such as AD and PD.308 They have also shown that vanadium nanowires are readily internalized into mammalian cells of various organs, exhibiting a robust enzyme like activity through ROS scavenging under intrinsic and extrinsic oxidative stress conditions. Vanadia nanowire nanozymes maintain redox balance without perturbing cellular antioxidant defense, thus protecting cells against harmful oxidative stress. In general, V2O5NPs exhibit vanadium haloperoxidase mimetic activity in response to H2O2 in the presence of vanadium haloperoxidase substrates (i.e., tyrosine/iodide or dopamine/iodide) producing vanadium peroxido species on the surface of vanadia nanowires.307,308 Recently, Das et al. have prepared the V2O5NPs using the microwave assisted polyol method and investigated for cytotoxicity against various cancer cell lines, and endothelial cells.309 They found that the developed nanoparticle was anti-angiogenic in both in vitro and in vivo assays, and showed significant inhibition of melanoma B16F10 cells proliferation, and subcutaneous tumor growth in C57BL6/J mice. Through various biological assays they found that the generation of superoxide radicals from nanoparticles causes the up-regulation of p53 protein and down-regulation of surviving protein might be the underlying mechanism for anti-cancer activity of V2O5NPs.
3.2.4. Manganese oxide nanoparticles. Manganese oxide nanoparticles (MnO2NPs/Mn3O4NPs) have gained significant interest for a broad-range of applications because of their unique properties including magnetism,310,311 catalytic activity,312,313 and high energy density.314 Nanosized MnO2NPs have long been applied for drug delivery,315 as imaging tools,316 for chelation of heavy metal ions,317 and as optical sensors.318 Recently, manganese-based nanomaterials have been utilized as nanomedicines in regenerative medicine due to their ability to scavenge intracellular ROS to minimize oxidative cell damage, and also for application in rheumatoid arthritis treatment.319–322 Alarifi et al. have evaluated MnO2NP-induced DNA damage and cytotoxic effect on human neuronal (SH-SY5Y) cells.323 They found that the MnO2NPs induced ROS generation and decreased membrane potential of the mitochondria in the SH-SY5Y cells in a time and dose dependent manner. Furthermore, lipid peroxide SOD, and catalase (CAT) activities were increased with a subsequent decrease of glutathione also occurring in a dose and time dependent manner. According to Singh et al., Mn3O4NPs generated by endothelial nitric oxide synthase contain multienzyme redox-activity that is shape dependent.324 They found that Mn3O4NPs mimic the functions of CAT and SOD glutathione peroxidase (GPx), the cellular antioxidant enzymes. This enzyme activity by Mn3O4NPs depends on the size, morphology, and surface area of the NPs, as well as the redox properties of metal ions. Furthermore, Mn3O4NPs do not affect the level of nitric oxide in endothelial cells unlike manganese complexes that have antioxidant enzyme-like activity which change nitric oxide bioavailability. The same group has also reported that Mn3O4NPs with flower-like morphology (“nanoflowers”), and a higher ratio of Mn3+/Mn2+ that was obtained by an oxidation with NaIO4, exhibit an enhanced catalytic activity compared to other materials with a lower ratio of Mn3+/Mn2+.325 Environmental pH can be a key factor in controlling the switch between Fenton chemistry (low pH) and catalase activity (high pH). Tootoonchi et al. have developed manganese oxide nanoparticles with four different crystal structures, namely, poorly ordered (crystalline) MnO2, cryptomelane α-MnO2, birnessite δ-MnO2, and hausmannite Mn3O4 for use as cytoprotective, oxygen generating agents, and to act as enzyme mimics in vitro.326 The capability of manganese oxide nanoparticles to produce oxygen, their rate of SOD, and their biocompatibility were all highly associated with their power to protect cells from damage. The cellular mechanisms of cytoprotective effects by these nanozymes remain to be investigated. The capability of MnO2NPs to convert H2O2 was utilized by Gordijo et al. to fabricate an autonomous insulin delivery system with a variable release rate dependent on blood glucose concentration.327 In these works, a proteinaceous membrane was created by crosslinking bovine serum albumin (BSA)-MnO2NP aggregates in the presence of poly(N-isopropylacrylamide-co-methacrylic acid) nanoparticles and the enzymes glucose oxidase (GOx) and CAT. The presence of both the MnO2NPs and CAT in the membrane enabled the immobilized GOx to trigger a pH change in the presence of glucose without inhibition by H2O2 formed during the glucose oxidation. This pH change caused a reversible contraction of the poly(N-isopropylacrylamide-co-methacrylic acid) nanoparticles, thereby increasing the porosity of the membrane and increasing the rate of insulin release in proportion to glucose concentration.328 Moreover, Prasad et al. have increased radiation therapy tumor elimination by using several MnO2NP formulations to treat hypoxia and glycolytic acidosis, two conditions commonly observed in tumor environment.329
3.2.5. Cobalt oxide nanoparticles. Cobalt oxide nanoparticles (Co3O4NPs) have been investigated for their potential antioxidant properties in various research studies. It has been used to create flexible supercapacitors due to their high specific capacitance and surface-to-volume ratio.330 Co3O4NPs have unique catalytic, magnetic, and optical properties which also make them suitable for biomedical applications. The antioxidant-like activity of three different shapes of Co3O4NP was found to decrease in the order of nanoplates, nanorods, and nanocubes, being inversely proportional to their redox-potentials.251 This result suggests that electron transfer during H2O2 reduction by Co3O4 might be a rate-determining step in the antioxidant activity. Furthermore, cobalt oxide nanoparticles have been investigated for scavenging of free radicals and helping to neutralize ROS like superoxide, and hydroxyl radicals.331,332 In addition to their potential antioxidant activity, cobalt oxide nanoparticles may possess anti-inflammatory activity, which is directly linked to oxidative stress, materials with anti-inflammatory effects can directly contribute to antioxidant defense.333 However, cobalt oxide nanoparticles induced cytotoxicity by release of Co2+ ions which strongly triggered the generation of ROS, and caspase cascade in cell lines. The toxicity of the cobalt oxide nanoparticles can be minimized by surface modification approach using active agents/biopolymers or doping in nanoparticles through reducing the Co2+ ions release.334
3.2.6. Iron oxide nanoparticles. Magnetic nanoparticles (MNPs), particularly iron oxide nanoparticles (Fe3O4NPs), have been intensively applied for drug delivery, cancer therapy, and tissue regeneration with different physicochemical properties. The redox-activity of MNPs depends on the oxidation state of iron, and a variety of physicochemical parameters including the particle's size, shape, surface chemistry, and magnetism. In a sequential reaction that produces ROS, the oxidation states of Fe (2+ and 3+) are important.27 Among many different forms of iron oxide nanoparticles, maghemite (Fe2O3) and magnetite (Fe3O4) are the most common. Recently, the application of these iron oxide NPs has gained great attention in cancer therapy as magnetic resonance imaging (MRI), and therapeutic agents by altering their redox status and increasing ROS levels, thus destroying cancer cells.335,336 The ROS generating properties of the NPs have also been demonstrated to sensitize cardiomyocyte cells (H9c2) to oxidative stress; this effect has been suggested to enhance cancer therapies.337 Considering the differential biological effects of transient ROS generation, intense oxidative insults by ROS are more cytotoxic to cancer cells compare to normal cells.338 Moreover, oxidative stress generated by exogenous agents can disrupt redox homeostasis in the cell and induce an imbalanced redox condition leading to a selective cancer toxicity.339,340 Recently, Torres-Herrero et al. have designed and synthesized the nanohybrid nanoparticles made of co-encapsulation of a prodrug converting therapeutic enzyme as HRP with nano-heater as MNPs in the silica matrix for cancer treatment.341 These nanohybrid MNPs achieve heat-triggered control of glucose oxidase (GOx)-peroxidase nanoenzyme cascade reaction was recently reported for production of intracellular ROS.342A recent study has further investigated the underlying mechanisms of anti-cancer effects induced by magnetite nanoparticles, demonstrating that ROS produced by magnetite NPs lead to mitochondrial damage and genotoxic effects in A549 cells. Mathias et al. have further demonstrated the cytotoxic mechanisms of magnetite-mediated ROS generation using A549 and H1299 human lung cancer cells.340 The study has clearly shown that ROS generated by magnetite nanoparticles induce gene mutations, without a direct effect on cell death. Hauser et al. have utilized ROS produced by iron oxide nanoparticles to enhance the efficacy of radiation therapy, as a combination treatment.343 In this study, they used iron oxide nanoparticles coated with TAT, a cell penetrating peptide, to avoid degradation by lysosomes after being internalized by cancer cells, enabling intracellular hydroxyl radical formation. In addition, TAT-coated MNPs have been shown to produce significantly more ROS compared to uncoated MNPs in A549 lung carcinoma cells. Combined treatment of TAT-functionalized MNPs and radiation therapy resulted in a synergistic effect due to the increased lysosomal permeability, ROS generation, and loss of mitochondrial integrity and function.344 In particular, when greater amounts of superoxide anions are generated under increased cellular respiration in mitochondria, MNPs may show synergic effects on the formation of the highly reactive hydroxyl radicals as described in Fig. 8. Aranda et al. have also suggested that the magnetite nanoparticles might generate higher levels of ROS and oxidative stress due to magnetite possessing both Fe3+ and Fe2+ ions, while maghemite has mostly ferric iron ions (Fe3+).154 For this reason, magnetite nanoparticles have been more frequently used to generate intracellular ROS.345–348
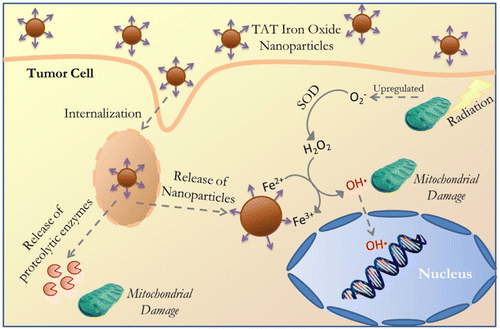 |
| Fig. 8 Schematic illustration of the cellular internalization of TAT-conjugated iron oxide via lysosomal membrane permeabilization followed by NP and proteolytic enzyme delivery into the cytoplasm and subsequent interactions with organelles (nucleus and mitochondria) and catalytic reaction to produce the hydroxyl radical. Reproduced with permission from Hauser et al.344 [Copyright 2016, Elsevier]. | |
3.2.7. Copper oxide nanoparticles. Copper is a very interesting metal which has been applied in various fields including biomedicine, electronics, the metal industry, and for medical device development. In normal conditions, copper possesses two oxidative states, namely Cu+ and Cu2+, thus it is a versatile candidate for biomedical applications. Copper oxide nanoparticles (CuONPs) have been explored for their antibacterial, angiogenic, and antitumor properties. For example, Sarkar et al. have biosynthesized CuONPs from copper sulfate using an (Adiantum lunulatum plant extract to provide boost the enzyme-based antioxidant defenses of Lens culinaris seeds).349 Another group, Sukumar et al., have prepared environmentally-friendly rice-shaped CuONPs from the seed extract of Caesalpinia bonducella and evaluated their electrocatalytic properties by electrochemical measurement of riboflavin and antibacterial activity.350 They found that the CuONPs have better antibacterial effects against S. aureus than Aeromonas. The effect of nano-particle shape has also been investigated, with plate like CuONPs found to exhibit the best antibacterial activities against Gram-negative and Gram-positive bacteria compared to grain or needle shaped NPs. It was suggested that differing extents of membrane damage were the cause of this due to geometry dependent interactions with the cell surface.351 Nasrollahzadeh et al. have demonstrated another efficient and environmentally-friendly way to synthesis CuONPs using an aqueous extraction of Gundelia tournefortii and have also evaluated their catalytic activity.352 As a result of an energy level transition available within the ring of the cinnamoyl system, and also because of absorbance of the benzyl system, the UV-visible spectrum of Gundelia tournefortii extract shows specific signals of phenolics within the plant as two distinct absorbances at 385 and 235 nm, respectively, relating to π → π* transitions. The presence of these excited energy states demonstrates that polyphenolics are a potential source of antioxidant activity suitable for coating CuONPs. Rehana et al. have synthesized CuONPs using medically important plant extracts using a different chemical method for comparative evaluation of their antioxidant and anticancer activities.353 They screened the performance of phytochemicals produced from leaf extracts for incorporation into CuONPs. They have also evaluated the antioxidant activity of CuONPs using ABBT, DPPP, and H2O2 assays and found that the CuONPs formed in the presence of plant extract have a significantly higher ability to scavenge radicals compared to chemically synthesized CuONPs. Possibly, this is due to secondary metabolites from the plant extracts residing at the NP surface, including flavonoids, carbohydrates, glycosides, saponins, phenolic compounds, and tannins. CuONPs primarily generate ROS via two mechanisms; first, reaction at the nanoparticle surface, and second, by copper ion release and reaction.354 Due to the short lifetime of ROS, the site of ROS production in both situations needs to be in close proximity to cause damage. Although these two mechanisms have suggested the details of how each component damages DNA, the exact mechanism remain unclear.355,356 Angelè-Martínez et al. have evaluated the ROS generation ability of CuONPs and evaluated them using a DNA damage assay and electron paramagnetic resonance (EPR) spectroscopy.354 To address the question of whether ROS are generated from the particle surface or by released ions, they separated CuONPs from their supernatant containing any dissolved copper and then measure the degree of DNA damage in the presence of H2O2, ascorbate, or both. They have found that NP induced DNA damage in the presence of ascorbate was much higher than that of the dissolved copper in the supernatant and that ROS generation primarily occurs on the surface of nanoparticles. EPR analysis also suggested that the ROS generated from the surface of CuONPs in the presence of ascorbate are mainly ascorbyl, hydroxyl, and superoxide radicals. It is likely that CuONPs generate ROS through both Fenton-like reactions at the surface and through Haber–Weiss reaction by dissolved copper ions, respectively.292,357
3.2.8. Zinc oxide nanoparticles. Zinc is known to be an essential element in human health that activates many enzymes such as carbonic anhydrase, carboxypeptidase, and alcohol dehydrogenase.358 Interestingly, zinc oxide nanoparticles (ZnONPs) have long been used in various industrial products such as paints and coating materials,359 cosmetics,360 plant fertilizers,361 electrical devices,362 drug delivery carriers,363,364 as a suitable agent for bioimaging365 and targeted gene therapy,366,367 as sensors for detecting pollutants368 and for environmental remediation.369 Particularly, ZnONPs have gained tremendous attention in many biomedical applications such as target-specific delivery of anticancer drugs, imaging, and for antibacterial applications due to their low-cost synthesis, versatile properties due to variable shape, size, and morphology, biocompatibility, and antioxidant activity. The biological effects induced by ZnONPs mainly depend on the size, shape, morphology, concentration, pH, and surface chemistry of the particles. ZnONPs are also known to promote ROS production in the form of superoxide radicals and H2O2 in the absence of photochemical energy in plants.370 ROS generated from ZnONPs can induce membrane lipid peroxidation subsequent to cellular damage, which is considered to be the primary reason for their plant toxicity.371 Nagajyothi et al. have demonstrated the antioxidant and anti-inflammatory activities of ZnONPs synthesized from Polygala tenuifolia root extract.372 They discovered that ZnONPs produced by Polygala tenuifolia inhibited the lipopolysaccharide (LPS)-induced synthesis of IL-1β and IL-6 proteins in a dosage-dependent manner, but dramatically reduced the expression of TNF-α at a concentration of 1 mg mL−1. However, ZnONPs have no effect on the level of anti-inflammatory related cytokines such as IL-1β, IL-6 and TNF-α in LPS treated RAW 294.7 cells and exhibited a dose dependent inhibitory effect on pro-inflammatory cytokines related to mRNA expression. The surface of green-synthesized ZnONPs is covered with phytochemicals which function as reducing agents, allowing ZnONPs to be less toxic compared to chemically synthesized ZnONPs.190 Shoeb et al. have synthesized ZnONPs from egg albumin that show an anticandidal effect by ROS produced from ZnONPs.373 They found that the anticandidal activity of ZnONPs was correlated with ROS production in a dose dependent manner which was confirmed by the protection of histidine against ROS. Moreover, ZnONPs can be easily internalized into cells via electrostatic interaction; once internalized, they produce excessive ROS leading to cell death.374 Another beneficial effect of ZnONPs is their ability to generate ROS without light or under only visible light.375,376 The antibacterial activities of ZnONPs seem to be caused by Zn2+ ions being easily internalized by the cell, destroying cell organelles, DNA, and proteins. Xia and Gupta et al. have suggested that the ROS generation from ZnONPs occurs right at the surface of the NPs causing a subsequent change of electronic transfer in mitochondria, affecting cellular metabolism.208,377
3.2.9. Gadolinium oxide nanoparticles. Gadolinium oxide nanoparticles (Gd2O3NPs) have been well studied for magnetic resonance imaging (MRI) application in cancer theranostics, but there has only been limited study of their antioxidant and redox-activities.378 Seo et al. have investigated the nanoradiator effect of Gd2O3NPs in enhancing ROS production by radio-sensitizing the high-atomic number (high-Z) nanoparticle to enhancing therapeutic treatment.379 The generation of ROS in a Gd2O3NPs solution was measured by either monochromatic synchrotron X-rays or protons with a power of 45 MeV. They found that both photoexcitation and proton impact can enhance ROS production in a dose dependent manner, ranging from a fold change in production from 1.6–1.94 compared with radiation. Radiation therapy benefits from the strong Gd–Gd interatomic de-excitation-driven nanoradiator effect because it increases the formation of ROS by irradiated nanoparticles. Gd-based contrast agents have been reported to be cytotoxic mainly because of free Gd ions, i.e. those unbound from the crystal structure or incorporated into organic compounds.380 This toxicity could be controlled with low doses of Gd. To improve the contrast ability (relaxivity), gadolinium content should enhanced in multifunctional nanoparticles.381
3.2.10. Cerium oxide nanoparticles. Cerium oxide nanoparticles (CeO2NPs), also known as ceria, have gained considerable attention due to their unique catalytic activities (i.e. oxidant and antioxidant). The catalytic activities of CeO2NPs are due to their different oxidation states (Ce(IV)) and (Ce(III)). In CeO2NPs, cerium atoms are positioned in a cubic crystalline fluorite lattice structure, where Ce3+ and its corresponding oxygen vacancies are localized at the surface of NPs.382 CeO2NPs are very well-known antioxidants with a great potential to be therapeutic candidates in oxidative stress related diseases. The redox-reaction cycles of CeO2NPs between Ce(III) and Ce(IV) oxidation states allow catalytic reactions with superoxide and H2O2, mimicking superoxide dismutase and catalase, the two key antioxidant enzymes which abate all noxious intracellular ROS via a self-regeneration mechanism. The main reasons for interest in CeO2NPs are unique physical and chemical characteristics such as redox activities, and oxygen buffering capacity. Furthermore, the cellular interactions, and cytotoxicity of CeO2NPs based redox-active nanoparticles are strongly associated and dependent on surface chemistry.383 Therefore, CeO2NPs are known to be well tolerated in living organisms and could be potentially useful in treating chronic inflammation and ROS related pathologies including cancer and neurodegenerative disease.384,385CeO2NPs are well known to act as antioxidants and to have redox-activity in tissue repair and regeneration. The relatively stable surface of peroxo/hydroperoxo species of CeO2NPs is used for ROS generation.386,387 CeO2NPs with high surface Ce4+/Ce3+ ratios function more efficiently as antioxidant enzyme mimics.388 The redox-activities of CeO2NPs (i.e., switching oxidation state of Ce3+ and Ce4+) make these NPs a potential candidate for biomedical applications. The direct scavenging of ˙OH by ceria NPs is presented in process 1 of Fig. 9(a), NO˙, OONO− chelating by CeO2NPs have also been investigated.389–391 CeO2NPs have also shown superoxide dismutase-like effects (process 3 and 5 in Fig. 9(a)), that are associated with surface concentrations of Ce3+ and Ce4+, pH, and chelating ligand concentrations.388,392 The inflammatory cell signalling response to CeO2NPs, and cell apoptosis upon ROS production are shown in process 4 and 6 in Fig. 9.268,393
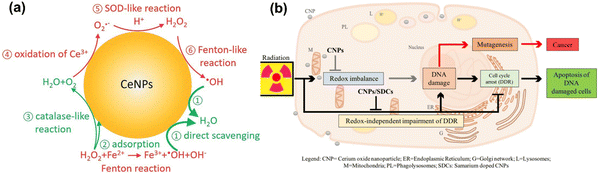 |
| Fig. 9 (a) Schematic illustration of Fenton reaction and reactive oxygen chemistry of ceria nanoparticles. Surface chemical reactions presented in red and green colors imply ROS generation and scavenging steps, respectively. This is a proposed model for ROS production and scavenging from ceria nanoparticles as redox-independent radio-sensitizing agents in HaCat keratinocytes cells. Reproduced with permission from Filippi et al.394 [Copyright 2019, Royal Society of Chemistry]. (b) Schematic diagram illustrate the administrative role of ceria nanoparticles (here CNPs) to promote in a redox-independent manner strengthening of the cell DNA damage response (DDR) after exposure to radiation, weakening X-ray-induced DNA lesions on one side, and strengthening the stringency of cell cycle checkpoints and forcing damaged cells to undergo apoptosis on the other, hence inhibiting radiation-induced mutagenesis. Reproduced with permission from Corsi et al.386 [Copyright 2018, Frontiers]. | |
Recently, Filippi et al. have demonstrated the antioxidant activity of CeO2NPs and cerium nanorods (CNRs) in scavenging hydroxyl radicals.394 They have shown that CeO2NPs and CeO2NRs exhibit stronger ROS scavenging activities than ˙OH generation in phosphate buffer saline (PBS) and surrogated lung fluid (SLF). Further, CeO2NRs have a larger surface area and higher defect density than CeO2NPs, resulting in greater ˙OH scavenging activity. Mahapatra et al. have suggested that the CeO2NPs with different directional shapes (aspect ratios) are internalized in different rates in human dental pulp stem cells.395 Nanoparticles with a smaller aspect ratio (CeO2NPs and CeO2NRs) are internalized faster into the cells and are more effective at suppressing ROS either intracellularly or extracellularly upon H2O2 treatment. Their findings suggest that special attention should be paid to the resulting particle geometry during synthesis of cerium oxide-based nanomaterials, depending on their use such as for ROS-scavenging to protect from the ROS-insult environment, stem cell protection, tissue engineering and regenerative medicine applications.
Cells internalizing CeO2NPs appear to respond more effectively to DNA damage via unrelated mechanisms that reduce DNA breaks, improving apoptotic outcomes.396 Moreover, CeO2NPs help cells to restore DNA integrity, the ability of which cancer cells lose during X-ray mediated mutagenesis by acting on the intimate pathways controlling survival of injured cancer cells. The radio-sensitization has no relationship with the redox-switching of CeO2 nanoparticles because it has not been affected by Sm-doping, a strategy that prevents a switch of Ce3+/Ce4+ and provides 3+ valence by providing an antioxidant action397,398 as shown in Fig. 9(b). The mechanisms of how CeO2NPs interact with cells have been studied at cellular and molecular levels by several groups. CeO2NPs have been suggested as cytoprotective antioxidants and free radical scavengers or oxidants but have also showed cytotoxicity. CeO2NPs delayed cellular damage with ROS scavenging properties, resulting in an increase of cellular resistance to an exogenous ROS stimulation in oxidative stress condition.399 On the contrary, the pro-oxidative effect of CeO2NPs induces oxidative stress leading to cell death upon the cellular internalization of CeO2NPs. Subsequently, ROS are generated from reduction of Ce(IV) to Ce(III), and the dual nature of CeO2NPs, as an antioxidant and a pro-oxidant, could be dependent on the shape, size, dose and exposure time of the nanoparticles.395,400–402 Recently, Pota et al. have synthesized the redox-active CeO2-based hybrid nanostructure via molecular combinations of organic and inorganic semiconductor for antibacterial and biomimetic radical homeostasis applications.403
3.3. Carbon-based nanomaterials
Carbon nanotubes (CNTs), fullerenes (C60), nanodiamonds, carbon quantum dots, graphene and its derivatives are all carbon-based nanomaterials (CBNs).25,404 CBNs have unique properties including a high electrical conductivity, high mechanical strength, thermal conductivity, tunable optical behaviour, and catalytic activities which have led to significant interest in diverse fields such as biomedicine (drug delivery, tissue engineering, and regenerative medicine), energy storage, electronics, and biosensing. CBNs have recently shown a huge impact in biomedical fields, particularly applied in therapeutic delivery and cell/tissue imaging in cancer treatment. In fact, the potential use of CBNs has been reported in tissue engineering, anticancer, and anti-inflammatory treatments with their effect mainly due to ROS generation in cancer. The ROS generation by CBNs causes lysosomal and DNA damage, mitochondrial dysfunction and eventually leads to cell death via either apoptosis or necrosis. Moreover, CBNs have been intensively studied in pulmonary macrophage activation and inflammation, and the mechanisms of their immune suppression continue to be investigated.405,406
3.3.1. Single-walled and multi-walled carbon nanotubes. Single-walled carbon nanotubes (SWCNTs) and multi-walled carbon nanotubes (MWCNTs) are the two types of carbon nanotube. The development of SWCNT-based cellular technologies has gained interest in nanomedicine for drug delivery, imaging, and immune response modulation.407 An additional advantage of SWCNTs is their ability to avoid interfering with normal cell process, while maintaining their inherent properties. Pristine SWCNTs have toxic and negative effects on cellular responses, but functionalized SWCNTs have shown less cytotoxicity, better dispersion in aqueous solutions and better delivery to the cells.408–410 In the last three decades, CBNs have been well established as a promising nanomaterial for technological and pharmaceutical applications. However, the long half-life of CBNs remains as a matter of concern in biological environments. Particularly for in vivo applications of CBNs, challenges remain in addressing their biodistribution that may affect their efficiency and safety. Michael et al. have developed a tumor-targeting SWCNT construct using covalent functionalization with multiple copies of tumor-specific antibodies, fluorescent probes, and radiometal-ion chelates.411 The construct was used in human lymphoma in vivo model, in vitro flow cytometry, and cell-based immunoreactivity experiments with respect to adequate controls because it was known to react with human cancer cells. They found that the SWCNTs were degraded slowly in phagosomes, although the underlying mechanisms of graphitic degradation remain unclear. The enzymatic degradation of CNTs was tested in the presence of horseradish peroxide which can oxidize SWCNTs in the presence of a low level of H2O2 at 4 °C in over 12 weeks of time at static conditions.412 The biodegradation of CNTs has been studied using several other peroxidase enzymes including myeloperoxidase (MPO),413 neutrophils and eosinophil peroxidase,414 and lactoperoxidase415 expressed by goblet cells in the epithelial layers of the respiratory tract. A mini review by Yang et al. has summarized studies on CNT biodegradation by macrophages and other factors that can influence CNTs biodegradation based on their individual characteristics and applications.416 The schematic illustration of biological pathways for CNT biodegradation and ROS generation is presented in Fig. 10(a). Once CNTs are internalized into macrophages, either NADPH oxidase activates and starts the formation of O2−˙ and then conversion of superoxide into H2O2 by SOD, or reacts with free radical nitric oxide to produce ONOO−. Hypochlorite is created when H2O2 and Cl− are combined by an enzyme like MPO. Conversely, if Fe3+ is present, ˙OH is created, which attacks unsaturated carbon bonds on the side walls of CNTs and causes holes to form in the graphitic structure, ultimately leading to the degradation of CNTs into carbon dioxide.417,418
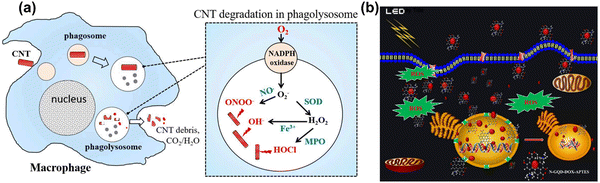 |
| Fig. 10 (a) A scheme illustration of CNTs degradation and generation of ROS in a macrophage cell. Reproduced with permission from Yang et al.416 [Copyright 2019, Frontiers]. (b) Light-mediated drug delivery and ROS production from GQD in breast cancer cell line MDA-MB-231 cells. Reproduced with permission from Ju et al.419 [Copyright 2019, Wiley]. | |
3.3.2. Carbon-based quantum dots. Carbon-based quantum dots (QDs) have also been explored for their antioxidant activity. Graphene quantum dots (GQDs) are well known for their electron donor and acceptor abilities and hence exhibit antioxidant activity and ROS generation under UV and visible light irradiation. The assembly of quantum dot-dye conjugates construct using peptide bridge can specifically design to recognize and interact with the breast cancer biomarkers.420 Christensen et al. have reported the dual nature of GQDs on the generation of singlet oxygen and ROS in an aqueous solution and radical either by chemical exposure using 2,2′-azobis (2-amidinopropane) dihydrochloride (AAPH) or by irradiation of visible light of 390–470 nm (peak 420 nm).421 This study also shows that GQDs inhibit the oxidation of radical probes by decomposition of AAPH, concluding that upon external irradiation GQDs can generate ROS acting as pro-oxidants, and also scavenge chemically generated radical like antioxidants. Interestingly, Ruiz et al. have reported that the antioxidant ability of GQDs can be tuned with different chemical compositions and sp2-hybridized carbon content.422 They used top-down and bottom-up methods for GQDs synthesis using three different precursors namely carbon black, glucose, and pyrene with various chemical compositions, electron densities, and sp2-hybridized carbon content. These results suggest that the GQDs with strong hydrogen donation potential and high contents of sp2-hydridized carbon domains are the most effective radical scavengers. Recently, Nilewski et al. have reported that coal-derived GQDs functionalized with poly(ethylene glycol) show antioxidants abilities in chemical, electrochemical, and in vitro biologically stimulating conditions.423 This study also showed that coal derived GQDs can quench superoxide and ˙OH, converting superoxide into oxygen, acting as SOD mimetics. Wang et al. have improved GQDs by optimizing the functional groups of oxygen.242 A study was performed to determine if surface oxygen functional groups had any relationship with antioxidant activity, researchers prepared GQDs with different total oxygen fractions and controlled oxygen concentrations. A GQD's antioxidant activity was affected by both its total oxygen level and types of oxygen groups. Furthermore, the transfer of hydrogen donor from the surface hydroxyl and carbonyl groups for the creation of adducts at the sp2 hybridized carbon atom site is the primary mechanism for the free radical scavenging ability of GQDs. It has been demonstrated that C–OH and C
O groups are more active than C–O–C groups for the scavenging of radical. However, different locations and chemical environments for each surface oxygen group may contribute to the scavenging actions of GQDs. Li et al. have reported electrochemical synthesis of phosphorus-doped GQDs and tested the particles for their free radical scavenging effects.424 They found that phosphorous doping on the GQDs played a critical role in scavenging DPPH radicals, as well as oxygen groups on the surface of GQDs. Carotenoids and other intriguing compounds with lengthy conjugated C
C chains have also been suggested as effective free radical scavengers.425 Similarly, other CBNs have been thoroughly investigated for their capacity to neutralize ROS and operate as free radical scavengers. For instance, fullerene derivatives exhibit high superoxide quenching activity, which is supported by a mechanism that combines electron transfer and adduct formation.426 Recently, CNT-tailored electrospun nanofibers with electrical conductivity and bi-modal nanotopography have been used for bone and neuronal tissue engineering applications.427,428 Fenogilo et al. found that MWCNTs in aqueous solution do not generate oxygen or carbon-centered radical in the presence of H2O2 and formate.429 On the contrary, purified MWCNTs are very effective scavengers for ˙OH and O2−˙, although the molecular mechanism is not fully understood. Galanio et al. have reported theoretical studies on the potential ability of SWCNTs as free radical scavengers using density functional theory calculations.430 Their findings suggest that once radicals are attached to a nanotube, additional reactions can be enhanced, indicating that SWCNTs can act as free radical sponges. In case of reaction with OH radicals, subsequent additions would lead to products in which the created OH groups are distributed in clusters rather than a homogeneous spread throughout the nanotube.
3.3.3. Graphene-based nanomaterials. Graphene-based (2D) nanomaterials such as graphene (G), reduced graphene oxide (rGO), and few-layer graphene (FLG) have been used as a new class of potential antioxidants that combine physical barrier function with an ultra-high surface area for free radical scavenging. Moreover, graphene-based nanomaterials have also been shown to have protective characteristics to many molecular targets from oxidation by these species, and to be highly effective as ˙OH scavengers. Qiu et al. have investigated the antioxidant chemistry of GO, rGO, and FLG focusing on their roles in oxygen protection technology.431 They found that the ˙OH scavenging activities were in the order FLG > rGO > GO, which is an inverse order of the total surface area of the corresponding graphene nanomaterials. Although FLG has a smaller surface area, its greater scavenging ability suggests that its main scavenging ability is due to the intact sp2-hybridized carbon domains on the basal surface, rather than hydroquinone or hydroxyl groups. In addition, GO is not a strong H-donor due to the non-phenolic nature of the hydroxyl groups on its surface, which reside at basal sp3-hybridized carbon atoms, and hence do not allow radical resonance stabilization following H-donation.Many other CBNs including fullerenes,432 CNTs, carbon nanodots and metal-doped carbon nanodots,433–435 GQDs,436 GO,437,438 rGO,439 FLG431 and metal functionalized GO16,165,440 have been investigated for ROS and radical scavenging effects. The scavenging abilities of CBNs are due to sp2-hybridized carbon sites, structural defects, surface electrophonic effects, H-donation from surface functional groups, and adduct formation. However, other possible factors including imperfect antioxidant mechanisms, a lack of an effective method for the regulation of antioxidants, and a low antioxidant activity need to be investigated.
ROS generation and their roles have been implicated in many disease animal models. Sharma et al. have performed a set of experiments to determine SWCNT toxicity in rat epithelial cells and measured ROS generation by change in fluorescence using dichloroflurescein.441 They found that the increased ROS production upon rat epithelial cell exposure to SWCNTs occurred in a dose and time dependent manner. Additionally, the decreased level of glutathione suggested a loss of cellular defense mechanisms against ROS generation in SWCNTs treated cells. The inhibition of mitochondrial function had no change on ROS levels, suggesting the role of SWCNTs in ROS production. Manna et al. have reported the effects of SWCNTs in human keratinocytes cells, looking at toxicity, oxidative stress and cell proliferation.442 They found that the SWCNTs increased oxidative stress in keratinocytes by activating NF-κB pathway in a dose dependent manner. The NF-κB signalling seems to be activated by stress-related kinases triggered by SWCNTs in the keratinocytes. Alarifi et al. have reported the underlying mechanism of the toxic effects and ROS generation of MWCNTs in mouse fibroblasts (L929).443 They found that the MWCNTs significantly increase ROS production, lipid peroxidation, superoxide dismutase levels, and decrease glutathione concentration. Rasras et al. have examined the effects of SWCNTs and MWCNTs in rat brains, in particular focusing on mitochondria. They measured ROS activity with altered levels of malondialdehyde, glutathione and mitochondrial membrane potential.444 They found that both SWCNTs and MWCNTs may damage brain tissue through mitochondria by increasing oxidative stress and activating apoptosis-related cell death pathways. According to Hou et al. the direct photoreactivity of pristine SWCNTs is usually low under sunlight, however indirect photoreactions that generate ˙OH may play a greater role in natural aquatic environments.445 Furthermore, the reactivity of SWCNTs to ˙OH depends on their chirality as well as the surfactant used. Singh et al. have demonstrated the influence of SWCNTs on multicellular chirality, a property that describes a state of directional cell migration. They also show that incubation of SWCNTs with mouse myoblasts (C2C12) and human umbilical vein endothelial cells (hUVECs) stimulated ROS production by intra and extracellular oxidative stress in dose and time dependent manner.446 They found that the SWCNTs-mediated oxidative stress leads to a loss of multicellular chirality.
Graphene itself is not considered a good candidate for biomedical applications due to its poor dispersibility in aqueous conditions such as water, phosphate buffer and culture media, however, chemically functionalized GO or rGO are good candidates for various biomedical applications.447–449 GO and rGO have widely been studied in cell biology due to their outstanding biocompatibility, large surface area, and simple modification of the surface.450,451 GO contains hydroxyl, carbonyl, epoxy, and carboxyl groups on the base plane and edges, which promote cell affinity and a high hydrophilicity. Due to the wrinkled nature of the carbon plane, GO may increase stem cell adherence and differentiation to the osteoblast lineage.452,453 Nanosized GO sheets could serve as nanocarriers of drugs451,454,455 and nucleic acids,456 for gene transfection457 into cells or animals458 for bioimaging,459 biosensing,460,461 and therapeutic purposes.462
Graphene-based materials can modulate the metabolic activities of macrophages by increasing ROS levels resulting in mitochondrial membrane damage and apoptotic cell death. It is complicated and challenging to study the stability and susceptibility to different reagents of graphene-based nanomaterials. In the study of Hsieh et al., the degradation rate constants of graphene-based nanomaterials have been calculated using dissolved organic carbon loss and steady-state concentrations of individual ROS as an indicator of their reactivity with OH, 1O2, and O2.463 Their findings suggest that ROS distribution and GO reactivity with ROS requires long-term exposure of GO and rGO. A detailed review by Niu et al. has summarized nanocarrier-based delivery vehicles to overcome the challenge of crossing the blood–brain barrier (BBB) to cure neurodegenerative diseases.464 Ju et al. have investigated ROS using graphene quantum dots (GQD) that function as a photosensitizer with various nitrogen atom dopant concentrations, thus achieving photodynamic therapy.419 They also found that amine functionalized doxorubicin (DOX) loading on GQDs can have two functions, first, as a drug delivery carrier for nuclear targeting with photosensitizer and second, as a strong ROS generator as shown in Fig. 10(b).
3.3.4. Silicon carbide nanomaterials. Silicon carbide (SiC) is a compound composed of silicon (Si) and carbon (C) atoms in a 1
:
1 ratio, and can be considered as carbon-based nanomaterials in the sense that it contains carbon as a significant component of its structure. In crystal lattice structure, each silicon atom is covalently bonded to a carbon atom.465 Recently, SiC is growing significant interests as a potential solution for enhanced energy storage and power density.466 Moreover, the unique physical and chemical characteristics of SiC such as high temperature resistance, strong mechanical strength, excellent resistance to wear and oxidation, and chemical stability compared to carbon-based materials endow SiC an excellent nanomaterials for renewable energy storage, and portable electron applications.467Silicon carbide is the second hardest-known material after diamond and is used in various industrial applications. SiC has potential applications in various fields but has been reported to have toxic effects on bacteria and mammalian cells.468,469 For example, Pourchez et al. investigated SiC nanoparticles for impact of physico-chemical features on pro-inflammatory and pro-oxidative effects on macrophage.470 They found that SiC nanoparticles generate oxidative stress in macrophages, leading to significantly enhanced and dose-dependent LDH release and pro-inflammatory response in the macrophage cell line. The cytotoxicity of SiC mostly depends on morphology of the SiC nanoparticles. Studies have shown that SiC nanostructures such as nanofibers and nanorods induce oxidative stress in Pseudomonas putida compared to micrometric SiC, likely by increasing the formation of highly reactive radicals and disrupting cell membrane integrity, leading to DNA damage.469 Similarly, research has revealed that SiC nanowires (SiCNWs) are more toxic to hMSCs than SiC nanoparticles (SiCNPs) at the same concentration. Moreover, SiCNWs negatively impact hMSCs’ cellular activities including adhesion, proliferation, migration ability, multipotency, phenotypes, and cytokine secretion. This adverse effect is likely due to an overproduction of pro-inflammatory cytokines MCP-1 and IL-8 and a deficiency in MMP-3 and C3. Furthermore, SiCNWs have a greater impact on MCP-1, IL-8, MMP-3, and C3 secretion than SiCNPs, leading to differences in cytotoxicity to hMSCs between the two.468 In summary, while SiC includes carbon as a key components, it is more accurately described as a ceramic or composite material rather than carbon-based materials.
3.4. Nanomaterials for drug delivery
RANs have also been developed for the delivery of molecules/drugs which can cause intracellular ROS generation after internalization by cells. Mesoporous silica nanoparticles (MSNs) are frequently used in drug delivery, imaging, and many biomedical applications due to their unique physical pore structure (pore size, shape, volume, and alignment), surface area, surface chemistry, biocompatibility and bio-stability.239 Their biomedical applications include targeted therapeutic delivery, biosensing, cell and tissue imaging, and photothermal or photodynamic therapy for cancer treatment. Both porous and non-porous silica nanoparticles can generate ROS in the form of ˙OH, O2−˙ and H2O2, causing an imbalance between oxidant and antioxidant states and creating intracellular oxidative stress.471 For example, Yi et al. have fabricated MSNs as redox-responsive short-chain gatekeeper nanoparticles for the controlled release of salicylic acid.472 Redox-responsive gatekeepers such as these MSNs are openable by glutathione, an endogenous reductant in cells, and thus do not require any external/exogenous agents. The versatility of MSN surface chemistry creates huge potential for the design of new multifunctional nanocarriers for more efficient delivery. An innovative redox-responsive delivery system of multifunctional nanoparticles capped with bovine serum albumin and hyaluronic acid has been developed by Chen et al. for cancer drug delivery and MRI imaging.473 An overview of the synthetic process for a multifunctional MSN for redox-responsive drug delivery and MR imaging can be found in Fig. 11. Zhao et al. have also modified MSNs with redox-responsive oligosaccharides for targeted drug delivery using disulfide bonds that are cleavable under high concentrations of glutathione (GSH).474 The covalent disulfide bond is relatively stable in the extracellular fluid and plasma, and is easily ruptured in intracellular fluid due to the increase in GSH concentration between the extracellular (2–20 μM), and intracellular (1–10 mM) fluids.475 Surface functionalized MSNs have been tested for GSH-responsive drug release in tumors. As shown in Fig. 11(b), oligomeric hyaluronic acid functionalized multifunctional MSNs (oHA-MSN) are internalized through endocytosis. The HA-mediated CD44 interaction and GSH-triggered disulfide cleavage led to drug release in the cell after cellular uptake. Singh et al. have developed cerium oxide capped MSN nanocarriers as a pH-responsive drug delivery system.25 In this system the cerium oxide nanoparticles are shown to facilitate the pro-oxidant properties of MSNs followed by increased ROS levels leading to the killing of cancer cells. Additionally, a DOX combination therapy with cerium oxide capped MSNs is more effective in ROS generation, demonstrating a cytotoxic synergism between the drug and nanoparticle in terms of ROS generation. The combined effect of the pH-triggered intracellular ROS therapy and chemotherapy caused by DOX-loaded cerium oxide capped MSNs is presented in Fig. 11(c). A proper range of ROS concentration seems to be critical in functioning as essential messengers to facilitate immune responses.476,477 ROS can be a signal for cellular danger in the immune system by the activation of dendritic cells (DCs),478,479 increasing the concentration of co-stimulating molecules like CD80 and CD86 to speed up the presentation of an antigen.480 Therefore, ROS generating nanoparticles might improve the immune response.481 For example, Lu et al. have found that mesoporous organosilica nanoparticles improve cancer immunotherapy by depleting glutathione.482 They used biodegradable glutathione-depleted dendritic mesoporous organosilica nanoparticles (GDMON) with a tetrasulfide-incorporated framework to deliver an antigen protein (ovalbumin) and a toll-like receptor 9 (TLR9) agonist into antigen-presenting cells (APCs). As a result of –S–S–/GSH redox chemistry, glutathione (GSH) levels are decreased intracellularly and ROS are generated, leading to the proliferation of cytotoxic T lymphocytes (CTLs) which inhibited tumor growth in B16- OVA cells, an aggressive melanoma model, described in Fig. 11(d). Overall, intracellularly generated ROS could be an oxidative stress and intracellular antioxidant system like GSH will be operated in nature.483 In cells, GSH is an endogenous antioxidant neutralizing excessive ROS, which can be cytotoxic.484 Farooq et al. have tested titania coated MSNPs with hydroxyl groups on the surface that can react with intracellular H2O2 producing ROS, possibly contributing to biocompatibility as well as therapeutic delivery. The MSNPs generate ROS much less than dense silica nanoparticles. In addition, MSNPs exhibit less toxicity than nonporous silica nanoparticles, whose surface modification has further improved the biocompatibility.
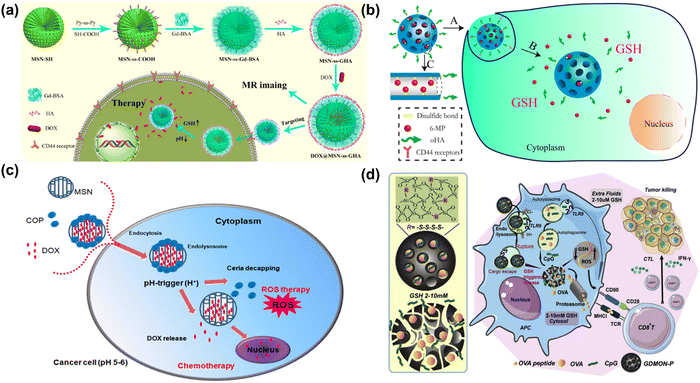 |
| Fig. 11 (a) Schematic diagram to illustrate the preparation method of multifunctional MSNs for redox-responsive theranostics (drug delivery and imaging). Reproduced with permission from Chen et al.473 [Copyright 2016, American Chemical Society]. (b) Schematic diagram presenting GSH-responsive drug release in the tumor cells from MSNs; (A) cellular internalization of oHA-modified MSNs via oHA mediated CD44 interaction, and (B) GSH-activated drug release into the cell, (C) Pore structure of CMS-SS-MP/oHA. Reproduced with permission from Zhao et al.474 [Copyright 2014, American Chemical Society]. (c) Schematic diagram illustrating the combined effect of intracellular ROS therapy and chemotherapy (doxorubicin), release from cerium oxide nanoparticles capped MSNs in cancer cells. Reproduced with permission from Singh et al.111 [Copyright 2019, American Chemical Society]. (d) Schematic illustration of GDMON-P + OVA + CpG enhanced cancer immunotherapy, and co-delivery of an antigen protein (ovalbumin) and CpG into antigen presenting cells (APCs) and inducing endosome escape. In the cytosol of APCs, GDMON-P diminish the intracellular glutathione (GSH) level through the –S–S–/GSH redox chemistry and up-regulating the intracellular ROS production and endowing specific cytotoxic T cell (CTL) proliferation and inducing cancer cell apoptosis. Reproduced with permission from Lu et al.482 [Copyright 2016, Elsevier]. | |
Previous research has attributed the increase in cytoplasmic and mitochondrial ROS production during silica nanoparticles (SiNPs) exposure solely to NADPH oxidase 2 (NOX2) pathways.485 However, research teams like Cui et al. and Joshi et al. reported ROS production upon exposure of cells such as macrophages and Cos7, to silica nanoparticles could also be independent from NOX2 pathways.485,486 Reported results suggest that silica nanoparticles are able to generate ROS independently from NOX2 once they are in the phagosomal system contributing to the increase in cytoplasmic ROS and subsequently downstream cytotoxic pathways such as over expression of COX2, enzyme linked to a variety of inflammatory pathways, or including phagosome leakage.485,486 The latter is hypothesized to happen trough Fenton reaction between the free ion left on the surface of the silica particles after production of hydrogen peroxide in the phagosomes, the resulting ˙OH radicals drive the damage of the phagosomal membrane via per-oxidation leaking ROS.486
In addition to mitochondrial and NOX2 mediated ROS production, other methods for ROS production induces by silica nanoparticles include peroxisomes and xanthine oxidase.487 Furthermore, Petrache Voicu et al. have shown that silica nanoparticles induced ROS production in MRC-5 cells is also enabled via severe down regulation of the tripeptides glutathione (GSH), antioxidant mechanism in cells.488 Moreover, the abundant hydroxyl groups on the surface of silica nanoparticles together with topographic irregularties enhances the production of hydroxyl radicals, which in turn can interact in the chemical reactions, such as production of superoxide that get dismutated by extracellular superoxide dismutases to produce hydrogen peroxide, or with membrane NADPH oxidase systems to produce superoxide anion even before entering into the cells.488
3.5. Biofouling and NPs aggregation effects
Surface biofouling is a common issue with the metallic nanoparticle's therapeutics, where non-specific biomolecules bind to and accumulate at the reactive nanoparticles surface, creating a protein corona.489 The formation of a protein corona has been shown to occur rapidly, to modulate nanoparticles toxicity, and to alter their uptake.490 Furthermore, quantification of the effect of a protein corona on the kinetics of redox-reactions at a NPs surface has not been carried out to our knowledge. It may be the case that this accumulation reduces the chance of direct interaction between electron donator and acceptor molecules and the NPs, thereby lowering its therapeutics efficacy. This magnitude will likely be particle and environment specific, with potential for some proteins to perhaps improve electron transfer from NPs surface if they undergo in redox-reactions themselves.491
The stability of a suspension of NPs, i.e. the likelihood of NPs aggregation, depends on its surface chemistry and the solvent its dispersed. The complex and dynamic physiological environments that a NP therapeutic may be exposed to during application, therefore present a great challenge in terms of retention activities. To overcome this, several passivation methods have been developed that preserve function and reduce NPs surface instabilities.492 Furthermore, various surface coatings approaches including polymers, lipids, and silica-based coatings have been developed to improve the stability, and functionality. Silica-based coatings introduced significant porosity at the surfaces with sustainable benefits for drug loading and controlled release,493 and lipid coating can be used to reduce cytotoxicity and improve cellular uptake due to the similarity to the cell membranes.494
3.6. Other types of nanomaterials
Up-conversion nanoparticles (UCNPs) are a distinct category of nanomaterials that are doped with rare earth metal atoms embedded in a crystalline structure. For example, lanthanide (Ln3+) can be used to dope nanocrystals. UCNPs are anti-Stokes type materials, which exhibit anti-Stokes optical properties. When these types of nanomaterials interact with infrared radiation (lower energy photons), they absorb and upconvert to emit in the visible light spectrum (higher energy photons) through a series of real as opposed to virtual levels, as in conventional two-photon dyes.495 The UCNPs can up-convert two or more lower energy photons into one high energy photon through either sequential excitation of one emitting atom or excitation actions of two different atoms and subsequent energy transfer between co-doped rare-earth atoms.496,497
The applications of UCNPs as delivery and bioimaging agents have been explored enormously, for example He et al. have developed up-conversion nano-onions (UNCOs) coated with polymer, which degrade sequentially in response to NIR stimulation in the extracellular environment.28,498 These UNCNOs have a core of UCNPs functionalized with the photosensitizer rose Bengal (RB), which is conjugated with poly ethylenimine 600 (PEI-600), followed by a ROS responsive middle layer of singlet oxygen (1O2) sensitive diselenide bonds (PEI-SeSe) connected to siRNA and cell penetrating peptide R8 modifications, and an outer coating layer of negatively charged hyaluronic acid (HA) represented as (UCNPs-PEIRB-PEI-SeSe-R8-HA) as presented in Fig. 12(a). Electrostatically adsorbed siRNA molecules on the middle PEI-SeSe layer were released when the UCNP core was irradiated with light between 525 and 540 nm to activate the RB in PEI-RB's inner layer, boosting ROS production and fully decomposing the PEI-SeSe bonds as shown in Fig. 12(b). The significance of the HA coating is to prolong the circulation of the nanoparticles in the bloodstream, by preventing NP degradation during delivery process and to control UCNO transporting to target special tumor cells via cell membrane receptor CD44.499 The subsequent stripping of UCNO coating upon internalization into the cells improved siRNA delivery without leaking, thus it can be an efficient strategy for NIR-assisted gene therapy.
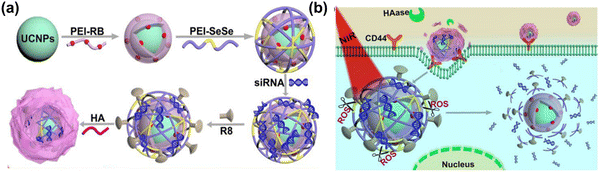 |
| Fig. 12 (a) Schematic illustration of step-by-step preparation and surface modification of up-conversion nanoparticles (UCNP-PEI-RB-PEI-SeSe/siRNA-R8-HA) for drug delivery and imaging applications, and (b) corresponding sequential responsive disintegration of UCNOs and NIR boosted intracellular siRNA release and reactive oxygen species therapy. Reproduced with permission from He et al.498 [Copyright 2019, Elsevier]. | |
4. Biomedical applications of RANs
4.1. Cancer
Reactive oxygen species are natural byproducts of various cellular processes including metabolism, proliferation, and differentiation. An excessive amount of ROS can damage cells by random interaction with proteins, lipids, and DNA, ultimately leading to cell death.35,500 ROS are generated as an oxidative phosphorylation byproduct in mitochondria including superoxide, hydrogen peroxide (H2O2) and hydroxyl (OH−) radicals.28,35 ROS regulation is important within the cellular microenvironment and is mostly controlled by ROS-scavenging mechanisms such as superoxide dismutases (SOD1, SOD2, SOD3), peroxiredoxins, glutaredoxins, thioredoxins, glutathione peroxidase, and catalases.501,502 However, in the event where these unstable radicals are uncontrolled due to a reduction of intracellular ROS-scavenging, oxidative stress can result.502 Oxidative stress conditions are harmful for normal cells, and a chronic over production of ROS can induce normal cells to transform into tumor cells through genetic alterations, thus reprogramming cancer cell metabolism.110
Among the pathophysiological processes that ROS are involved in, such as genomic instability, inflammation, and metabolic reprogramming, tumorigenesis is of particular interest.35 Disruption of ROS-scavenging-enzyme production can trigger several cancers signalling cascades, including cancer angiogenesis and metastasis as presented in Fig. 13. All these cellular activities mentioned above are highly dependent on the levels of ROS, which promote cell proliferation and survival at low levels, induce cell cycle arrest leading to cellular differentiation at intermediate levels and cause oxidative damage and DNA mutations at high levels potentially leading to cancer.501
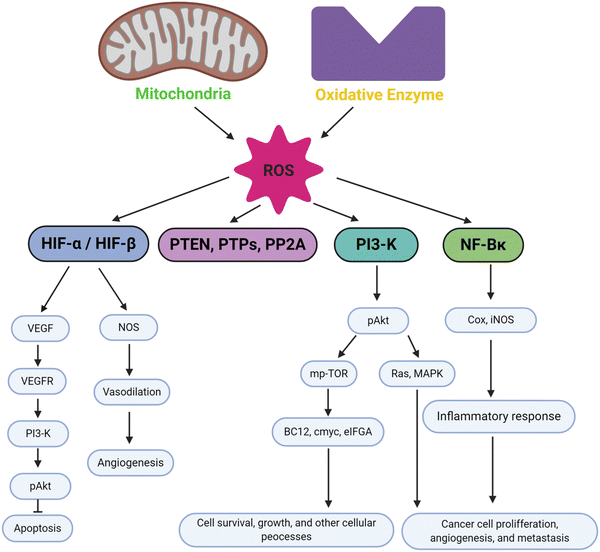 |
| Fig. 13 The biological significance of ROS in inducing cell signalling pathways in cancer for apoptosis, angiogenesis, cell survival, inflammatory response, and cancer proliferation and metastasis. Figure created by authors using BioRender.com. | |
ROS generation mainly occurs in mitochondria within the electron transport chain (ETC) in which one-electron reduction of oxygen happens at several major sites such as complexes I, II and III.35,501 Complexes I and II produces O2˙ in the mitochondrial matrix, and superoxide dismutase protein 2 (SOD2) will subsequently convert O2˙ into H2O2 though complex III when intermembrane O2˙ is transferred to the outer mitochondrial membranes and cytosol. Subsequently, O2˙ is converted into H2O2 by superoxide dismutase protein 1 (SOD1). Another ROS production pathway utilizes nicotinamide adenine dinucleotide phosphate (NADPH) oxidases (NOX) located in the cell membrane, which activate the conversion of oxygen to O2˙ via electron transfer across biological membranes, which will then be further reduced by superoxide dismutases to produce H2O2.35
Over the last few decades, surface functionalized metal nanoparticles, lipids particles, liposomes particles, quantum dots, and dendrimers have been extensively applied for anticancer drug delivery and imaging applications.503 The oxidative nature of ROS generated by NPs is very useful in anticancer therapy to suppress and eliminate cancerous cells. The activation of ROS is highly dependent on the redox-related application, such as photodynamic therapy (PDT), chemodynamic therapy (CDT), radiation therapy (RT), sonodynamic therapy (SDT), controlled drug release (CDR) etc., as well as the functionalities of the nanoparticles. Meanwhile nanoparticles containing prooxidant can be internalized into cancer cells followed by ROS generation within mitochondria with subsequent activation of tumor necrotic factor and other transcription factors, which finally lead to adenosine triphosphate depletion and cell necrosis.503 Alternatively, cancer cells can be killed by cell cycle arrest via disruptions of the mitochondrial membrane, which will further increase ROS levels, exceeding body defense system, and activate effector caspases.504,505
Chen et al. have reported the mechanism of plasmon enhanced PDT based on photosensitizers (PS) that generate ROS to enhance cancer therapeutic efficiency. The PS used in the study are upconversion nanoparticles conjugated with gold nanorods that convert near-infrared (NIR) light to visible light and then excite surface plasmon resonance (SPR) locally to generate cytotoxic ROS.506 During this process, apoptotic cell death via caspases-3 activation occurs followed by the release of cytochrome c from mitochondria upon ROS production as shown in Fig. 14(a). A similar study performed by Sonkusre et al.,503 involved selenium induced necroptosis reported in PC-3 cells, where the nanoparticle activates TNF and the transcription factor IRF1, leading to ROS-mediated necroptosis through RIP1 protein as illustrated in Fig. 14(b). Selenium nanoparticles (SeNPs) have been widely studied as they show strong anticancer activity and excellent biocompatibility in a physiological environment.503 Several forms of SeNP such as chemically synthesized SeNP, sol–gel synthesized SeNPs, and co-precipitated SeNPs have been reported.504 It has been demonstrated that chemically functionalized SeNPs inhibit cell growth by suppressing the transcription and translation of androgen receptors, leading to a loss of androgen receptors via phosphorylation, ubiquitination and Akt/Mdm2 degradation pathways.507,508 Meanwhile transferrin-conjugated SeNP increases intracellular ROS and activates MAPKs pathways inducing p52-mediated apoptosis. Glucose incorporated into SeNPs is also known to induce cell apoptosis.
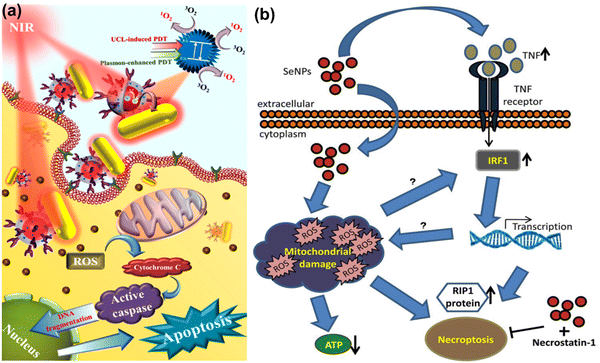 |
| Fig. 14 (a) Cell apoptosis pathway of plasmon-enhanced PDT treatment using upconversion nanoparticles conjugated with gold nanorods. Reproduced with permission from Chen et al.506 [Copyright 2016, American Chemical Society] (b) Selenium nanoparticles induced ROS-mediated necroptosis in PC-3 cells. Reproduced with permission from Sonkusre et al.503 [Copyright 2017, Springer]. | |
Recently, Zhuang et al. have reported that SeNPs enhanced the permeability and retention (EPR) of NP in the cancer area, thus inhibiting tumor growth more effectively. In addition, SeNPs accumulate within the cancer causing a drastic increase of ROS and oxidative stress, thus activating cell-death pathways.509 It is of interest that SeNPs coated with human serum albumin (HSA) show enhanced mitochondrial targeting compared to unmodified SeNPs. Alternatively, Chan et al. have investigated the potential combination of SeNPs seeded with iodine-125 (125I) for chemo-radiotherapy application to be more effective and safer to target cancer.510 Sensitizer based SeNPs have shown Auger-electron effect and Compton effect by generating a high concentration of intracellular ROS with only low-dosages for long-term activation, regulating p53-mediated DNA damage apoptotic signalling pathways and MAPKs phosphorylation to prevent cancer cell growth.510
4.2. Neurodegenerative diseases
Neurodegenerative diseases are a heterogeneous group of disorders including, Parkinson disease, Huntington diseases (HD), Alzheimer's disease (AD), epilepsy, multiple sclerosis (MS), amyotrophic lateral sclerosis (ALS), and strokes,511–514 defined by a decrease in the number of neurons and glial activation.515,516 Oxidative stress has been considered as a central and crucial element in the onset of these neurodegenerative conditions. Some important and comprehensive reviews have summarized ROS in neurodegenerative diseases in detail.514,517–519 The brain is far more vulnerable to excessively generated ROS than other tissues because of its high oxygen requirement and peroxidation vulnerability.
The most common type of senile dementia is Alzheimer's disease (AD), which is characterized by the presence of intracellular tangles of beta amyloid (Aβ) peptides and extracellular amyloid plaques made of hyperphosphorylated tau proteins.520,521 Numerous studies have reported the relationship between ROS and amyloid plaques. High levels of ROS can induce an inflammatory response, hyperphosphorylation of tau and neural dysfunction. Thus, it has been a long therapeutic strategy for AD to find drugs to inhibit the formation of plaques and tangles and to reduce the inflammation response. In this context, Yin et al. have created sialic acid-modified selenium nanoparticles incorporating a highly effective blood–brain barrier-penetrating peptide known as B6 (referred to as B6-SA-SeNPs). These nanoparticles serve as a synthetic selenoprotein analog, designed for Alzheimer's disease therapy.522 The results of their in vitro experiments suggest that B6-SA-SeNPs exhibit effectiveness not only in preventing the aggregation and promoting the disaggregation of Aβ, but also have the capacity to shield PC12 cells from Aβ-induced damage. Moreover, they claimed the developed nanoparticles cross the blood–brain-barrier in an in vitro system of PC12 cells. In order to prevent metal-induced Aβ aggregation, Yang et al. developed modified selenium/ruthenium nanoparticles (Se/Ru NPs) containing L-cysteine.523 Se/Ru NPs suppress a Zn2+ – amyloid mediated ROS generation process, thus reducing neurotoxicity in PC12 cells. A significant reduction in intracellular peptide aggregates was observed when spherical NPs with varying surface charges were used. NPs containing Ru also inhibit the random coiling or sheeting of Aβ that disrupts the alpha helical structure of amyloid plaques. Additionally, Se/RuNPs decrease Aβ40 fibrillization intracellularly, independent from lysosomal pathways. The release of borneol (Bor), which occurs when mesoporous nanoselenium (MNSe) reacts with blood or intracellular esterase, enables the nanosystem to cross the BBB.524
At the beginning stages of AD, the buildup of Aβ causes a surge in oxidative stress, which in turn causes mitochondrial malfunction and energy failure.517,525 A decline in dopaminergic input into the brain's nigrostriatal dopaminergic pathways and a specific neuronal loss of dopaminergic neurons in the substantia nigra pars compacta (SNc) are characteristics of the neurodegenerative disorder Parkinson's disease (PD).526–528 A genetic mutation of the Huntingtin (HTT) gene on chromosome 4 (4p63) results in HD, which is characterized by progressive loss of function in the brain and muscle, loss of neurons, uncontrollable movements, loss of intellectual ability, and emotional disorders.529 These neurogenerative disorders share common pathological mechanisms including protein aggregation, oxidative damage, and mitochondrial dysfunction. Many of the genes involved in these neurodegenerative diseases are associated with mitochondrial function.530 Therefore, it is vital to understand the underlying mechanism of mitochondrial dysfunctions when proposing RAN treatments for NDs. In addition to mitochondrial dysfunction, other cellular processes have recently been investigated for ROS generation. Activation of the peroxisome and endoplasmic reticulum, neuroinflammation, genetic mutation, and antioxidant depletion can induce ROS formation in the cell with subsequent loss of neurons by lipid peroxidation, and DNA or protein oxidation.531
Many studies have shown the roles of ROS in the development of neurodegenerative disorders.530–532 Although ROS might not be the main key factor in all events of progression of the disease developments, ROS generation in oxidative stress conditions could be a common boosting influence on cell damage. Considering that many good antioxidant candidates cannot cross the BBB, nanoparticles with antioxidant properties would be a good option for antioxidant delivery.533 Recently cerium oxide nanoparticles have been intensively studied in antioxidant delivery applications. A systematic representation of CeO2NPs and their inherent antioxidant characteristics for use as efficient neurodegeneration therapies is presented in Fig. 15(a). ROS scavenging from the injured area of the brain is critically aided by the low reduction potential of CeO2NPs and the coexistence of mixed valence states. Moreover, CeO2NPs can cross the BBB due to their nanoscale size. Considering the important role of oxidative stress, regulation of ROS concentrations is a promising treatment mechanism for the early stages of neurodegenerative disease. In this respect, numerous compounds that have antioxidant properties such as GSH, vitamin C, vitamin E and coenzyme Q10 have been studied for their potential to constrict neurodegenerative side effects in concert with NPs or using various delivery vehicles.532
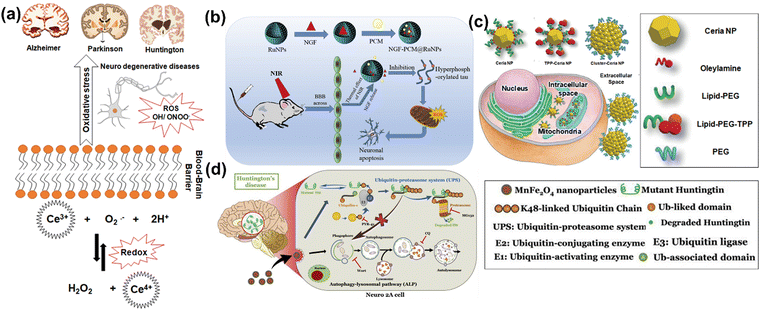 |
| Fig. 15 (a) Relationship between oxidative stress and neurodegenerative diseases; illustrating NP effect (cerium chosen randomly to illustrate oxidation reduction reactions). Reproduced with permission from Naz et al.534 [Copyright 2017, Future Medicine LTD]. (b) Schematic illustration of synthesis of RuNPs, their loading with nerve growth factor (NGF) and further phase change material (PCM) to create (NGF@PCM-Ru) NPs for drug delivery through the BBB under NIR-radiation in vivo and projection of thermos-responsive RuNPs to AD mice model, which subsequently down-regulated the ROS production and mitigation of neuronal damage by inhibiting tau hyperphosphorylation. Reproduced with permission from Zhou et al.,535 [Copyright 2020, Elsevier]. (c) Three different functionalized ceria nanoparticles and their cellular localization-dependent ROS scavenging in Parkinson's disease. Reproduced with permission from Kwon et al.,536 [Copyright 2018, Wiley]. (d) Schematic illustration of MnFe2O4 nanoparticles cellular internalization and accelerated clearance of mutant huntingtin (Htt) selectively through ubiquitin-proteasome (UPS) system in Huntington's disease. The rapid clearance of mutant Htt using MnFe2O4NPs via UPS rather than autophagy-lysosomal pathway (ALP). Among the enhanced degradation of Htt by UPS, K48-linked polyubiquitin chains target substrates to the proteasome and Ubiquilin-1 is the chosen ubiquitin receptor for mediating the cargo delivery. Reproduced with permission from Zhang et al.537 [Copyright 2019, Elsevier]. | |
Other RANs have also been investigated for neurodegenerative disease treatment. For example, Zhou et al. created a thermo-responsive hollow ruthenium flower-like system to maintain the release of nerve growth factor (NGF) and prevent tau hyperphosphorylation and neuronal death, offering a potential therapeutic for AD treatment.535 The thermally controlled drug delivery system of NGF-PCM@Ru NPs not only prolonged NGF circulation time but also penetrated the BBB and inhibited tau-related AD pathogenesis via NIR irradiation as presented in Fig. 15(b). In another study, Kwon et al. developed three forms of functionalized ceria NPs for ROS scavenging in Parkinson's disease, which inhibited microglial activation, lipid peroxidation, and restored tyrosine hydroxylase expression.536 The three differently functionalized NPs, also varying in size, were localized to different subcellular parts when delivered to the cell. ROS can be detected in mitochondria, intracellularly, and extracellularly as presented in Fig. 15(c), all these ROS play a distinctive role in pathological conditions. This study represents the first biomedical application of NPs for clearing reactive oxygen by-products in Parkinson's disease demonstrating their potential as new therapeutic targets. In the future, this concept could be implemented in various neuronal disorders associated with ROS including cancer, cardiovascular diseases, and sepsis. Zhang et al. have developed biocompatible MnFe2O4 NPs and applied them to chelate the 74 glutamine repeats (Htt(Q74)) of mutant huntingtin protein (Htt), the key disease factor in Huntington disease.537 Instead of triggering the autophagy pathway, Zhang's group found that MnFe2O4NPs facilitated Htt(Q74) degradation through the ubiquitin-proteasome system (UPS). In contrast to p62/SQSTM1, MnFe2O4NPs improved the ubiquitination of GFP-Htt(Q74) associated with the Lys 48 (K48) ubiquitin chain through the ubiquitin receptor. Further, they evaluated the potential efficacy of MnFe2O4NPs in Htt protein clearance and explored the underlying mechanism for protein degradation using Neuro 2A, a neural cell line stably expressing GFP-tagged mutant Htt aggregates with 74 polyQ tracts (mHtt-GFP-Q74) Fig. 15(d). This suggests that MnFe2O4NPs are good candidate nanoparticles in nanomedicine therapy for HD.
Interestingly, exosomes, extracellular vesicles (∼30–120 nm) that are secreted by cells, have also been used for drug delivery as they can also cross the BBB.538 It has been discovered that exosomes significantly contribute to the pathways leading to dementia. For instance, they contribute to the buildup of Aβ and the development of amyloid plaques in the brains of AD patients. Furthermore, exosomes transfer pathological protein aggregates containing α-synuclein to the CNS, contributing to PD.539 The biggest hurdle for RAN-based AD treatment is the potential side-effects arising from their short and long-term use. The gradual accumulation of NPs may be harmful with serious side effects in the body; therefore, it will be necessary to optimize the conditions of their use for therapy.
A recent study highlights the potential of graphene-based acid quantum dots (GAQDs) as a new therapeutic tool in treating neurodegenerative diseases. GAQDs have shown effectively reduce ROS by 50% at a concentration of 100 μg mL−1 when exposed to a free radical generator. Moreover, GAQDs demonstrated a 70% suppression of hen egg-white lysozyme fibril production at a 5 mg mL−1 concentration. In experiments using human neuroblastoma-derived SHSY-5Y cells, GAQDs were found to be biocompatible and capable of protecting cells from rotenone-induced apoptosis.540
RANs-based nanoparticles coated with biological membranes combine synthetic and biological elements were developed to improve targeting and therapeutic effectiveness. For example, Lin et al. developed drug-loaded hybrid cell-membrane liposomes that allow for multi-drug therapy and precise targeting of inflammatory brain lesion.541 This hybridization technique involved loading of two small molecule medications (rapamycin and TPPU) and combining a platelet membrane with a membrane expressing a high level of CCR2. In vitro, cell death was prevented by the autophagy enhancer rapamycin and the soluble epoxide hydrolase (sEH) inhibitor TPPU, which also improved cognitive function in AD model mice, reduced amyloid plaque levels in their brains, and decreased neuroinflammation.
L-Molybdenum disulfide quantum dots (L-MoS2 QDs), along with a carrier material and a surface coating, were utilized as the main component to address neuronal loss in AD. The L-MoS2 QDs were more effective in inducing neural stem cells (NSCs) neuronal development when exposed to CPL NIR radiation. Moreover, when injected in vivo into the tail vein of AD mice, the L-MoS2 QDs had complementary effects such as the photothermal clearance of Aβ plaques, the production of H2, which eliminated ROS, mitochondrial protection, and an overall improvement of the brain microenvironment.542
Combining ROS scavengers with nanomaterials exhibiting photothermal properties has emerged as a recent treatment for Alzheimer's disease. Song et al. developed Prussian blue nanomaterials (PBK NPs) with outstanding antioxidant and photothermal properties. The PBK NPs displayed activities similar to multiple antioxidant enzymes, such as peroxidase, superoxide dismutase, and catalase, which can help eliminate excessive ROS and alleviate oxidative stress. When subjected to near-infrared irradiation, the PBK NPs generated localized heat, effectively breaking down Aβ fibrils in vitro. Furthermore, modifying the PBK NPs with CKLVFFAED peptide enhanced their ability to penetrate the blood–brain barrier and target Aβ. Results from in vivo studies demonstrated that the PBK NPs disintegrated Aβ plaques and reduced neuroinflammation in an AD mouse model.543 A systematic summary of therapeutic roles of RANs in various diseases are presented (Table 3).
Table 3 Summary of the latest publications based on RANs nanoparticles and their therapeutic application using ROS in neurodegenerative diseases
ROS active NMs |
Application methods/surface modifications |
Brief outcomes in biomedical applications |
Ref. |
CeO2NPs |
Reverse micelle methods |
• CeO2NPs reduced neuronal apoptosis by altering BDNF signalling, and decreased Aβ-aggregation in Alzheimer's disease. |
534 |
• CeO2NPs also displayed a successful reduction of in manganese induced neuronal death in PD. |
CeO2NPs |
Yeast model of Parkinson's’ Disease |
• The ideal amount of CeO2NPs has been shown to significantly diminish the harm caused by α-synuclein-related toxicity, primarily stemming from the buildup of α-synuclein in the cytoplasm, which leads to the repositioning of α-synuclein to the plasma membrane upon the application of NPs. |
544 |
• CeO2NPs have demonstrated their ability to mitigate mitochondrial dysfunction induced by α-synuclein and reduce the levels of reactive oxygen species (ROS) in yeast cells. |
• Through the adsorption of α-synuclein onto their surface, CeO2NPs effectively function as a stable inhibitor of α-synuclein's harmful effects rather than a radical scavenger suggested that CeO2NPs may interact co-ordinately with α-syn in vivo. |
CeO2NPs |
Citrate–EDTA stabilizations to CeO2NPs Murine model of ALS (SOD1G93A transgenic mice) |
• The study found that administering 20 mg kg−1 of CeO2NPs intravenously twice per week increased the lifespan of SOD1G93A transgenic mice, a model for ALS. |
545 |
• Even when treatment was initiated at the onset of muscle weakness, the mice survived longer. |
• Both male and female mice benefited equally from CeO2NPs treatment suggests that CeO2NPs act as redox-agents that lower ROS levels, making them a promising therapeutic option. |
Se-incorporated clioquinol compounds |
Neuroblastoma |
• Showed protective properties against the oxidation and aggregation of Aβ induced by Cu(II). |
251 |
SeNPs |
Transgenic Huntington Disease (HD) models of Caenorhabditis elegans |
• Nano-Se has been found to reduce neuronal death in a dose-dependent manner and provide protection against neuronal dysfunction. |
546 |
• In a HD model, treatment with Nano-Se resulted in decreased levels of ROS and HTT aggregation, indicating that Nano-Se functions as an antioxidant by regulating the expression of the histone deacetylase family and reducing polyQ aggregation. |
OX26-PEG-Se NPs |
To targeting the transferrin receptor. – in vivo |
• Se nanoparticles coated with a polyethylene glycol (PEG) layer and treated with a monoclonal antibody (OX26) |
257 |
• The injection of OX26-PEG-SeNPs into the peritoneal cavity reduced brain edema in Wistar rats with ischemic cerebral stroke. |
Fe3O4NPs |
Coated with (NIPAm-AA) and modified oleic acid/chronic Parkinson disease model |
• Reduction of apoptosis and α-syn toxicity and showed neurorepair effect in vitro and in vivo models of PD. |
340 and 464 |
AgNPs |
NP–protein complex, protein-corona formation by incubating human plasma |
• Exposure to AgNPs may activate pathways associated with neurotoxicity and neurodegeneration. |
547 |
Triphenylphosphonium-conjugated ceria (TPP-ceria) |
Hydrolytic sol–gel, metal chelators or DSPE-PEG coatings |
The results indicated that these nanoparticles successfully decreased the buildup of mitochondrial ROS caused by Aβ in the U373 astrocyte cell line, implying that TPP-ceria NPs could potentially alleviate brain inflammation by lowering mitochondrial ROS levels. |
548 |
Gd3N@C80 encapsulated NP |
Colloidal stability in a polymer solution |
• The nano-contrast agent for MRI is designed to possess colloidal stability, ROS-scavenging ability, and long-term circulation ability, making it an excellent choice for clinical diagnosis. |
549 |
MSNP |
Lipoprotein receptor (LDLR) (conjugating and PLA coating) |
• The use of LDLR peptides significantly improved the transcytosis of MSNPs and facilitated their passage through the BBB. |
550 |
• Additionally, PLA-coated MSNPs were able to release resveratrol (antioxidant) in response to artificially induced superoxide released by activated microglia. This released resveratrol effectively reduced oxidative stress. |
PEG-b-poly[4-(2,2,6,6 tetra-methylpiperidine-1-oxyl)aminomethylstyrene] (PEG-b-PMNT) |
2,2,6,6-Tetramethylpiperidine-1-oxyl (TEMPO) added as side chain-oral intake to Alzheimer disease (AD) mice model |
• When mice orally consumed these particles, they not only prevented the accumulation of Aβ, but also significantly improved both spatial and non-spatial memory. |
551 |
• Additionally, the particles enhanced neuronal densities in the cortex and hippocampus by reducing the activity of glutathione peroxidase and superoxide dismutase. Furthermore, the levels of Aβ (1–40), Aβ (1–42) and gamma (γ)-secretase were reduced, ultimately leading to a reduction in Aβ plaque. |
RuNPs |
NGF loading, phase change material (PCM) presenting to AD mice |
• The nanocomposites showed excellent choice for biosafety in AD treatment due to its good biocompatibility and high stability. |
535 |
• With the aid of RuNPs' photothermal activity, cross-BBB movement and brain penetration was improved under NIR irradiation, leading to enhanced circulation efficiency in vivo. |
• The researcher found that modified RuNPs had the ability to effectively inhibit hyperphosphorylation of tau, reduced oxidative stress, and reduced tau aggregation, restore nerve damage, and alleviate cognitive impairment in AD mice. |
AuNPs |
1-Methyl-4-phenyl-1,2,3,6-tetrahydropyridine (MPTP) induced rat models with PD. |
• AuNPs can effectively reduce motor disorders, oxidative stress, and inflammatory cytokines, as well as inhibit TLR/NF-κB signalling in rats with PD. |
552 |
• Additionally, the authors observed that MPTP administration in the brain increased ROS generation, while treatment with AuNPs was able to scavenge ROS in a dose-dependent manners. |
4.3. Infection and inflammation
Acute or chronic pathogenic infections by bacteria, viruses and parasites are a primary cause of human morbidity and mortality. During infection, overproduction of ROS can occur. This occurs most notably in infections caused by blood-borne viruses such as human immunodeficiency virus (HIV), hepatitis (B, C and D), influenza A, respiratory syncytial virus, Epstein-Barr virus and others.553 In liver diseases, production of ROS associated with transcriptional activation of some cytokines and growth factors leads to hepatic damage. Additionally, viral infections downregulate glutathione (GSH) in liver cells, leading to the oxidation of DNA, lipids and proteins, and the cascade of these events contributes to the development of liver cirrhosis and hepatocellular carcinoma.554 There are antioxidant strategies to react with ROS produced from the electron transport chain, including the production of non-enzymatic ROS scavengers [GSH and NAD(P)H] and antioxidant enzymes [superoxide dismutase, catalase and family members of peroxidase].555 However, temporary imbalance between antioxidants and ROS can alter cellular signalling pathways, which could be reversed by nanoparticles encapsulated with antioxidants to treat liver diseases.
The versatile properties of nanoceria make it an ideal antibacterial solution against both Gram-negative and Gram-positive bacteria by generating ROS. It acts as an antioxidant in healthy cells, protecting them under normal physiological pH, while also acting as a pro-oxidant in cancer cells under low pH environments, which may lead to the death of cancer cells. Furthermore, nanoceria has been successfully utilized as a carrier for targeted drug and gene delivery in both in vitro and in vivo models. Besides, nanoceria is known to be a good candidate as an anti-diabetic, anti-inflammatory agent with protective effects against diabetes pathophysiology associated with a decrease in ROS concentration in diabetic patients. Nanoceria is also actively studied in the field of tissue engineering.556,557
ROS have two faces in normal cell responses, such that they are important in host defense, but paradoxically inhibit inflammation and immune response.558,559 In non-infectious hyper-inflammatory conditions such as chronic granulomatous disease (CGD), there are both high and low levels of ROS in the inflammatory responses.558 The lack of ROS generation by the NOX2 complex is known to be associated with a high incidence of autoimmune disease in CGD patients. A deficiency in NOX2 can lead to hyperinflammation and increased immune activation, which may have some benefits. In CGD mice, heightened inflammation has been shown to protect against pulmonary infection caused by influenza.404,560 The association between NOX2-dependent ROS generation deficiency and increased inflammation is unexpected and requires further investigation.
Oxidative stress arises when the production of reactive ROS surpasses the capacity of antioxidant defense mechanisms, potentially resulting in the development of various diseases. Hydrogen peroxide (H2O2) is a widely seen and long-lasting form of ROS that has been linked to various physiological processes including inflammation, cellular malfunction, and apoptosis. These factors ultimately have a role in the development of tissue and organ damage.561 Cellular DNA damage arises from a combination of intrinsic and extrinsic causes, encompassing ionizing radiation, chemical intake, light exposure, and radical generated during oxygen metabolism. The presence of these external stimuli leads to the production of radical, which in turn initiates a pathway that causes damage to DNA, resulting in a total of 465 instances of such damage.562 The phenomenon of DNA damage, commonly referred to as genotoxicity, is of great importance within the field of nanomaterials. Although the development of non-cytotoxic therapies is challenging, DNA damage offers a promising avenue for the creation of anti-cancer medications.563 ROS generation has been recognized as a crucial determinant in the modulation of the cellular response to chemotherapy or radiotherapy, exerting its influence on the downstream signalling pathways involved in cell survival and death.
The role of ROS in the DNA damage response is complicated and can be revealing in different ways. It is of utmost significance to distinguish between the occurrence of DNA damage resulting from oxidative stress and the following initiation of DNA damage repair (DDR). Additionally, it is crucial to consider the influence of ROS on the regulation of DDR components, encompassing both signalling and effectors. Multiple studies have indicated that the dysregulation of ROS plays a substantial role in the development of cancer, as well as in the resistance to chemotherapy and radiation therapy.564 The presence of active nanoparticles in the presence of ROS can result in the induction of DNA damage by the production of a hydroxyl radical (HO˙). This radical interacts with DNA molecules, leading to the formation of a specific DNA lesion called 8-hydroxy-2′-deoxyguanosine (8-OHdG). Ultimately, this process contributes to the occurrence of DNA damage.565 The excessive generation of radical can induce oxidative stress and inflammation, hence contributing to the development of numerous diseases.566 Certain nanoparticles, namely copper, copper oxide, iron, silver, TiO2, and nickel, exhibit pronounced toxicity. Even at low concentrations, exposure to these NPs can induce DNA damage and elicit the expression of inflammatory markers. These particles induce cellular apoptosis and inflammation through the generation of ROS, primarily targeting mitochondria and pro-oxidant enzymes.567 The utilization of various nanomaterials has been found to result in toxicity associated with the formation of ROS in multiple biological systems, such as human erythrocytes, skin fibroblasts, and various tumor cells.568 The mitochondria play a pivotal role in the production of ROS that are linked to nanoparticles. Previous studies have demonstrated that NPs have the capability to stimulate NADPH-associated enzymes, resulting in the depolarization of the mitochondrial membrane and the disruption of the electron transport chain.208,569 This inhibition of a key metabolic process would increase the quantity of ROS in cells by allowing electron transfer from respiratory carriers to oxygen.570 The mechanism implicated in NP-induced ROS production and factors affecting the ROS generation in cells are presented in Fig. 16.
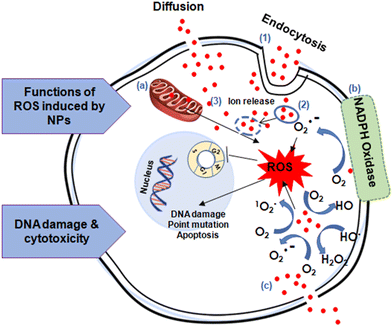 |
| Fig. 16 Schematic illustration of the cellular mechanisms associated with NP-induced ROS production. The nanoparticles can be internalized into the cell by (1) endocytosis; (2) which then accumulate through formation of endocytosis vesicles, and finally (3) the ions release from nanoparticles into the cell. ROS generation by NPs is mainly dependent on (a) interaction of nanoparticles with the mitochondria; (b) interaction of nanoparticles with NADPH oxidase; and (c) the physicochemical factors of the nanoparticles including size, shape, photoreactive properties, and the surface chemistry of the particles. These factors play an important role and lead to ROS generation and its severe consequences, including DNA and cell membrane damage, and cell apoptosis. Figure created by authors using BioRender.com. | |
There are several other diseases such as diabetes, osteoarthritis (OA), rheumatoid arthritis (RA), glaucoma, hearing loss, atherosclerosis, hypertension, restenosis, ischemia/reperfusion injury, and pulmonary and liver fibrosis that are highly affected by ROS. A potentially more favorable strategy for achieving therapeutic efficacy involves the targeted inhibition of enzymes responsible for the generation of reactive oxygen species. The occurrence of excessive formation of ROS is observed in several clinical states. Additionally, there exists a genetic factor that influences the likelihood of ROS generation, as supported by research.571
With over 170 million people worldwide affected, diabetes is a metabolic inflammatory disease that poses a significant threat to public health. Despite efforts to control the disease, its complications can only be slowed down rather than halted completely. The primary characteristic of diabetes is hyperglycemia, which initiates various metabolic signalling pathways that cause inflammation, cell death, and eventually lead to diabetic complications. The activation of diacylglycerol (DAG)–protein kinase C pathways by hyperglycemia is well-known to trigger a specific metabolic route. This signalling pathway is gaining more attention for its ability to regulate angiogenesis, reduce oxidative stress and prevent cellular death.572 It is widely believed that the disparity between the generation and removal of ROS and the resulting oxidative stress, induced by elevated blood glucose levels (hyperglycemia), is the primary factor contributing to the development of diabetic complications.573 In addition to hyperglycemia and ROS, Toll-like receptor 4 (TLR 4) activation can also trigger apoptosis. To control cardiac apoptosis in diabetes, researchers have found that silencing the TLR 4 gene or upregulating its expression in diabetic mice can be effective. This ultimately results in the reduction of oxidative stress and suppression of caspase 3 in cardiomyocytes.573 As a result, controlling ROS generation and TLR 4 downregulation may play a crucial role in managing diabetic complications.
Osteoarthritis is a degenerative joint condition that mostly impacts the geriatric population and is a significant contributor to functional impairment. The condition is distinguished by alterations in bone cells, resulting in softness, ulceration, fibrillation, degradation of articular cartilage, sclerosis of subchondral bone, inflammation of the synovial membrane, and the development of osteophytes and subchondral cysts. The mitochondria in OA produce an excessive amount of reactive oxygen species, leading to the oxidation of proteins. This oxidative process alters cell signalling pathways, favouring catabolic signalling, and ultimately contributing to cell death. A recent study has indicated that an elevated amount of ROS can interfere with the signalling pathways of IRS-1-PI-3 kinase-Akt, which is crucial for the integration of anabolic and catabolic signalling and the promotion of cell survival.574 The activation of Akt is hindered in OA chondrocytes and normal cells under oxidative stress, resulting in a decrease in matrix formation and an increase in vulnerability to cell death. Reactive oxygen species are mostly generated at minimal levels within articular chondrocytes, predominantly through the activity of NADPH oxidase. These entities play a significant role in the intracellular signalling processes that are involved in maintaining the equilibrium of cartilage. They achieve this by regulating many aspects such as chondrocyte death, gene expression, synthesis of the extracellular matrix (ECM), and cytokine production breakdown.575–577 Patients diagnosed with osteoarthritis have heightened levels of ROS generation and experience an increase in oxidative stress.578,579 The impact of interleukin-1 (IL-1) on DNA damage induced by ROS is significantly greater in cartilage afflicted by OA compared to healthy cartilage.580
Rheumatoid arthritis (RA) is a pathological condition characterized by the immune system's aberrant response, resulting in the degradation of several bodily structures, including joints, synovial tissue, cartilage, bone, and ligaments.581–583 HLA-DR-1 and HAL-DR-4 major histocompatibility complex (MHC) class II molecules exhibit a strong correlation with RA susceptibility in the human population. Additionally, DBA/1 and B10 strains have also been implicated in this association. The mice strains had I-Ag and I-Ar haplotypes, rendering them significantly vulnerable to collagen-induced arthritis (CIA), which serves as an experimental model for rheumatoid arthritis. The development of CIA in mice is associated with the expression of a limited T-cell receptor (TCR).584–586 The spontaneous development of arthritis in inbred mouse strains can be attributed to several factors, such as the expression of T cell receptor or TNF-α, as well as mutations in ZAP70 or Ncf1. There is a potential for these strains to have arthropathy, especially when exposed to specific environmental factors such as hormone imbalances, stress, or infections.587,588 There is a potential for these strains to have arthropathy, especially when exposed to specific environmental factors such as hormone imbalances, stress, or infections.589,590 Ncf1 is a constituent of the phagocyte NADPH oxidase complex, which plays a crucial role in modulating the magnitude of oxidative burst within phagocytes. Research has demonstrated that reduced levels of ROS generated by the NADPH oxidase complex have been found to exacerbate arthritis, inflammation, and immunological responses, contradicting commonly held beliefs.591
4.4. Tissue regeneration
Tissue regeneration is a dynamic process, which mainly includes cell migration and tissue reformation through morphogenesis followed by vigorous cell proliferation activity which commonly occurs at the traumatic or infected sites.592 There are complicated mechanisms underlying tissue regeneration including activation of specific cells or certain signalling molecules required for the effective recovery of injured tissues. Thus, it is important to understand intracellular and extracellular mechanisms with key mediators in tissue regeneration.593,594
Radical have been implicated as an initiator of tissue damage in various diseases, such as skin aging, cardiovascular disease, chronic diabetic wounds and so on.592,595 Oxidative stress due to an imbalance of oxidative and antioxidative byproducts contributes to the development of the diseases mentioned above. On the other hand, free radical species can be beneficial, acting as a mediator in cellular signalling for tissue regeneration, in particular when there is a high demand for mitochondrial production of adenosine triphosphate (ATP) during tissue renewal.593 ROS act as secondary messenger-signalling molecules that induce a respiratory chain reaction via oxidation and reduction.348,593 There are various signalling pathways reported to be involved in the ROS-mediated tissue regeneration response, such as Jun N-terminal kinase (JNK) and p38 mitogen-activated protein kinases (MAPK).596 ROS also play a role in regulating vasoconstriction and vasodilation, the important events in vascularization, an event that accompanies tissue regeneration.593 Nitric oxide, which belongs to reactive nitrogen species, reacts with a number of molecules including ROS to regulate a vascular relaxation.593 Essentially cells and molecules including macrophages, fibroblasts, platelets, keratinocytes and endothelial cells utilize these radicals to facilitate a healing response. Initial release of ROS activates vasoconstriction and reduces local cell signalling to enable thrombus formation. An antibacterial environment is then created after blood vessel-bound neutrophils are recruited to the injury site as shown in Fig. 17. Subsequently more ROS are released by phagocytosis to prevent the growth of bacteria and induce vital signals to other immunocytes in response to the pathogens at the wound site, including monocytes.593 These further stimulate endothelial cell division and migration for new ECM formation, which subsequently promotes keratinocyte proliferation and migration by activating angiogenesis and fibroblast division and migration.593
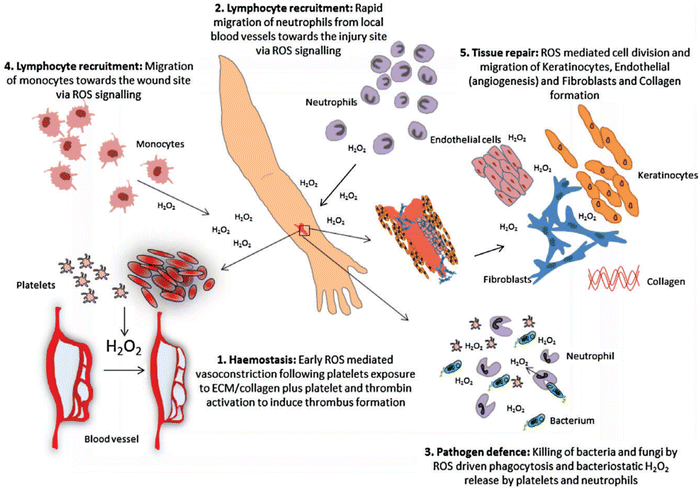 |
| Fig. 17 Schematic diagram illustrates the various role of ROS throughout severe wound healing conditions (i.e., homeostatic, level of ROS); (i) at early-stage, ROS help to reduce the blood flow and cell-signalling for thrombus formation, (ii) activated local neutrophils associated to blood vessels accumulate at the wound site for protection from bacteria, (iii) ROS produced by phagocytosis processes inhibit bacterial growth and help signal support for wound response, (iv) this helps to recruit immunocytes (monocytes) and help them to migrate towards to injury site to prevent attack invading pathogens, (v) at final stage, ROS stimulate endothelial cell differentiation and migration for blood vessel formation, and fibroblast differentiation and migration for new extracellular matrix formation and tissue regeneration. Reproduced with permission from Dunnill et al.593 [Copyright 2015, Wiley]. | |
ROS responsive nanoparticles can play a vital role in responding to oxidative stress in a physiological environment. Under such conditions, the nanoparticles are targeted to the injury site as they exhibit either switchable solubility or the ability to degrade chemical bonds to create an oxidative microenvironment.596 Their functionalities are highly dependent on the types of ROS being targeted, material structure/characteristics, and exposure time.596 Wang et al. have reported a novel bioactive chitosan nanoparticle-loaded calcium alginate hydrogel for wound healing.597 Apart from inducing an antibacterial environment even at a very low dosage, the formulation also facilitated proliferation and migration of vascular endothelial cells (VEC) via massive production of ROS that subsequently assisted in metastasis and neovascularization creating an effective wound healing effect.597 Tang et al. have demonstrated a ROS-reactive nanomaterial poly-(1,4-phenyleneacetone dimethylene thioketal) loaded with stromal cell-derived factor-1α (SDF-1α). The loaded nanoparticle is reported able to respond to ROS by delivering drugs to inflammatory tissues accumulated with large amount of ROS, which subsequently attracts BMSCs, triggering vascularisation and healing.598 The role of RANs in various cellular functions including DNA damage and cytotoxicity are presented (Table 4).
Table 4 Summary of the role of RANs in DNA damage and cytotoxicity, based on recent studies
RANs |
Application methods/surface modifications |
Brief outcomes |
Ref. |
Fe3O4 and Ag NPs |
Low-toxic concentration of deferoxamine (DFO) pre- treatment – applied in vitro HepG2 cells |
• The toxicity of AgNPs was reduced by using iron as a chelator. |
565 |
• AgNPs induced mitochondrial collapse, and O2˙− was produced and quickly dismutated to H2O2. |
• In the presence of iron ions, H2O2 underwent a Fenton reaction, resulting in the production of an extremely reactive hydroxyl radical (˙OH). |
• However, pre-treating AgNP-exposed cells with DFO significantly reduced the induction of DNA damage. |
TiOxNPs and (PAA-TiOxNPs) |
Polyacrylic acid-(PAA) modification |
• In vitro, the absorption of PAA-TiOxNPs increased DNA damage and led to greater cytotoxicity when exposed to X-ray irradiation. |
599 |
• In combination with X-ray treatment PAA-TiOxNPs had a significantly more powerful effect on inhibiting tumor growth than either treatment alone. |
Polyvinylpyrrolidone (PVP) + iron oxide nanoparticles |
S. aromaticum flower bud extract loading |
• The application of nanoparticles to the MCF-7 breast cancer cell line has resulted in a notable increase in the accumulation within the sub-G1 phase, hence leading to the initiation of apoptosis. |
600 |
• The augmentation in the quantity of nanoparticles resulted in a concomitant elevation in the enzymatic activity of caspase-3, caspase-8, and caspase-9. |
• Besides, the introduction of functionalized iron nanoparticles (FeNPs) led to the initiation of oxidative stress, which subsequently triggered the creation of ROS and promoted death in MCF-7 cells. |
• These findings underscore the promising prospects of using functionalized FeNPs in the field of cancer therapy. |
Cu/CuONPs |
Produced by chemical reductions |
• The toxicity of Cu/CuONPs was assessed by evaluating their effects on human lung carcinoma cell line (A549) and human lung normal cell line (WI-38). |
601 |
• The IC50 values of Cu/CuONPs were applied to both cell types, resulting in DNA damage and the generation of reactive oxygen species. |
• The potential utility of investigating the toxicological impacts of Cu/CuONPs on mature A549 cells lies in the development of targeted therapeutic delivery systems for certain cancer cell types. |
SiNPs |
Suspended in DI and applied to murine neuroblastoma cell line: Neuro-2a |
• The research conducted demonstrated that exposure to SiNPs resulted in a notable elevation of ROS levels within cellular structures. |
602 |
• Nevertheless, prior administration of the antioxidant N-acetylcysteine (NAC) successfully mitigated the generation of ROS and the subsequent decline in cell viability, occurrence of apoptotic events, and activation of molecules associated with endoplasmic reticulum (ER) stress. |
• The findings provide evidence that ROS plays a pivotal role in the harmful effects generated by silicon nanoparticles (SiNPs). |
• This involvement of ROS leads to the activation of ER stress and subsequent initiation of apoptosis, finally culminating in the demise of neuronal cells. |
ZnONPs |
Dispersed into the culture media and up-taken by HEK-293 cells |
• The concentration of ZnONPs has caused an excessive increase in ROS and O2 species, leading to impaired cellular oxidative stress management. |
603 |
• The expression of TET-methylcytosine dioxygenase genes has significantly increased, while DNA-methyltransferases (DNMTs) expression remains unchanged. |
• ZnONPs can cause a significant increase in ROS, resulting in various structural and functional abnormalities in cells. |
CeO2NPs |
Cellular internalization of nanoceria to the THP-1 cells |
• The study found that nanoceria has powerful antioxidant properties, as shown by its ability to scavenge radical in mammalian cells. |
566 |
• This suggests that nanoceria may be a promising treatment for conditions related to inflammation and oxidative stress. |
AuNPs |
Three types of 3–4 nm AuNPs on human A549 cells |
• After 24 hours, cells were found to have agglomerated AuNP, but this did not affect cell viability or inflammation. |
604 |
• Although no increase in ROS production was observed, however, intracellular glutathione levels decreased over time, indicating oxidative stress. |
• Furthermore, all three types of AuNPs caused DNA damage, with the strongest genotoxic effect observed for positively charged AuNP I. |
• However, despite the significant DNA damage caused by single exposure to AuNP I, A549 cells were able to recover and repair the damage over time. |
TiO2NPs |
Different concentration NPs orally presented to male rats |
• The administration of escalating doses of TiO2NPs was observed to induce elevated levels of cytotoxicity, oxidative stress, and apoptosis in both Caco-2 and HepG2 cell lines. |
605 |
• Additionally, it was shown that the inclusion of TiO2NPs resulted in a notable reduction in the activity of antioxidant enzymes and the expression of related genes, such as SOD, CAT, and GPx. |
• Furthermore, the levels of glutathione (GSH) and the overall capacity for antioxidant activity (TAC) were also found to be diminished. |
• The investigation revealed that the presence of TiO2NPs induced gene expressions associated with apoptosis and inflammatory pathways, along with caspase-3 activity in both the intestinal and hepatic tissues. |
SeNPs |
Hepatocellular carcinoma rat model (HCC) |
• The co-administration of doxorubicin and SeNPs resulted in a notable decrease in chromosomal abnormalities, micronuclei formation, and DNA damage in male rats with hepatocellular carcinoma (HCC) when compared to the administration of doxorubicin alone. |
606 |
AgNPs and AgNO3 |
Nematode Caenorhabditis elegans |
• The research demonstrated that the presence of NM300K AgNPs resulted in heightened production of ROSand elevated levels of oxidative stress, predominantly inside the intestinal lumen. |
607 |
• To conduct a more comprehensive examination of the relative toxicity of these NPs in comparison to AgNO3, the research employed SOD-1 and two biosensors, namely HyPer and GRX. |
• The findings of the study demonstrated that even at low concentrations, NM300K AgNPs induced cellular redox-potential instability and suggested the occurrence of oxidative stress. |
• Furthermore, the data consistently demonstrated discernible variations in behavior between the two forms of Ag, namely in relation to uptake, retention, toxic effects, and patterns of oxidative stress. |
Bi2O3NPs |
In vitro human breast cancer (MCF-7) cells |
• This study aimed to examine the impact of Bi2O3NPs on MCF-7 cells, with a specific focus on evaluating its cytotoxicity and induction of apoptosis. |
608 |
• The findings indicated that the presence of Bi2O3NPs resulted in a decrease in cell viability and induced damage to the cell membrane, with the extent of these effects being directly proportional to the dosage administered, and cell cycle was observed, along with the induction of oxidative stress responses. |
• This was demonstrated by the heightened production of ROS, elevated levels of lipid peroxidation, decreased GSH levels, and diminished activity of the SOD enzyme. |
• Additionally, the investigation revealed that Bi2O3NPs triggered apoptosis via the mitochondrial route, as evidenced by a decrease in mitochondrial membrane potential (MMP) and an elevated expression ratio of bax/bcl-2 genes. |
• In general, the results underscore the possible cytotoxic properties of Bi2O3NPs on MCF-7 cells. |
5. Conclusions and current and potential future clinical directions
The unique features of RANs such as intrinsic ROS production as well as their physicochemical properties such as particle shape, size, dimensions, surface chemical functionality, surface area, and imaging ability make them a promising nanoplatform for future application in biomedicine. The physical and chemical engineering of RANs has significantly changed their ability to generate ROS and provide control over pathological conditions. Furthermore, recent engineering of NPs has also helped to reduce their potential cytotoxicity, thereby improving the biosafety of NPs for therapeutic applications. Thanks to the advancement of nanoscience and nanotechnology, there are now many immense tools to synthesize various types of NPs and explore them as ROS-based therapeutic candidates in in vitro and in vivo applications.
ROS is an essential biochemical component in various pathological conditions, ranging from tissue injury/damage, infection/inflammation, tissue regeneration, cancer, OA, and neurodegenerative disease. Furthermore, ROS also plays a crucial multifunctional role in cell biology including cell signalling, cell viability, proliferation, differentiation, and cell apoptosis. As a result, controlling and treating diseases by regulating the excess or deficiency of ROS in tissue/organ/body using RANs in the form of ROS generating or scavenging NPs, is possible. However, concerns about potential toxicities and the ability to target specific cells are significant. Some RANs may not be inherently biocompatible, and their introduction into the body could lead to adverse reactions such as inflammation, oxidative stress, or cellular damage. Moreover, some RANs release toxic ions or molecules during their redox-reactions, causing harm to cells or tissues. For example, certain metals used in nanoparticles formulations could pose toxicity risks. Additionally, if these nanoparticles are not effectively cleared from the body, they can accumulate in the organs such as the liver, spleen, kidneys, and leads to the potential toxicity over time. Furthermore, RANs face biological barriers, such as the blood brain or endothelial barriers in tumors, which can limit their ability to reach target cells or tissues. Therefore, precisely targeting RANs to specific cells is also a challenge for RANs.
Targeted application of redox-active nanoparticles in cells, tissues, or within the body requires advanced design and engineering to lower the energy barriers of ROS associated catalytic reactions within location specific conditions to regulate the favorable in vivo ROS-based therapeutic effect. However, these local in vivo physiological conditions (pH, temperature) can also trigger the ROS catalytic reactions by exciting the energy level of NPs, and thus can have severe consequences on health. Further exploration of the generation or scavenging of ROS by NPs and their associated cellular and molecular mechanisms is therefore required. A thorough understanding of these mechanisms will help significantly in efforts to engineer NPs with appropriate physicochemical functionalities.
Advancements in nanotechnology, biomaterials, and biomedical engineering are pushing towards overcoming challenges in medicine and unlocking the full potential of RANs. For instance, multifunctional nanoparticles adorned with targeting ligands and responsive moieties are making precision delivery of therapeutic agents to diseased tissues a reality. These strategies could enhance treatment efficacy while reducing the off-target effects and systemic toxicity. In future, developed RANs may respond to specific stimuli, prompting controlled drug release at the target site, which could improve treatment efficacy while minimizing side effects. Multi-therapeutic agent delivery is also promising, holding the potential to overcome drug resistance and improve treatment outcomes. Lastly, RANs with intrinsic fluorescence or luminescence properties could be used for minimally invasive in vivo bioimaging and biosensing, enabling early disease detection, monitoring, and personalized treatment approaches. Future redox-active nanomaterials will likely exhibit improved biodistribution, biodegradability, and clearance from the body, reducing the long-term accumulation and potential toxicity. Biocompatible degradation products could also be engineered to ensure safe elimination via renal or hepatobiliary pathways.
A common concern across the various nanomedicine modalities is their potential adverse effects on human health and safety. While RANs hold promises in preclinical studies for disease prevention and treatment, however their clinical applications remain limited, with research predominantly focused on in vitro studies and animal experiments. These models do not fully replicate human pathological conditions, particularly metastatic tumors, posing challenges in accurately mimicking the cellular or tissue damage caused by oxidative stress in physiological conditions.609 Moreover, the lack of clinical trial results is a major factor restricting the clinical translation of RANs. One of the key challenges includes inadequate methods for evaluating biocompatibility, which require a deeper understanding of toxicity mechanisms and enhanced in vivo cytotoxicity detection. This is mainly due to conventional antioxidants possess a double-edged sword effect.610 They suffer from low bioavailability due to poor gut absorption and nonspecific distribution, which reduces their local concentration and often leads to a failure in neutralizing excess free radicals.611 Conversely, high local concentrations can disrupt crucial ROS-mediated signalling pathways necessary for cell function and, in some cases, act as prooxidants, exacerbating oxidative damage.610,611 Additionally, they lack reusability, as they become inactive after neutralizing ROS through self-oxidation.611 Hence, to develop a comprehensive biocompatibility evaluation index, systemic toxicity testing must be performed, emphasizing the retention of nanomaterials in vital organs like the liver and kidneys, coupled with in vitro biosafety evaluations using human cell lines, which are also essential for advancing clinical trials.609
Advancements in nanotechnology offer various methods for modifying nanomaterials, such as using small molecules, polymers, antibodies, or nucleic acids, for targeted delivery by attaching cell-specific ligands that bind to receptors overexpressed in particular tissues.612 For example, nanomaterials capped with folate can precisely target cancer cells, which have higher folate receptor expression than normal cells, allowing for controlled local concentrations and maintaining cellular redox balance.611 Although nanomaterials may exhibit inherent properties such as ideal size, shape, hydrophobicity, rigidity, and composition, influencing biodistribution within the body, another important challenge is that redox-active nanodrugs, despite their longer-lasting effects and reduced side effects compared to traditional drugs, currently lack sufficient therapeutic efficacy for widespread clinical use, partly due to high design costs.609 These costs are associated with the complex structures of redox nanodrugs, especially those involved in multifunctional nanoplatform therapy systems. Additionally, RANs with strong permeability and retention effects often have low drug delivery efficiencies, highlighting the need for precise controlled release capabilities.609 While these nanoparticles show promise in diagnostics and bioassays, their therapeutic application faces challenges, including their use in topical or injectable solutions and implants. Some, like citrate-functionalized Mn3O4NPs, have shown success in preclinical trials, but others need further animal testing to confirm the efficacy observed in vitro.611 Injected nanoparticles must have minimal side effects and efficient excretion pathways, as certain materials like gold cannot be metabolized and must be excreted via urine or faeces.613 Their interaction with biological fluids and the need for precise targeting without causing toxicity are crucial considerations. Although simpler nanoparticle designs have paved the way for initial attempts, more complex structures pose significant translational challenges. Despite these complexities, progress has led to FDA-approved nanomedicines.614 However, more research on catalytic activity, therapeutic efficacy, and safety is needed to advance clinical applications effectively.611
In essence, addressing these challenges by selecting more cost-effective redox-responsive nanomaterials and improving synthesis methods can enhance their practicality and efficacy. Ultimately, it is crucial to understand comprehensively how these nanomaterials induce unintended effects on the immune and nervous systems. This necessitates a shift from descriptive toxicology to predictive toxicology.615 Given the diverse array of nanomaterial types and formulations, individual assessments for each are not feasible. Therefore, employing predictive toxicology models alongside high-content cellular screening emerges as a practical and promising approach to evaluate nanomaterial toxicity in humans, gradually making them applicable in clinical medicine.616
Overall, the field of redox-active nanomaterials in medicine will rely heavily on cross-disciplinary collaboration, inventive nanomaterial design, fabrication techniques, and thorough preclinical and clinical testing to guarantee both safety and effectiveness. With ongoing improvements in our comprehension of nanoscale phenomena and biological mechanisms, RANs are poised to transform personalized medicine in areas such as diagnostics tools, imaging technologies, drug administration, and therapeutic interventions.
Data availability
The data that support the finding of this review study are openly available and collected from the published research articles, review papers with permission of publishers.
Conflicts of interest
The authors declare that they have no financial interests or personal conflict of interest.
Acknowledgements
The authors would like to acknowledge the Engineering and Physical Science Research Council (EPSRC), the UK for empower program at University of Bristol under grant no. EP/T020792/1, and the National Research Foundation (NRF; 2021R1A5A2022318, RS-2024-00348908, and RS-2023-00220408) of Republic of Korea. The Biotechnology and Biological Science Research Council (BBSRC) London Interdisciplinary Bioscience PhD Consortium (LIDo; BB/M009513/1).
References
- B. Perillo, M. Di Donato, A. Pezone, E. Di Zazzo, P. Giovannelli, G. Galasso, G. Castoria and A. Migliaccio, Exp. Mol. Med., 2020, 52, 192–203 Search PubMed.
- H. Sies and D. P. Jones, Nat. Rev. Mol. Cell Biol., 2020, 21, 363–383 Search PubMed.
- C.-S. Lee, R. K. Singh, H. S. Hwang, N.-H. Lee, A. G. Kurian, J.-H. Lee, H. S. Kim, M. Lee and H.-W. Kim, Prog. Mater. Sci., 2023, 135, 101087 Search PubMed.
- J. Bi, J. Zhang, Y. Ren, Z. Du, Q. Li, Y. Wang, S. Wei, L. Yang, J. Zhang, C. Liu, Y. Lv and R. Wu, Redox Biol., 2019, 20, 296–306 Search PubMed.
- F. Pagliari, C. Mandoli, G. Forte, E. Magnani, S. Pagliari, G. Nardone, S. Licoccia, M. Minieri, P. Di Nardo and E. Traversa, ACS Nano, 2012, 6, 3767–3775 Search PubMed.
- T. Zhou, E. R. Prather, D. E. Garrison and L. Zuo, Int. J. Mol. Sci., 2018, 19(2), 417 Search PubMed.
- N. Solovyev, E. Drobyshev, G. Bjørklund, Y. Dubrovskii, R. Lysiuk and M. P. Rayman, Free Radicals Biol. Med., 2018, 127, 124–133 Search PubMed.
- M. P. Murphy, H. Bayir, V. Belousov, C. J. Chang, K. J. A. Davies, M. J. Davies, T. P. Dick, T. Finkel, H. J. Forman, Y. Janssen-Heininger, D. Gems, V. E. Kagan, B. Kalyanaraman, N.-G. Larsson, G. L. Milne, T. Nyström, H. E. Poulsen, R. Radi, H. Van Remmen, P. T. Schumacker, P. J. Thornalley, S. Toyokuni, C. C. Winterbourn, H. Yin and B. Halliwell, Nat. Metab., 2022, 4, 651–662 Search PubMed.
- A. Marino, M. Battaglini, N. Moles and G. Ciofani, ACS Omega, 2022, 7, 25974–25990 Search PubMed.
- S. Mollazadeh, M. Mackiewicz and M. Yazdimamaghani, Mater. Sci. Eng., C, 2021, 118, 111536 Search PubMed.
- L. Han, X.-Y. Zhang, Y.-L. Wang, X. Li, X.-H. Yang, M. Huang, K. Hu, L.-H. Li and Y. Wei, J. Controlled Release, 2017, 259, 40–52 Search PubMed.
- Y. Liu, P. Bhattarai, Z. Dai and X. Chen, Chem. Soc. Rev., 2019, 48, 2053–2108 Search PubMed.
- M. Hao, B. Chen, X. Zhao, N. Zhao and F.-J. Xu, Mater. Chem. Front., 2020, 4, 2571–2609 Search PubMed.
- A. Moazzen, N. Öztinen, E. Ak-Sakalli and M. Koşar, Heliyon, 2022, 8, e10467 Search PubMed.
- Q. Guo, S. Ji, Q. Yue, L. Wang, J. Liu and J. Jia, Anal. Chem., 2009, 81, 5381–5389 Search PubMed.
- G. Goncalves, P. A. A. P. Marques, C. M. Granadeiro, H. I. S. Nogueira, M. K. Singh and J. Grácio, Chem. Mater., 2009, 21, 4796–4802 Search PubMed.
- J. Bai Aswathanarayan, R. Rai Vittal and U. Muddegowda, Artif. Cells, Nanomed., Biotechnol., 2018, 46, 1444–1451 Search PubMed.
- S. Franco-Ulloa, D. Guarnieri, L. Riccardi, P. P. Pompa and M. De Vivo, J. Chem. Theory Comput., 2021, 17, 4512–4523 Search PubMed.
- Z. Nie, K. J. Liu, C.-J. Zhong, L.-F. Wang, Y. Yang, Q. Tian and Y. Liu, Free Radicals Biol. Med., 2007, 43, 1243–1254 Search PubMed.
- S. M. Ansar, S. Chakraborty and C. L. Kitchens, Nanomaterials, 2018, 8, 339 Search PubMed.
- T. Yasukawa, H. Miyamura and S. Kobayashi, ACS Catal., 2016, 6, 7979–7988 Search PubMed.
- K. N. L. Hoang, S. M. McClain, S. M. Meyer, C. A. Jalomo, N. B. Forney and C. J. Murphy, Chem. Commun., 2022, 58, 9728–9741 Search PubMed.
- P. Vardakas, I. A. Kartsonakis, I. D. Kyriazis, P. Kainourgios, A. F. A. Trompeta, C. A. Charitidis and D. Kouretas, Environ. Res., 2023, 220, 115156 Search PubMed.
- Y. González-García, E. R. López-Vargas, G. Cadenas-Pliego, A. Benavides-Mendoza, S. González-Morales, A. Robledo-Olivo, Á. G. Alpuche-Solís and A. Juárez-Maldonado, Int. J. Mol. Sci., 2019, 20, 5858 Search PubMed.
- K. D. Patel, R. K. Singh and H.-W. Kim, Mater. Horiz., 2019, 6, 434–469 Search PubMed.
- I. L. Medintz, H. T. Uyeda, E. R. Goldman and H. Mattoussi, Nat. Mater., 2005, 4, 435–446 Search PubMed.
- C. M. Sims, S. K. Hanna, D. A. Heller, C. P. Horoszko, M. E. Johnson, A. R. Montoro Bustos, V. Reipa, K. R. Riley and B. C. Nelson, Nanoscale, 2017, 9, 15226–15251 Search PubMed.
- B. Yang, Y. Chen and J. Shi, Chem. Rev., 2019, 119, 4881–4985 Search PubMed.
- Y. Nosaka and A. Y. Nosaka, Chem. Rev., 2017, 117, 11302–11336 Search PubMed.
- X. Shi, Y. Tian, S. Zhai, Y. Liu, S. Chu and Z. Xiong, Front. Chem., 2023, 11, 1115440 Search PubMed.
- P. Chen, W. Zhang, X. Fan, X. Shi, Y. Jiang, L. Yan, H. Li, C. Wang, L. Han, X. Lu and C. Ou, Nano Today, 2024, 55, 102157 Search PubMed.
- P. H. Hoet, B. Nemery and D. Napierska, Nano Today, 2013, 8, 223–227 Search PubMed.
- H. Sies, Redox Biol., 2015, 4, 180–183 Search PubMed.
- A. Costa, A. Scholer-Dahirel and F. Mechta-Grigoriou, Semin. Cancer Biol., 2014, 25, 23–32 Search PubMed.
- S. H. Yoo, H.-W. Kim and J. H. Lee, J. Tissue Eng., 2022, 13, 1–14, DOI:10.1177/20417314221083414.
- Y. Vakrat-Haglili, L. Weiner, V. Brumfeld, A. Brandis, Y. Salomon, B. McLlroy, B. C. Wilson, A. Pawlak, M. Rozanowska, T. Sarna and A. Scherz, J. Am. Chem. Soc., 2005, 127, 6487–6497 Search PubMed.
- M. Wang, M. Chang, C. Li, Q. Chen, Z. Hou, B. Xing and J. Lin, Adv. Mater., 2022, 34, 2106010 Search PubMed.
- Y. Lin, X. Chen, C. Yu, G. Xu, X. Nie, Y. Cheng, Y. Luan and Q. Song, Acta Biomater., 2023, 159, 300–311 Search PubMed.
- M. Sharifi-Rad, N. V. Anil Kumar, P. Zucca, E. M. Varoni, L. Dini, E. Panzarini, J. Rajkovic, P. V. Tsouh Fokou, E. Azzini, I. Peluso, A. Prakash Mishra, M. Nigam, Y. El Rayess, M. E. Beyrouthy, L. Polito, M. Iriti, N. Martins, M. Martorell, A. O. Docea, W. N. Setzer, D. Calina, W. C. Cho and J. Sharifi-Rad, Front. Physiol., 2020, 11, 694 Search PubMed.
- E. Birben, U. M. Sahiner, C. Sackesen, S. Erzurum and O. Kalayci, World Allergy Organ. J., 2012, 5, 9–19 Search PubMed.
- C. M. C. Andrés, J. M. Pérez de la Lastra, C. Andrés Juan, F. J. Plou and E. Pérez-Lebeña, Int. J. Mol. Sci., 2023, 24, 1841 Search PubMed.
- J. Fujii, T. Homma and T. Osaki, Antioxidants, 2022, 11, 501 Search PubMed.
- B. Lipinski and E. Pretorius, Hematology, 2012, 17, 241–247 Search PubMed.
- B. Lipinski, Oxid. Med. Cell. Longevity, 2011, 2011, 809696 Search PubMed.
- S. Adhikari, R. Crehuet, J. M. Anglada, J. S. Francisco and Y. Xia, Proc. Natl. Acad. Sci. U. S. A., 2020, 117, 18216–18223 Search PubMed.
- P. Chaudhary, P. Janmeda, A. O. Docea, B. Yeskaliyeva, A. F. Abdull Razis, B. Modu, D. Calina and J. Sharifi-Rad, Front. Chem., 2023, 11, 1158198 Search PubMed.
- L. M. Smith, H. M. Aitken and M. L. Coote, Acc. Chem. Res., 2018, 51, 2006–2013 Search PubMed.
- J. Helberg and D. A. Pratt, Chem. Soc. Rev., 2021, 50, 7343–7358 Search PubMed.
- C. von Sonntag, in Mechanisms of DNA Damage and Repair: Implications for Carcinogenesis and Risk Assessment, ed. M. G. Simic, L. Grossman, A. C. Upton and D. S. Bergtold, Springer, US, Boston, MA, 1986, pp. 51–59 DOI:10.1007/978-1-4615-9462-8_6.
- K. Jomova, R. Raptova, S. Y. Alomar, S. H. Alwasel, E. Nepovimova, K. Kuca and M. Valko, Arch. Toxicol., 2023, 97, 2499–2574 Search PubMed.
- K. Wang, W. Mao, X. Song, M. Chen, W. Feng, B. Peng and Y. Chen, Chem. Soc. Rev., 2023, 52, 6957–7035 Search PubMed.
- V. Nayak, K. R. B. Singh, A. K. Singh and R. P. Singh, New J. Chem., 2021, 45, 2849–2878 Search PubMed.
- F. Collin, Int. J. Mol. Sci., 2019, 20(10), 2407 Search PubMed.
- D. D. Saraev, Z. Wu, H.-Y. H. Kim, N. A. Porter and D. A. Pratt, ACS Chem. Biol., 2023, 18, 2073–2081 Search PubMed.
- L. K. Folkes, S. Bartesaghi, M. Trujillo, P. Wardman and R. Radi, Int. J. Mol. Sci., 2022, 23, 1797 Search PubMed.
- E. Illés, A. Mizrahi, V. Marks and D. Meyerstein, Free Radicals Biol. Med., 2019, 131, 1–6 Search PubMed.
- R. Luc and C. Vergely, Int. J. Biomed. Sci., 2008, 4, 255–259 Search PubMed.
- A. M. Fleming and C. J. Burrows, Chem. Soc. Rev., 2020, 49, 6524–6528 Search PubMed.
- R. Xiao, Y. Meng, Y. Fu, S. Wacławek, Z. Wei, R. Spinney, D. D. Dionysiou, W. Zeng and W. P. Hu, Chem. Eng. J., 2023, 474, 145245 Search PubMed.
- L. Wojnárovits, T. Tóth and E. Takács, Sci. Total Environ., 2020, 717, 137219 Search PubMed.
- S. M. Andrabi, N. S. Sharma, A. Karan, S. M. S. Shahriar, B. Cordon, B. Ma and J. Xie, Adv. Sci., 2023, 10, 2303259 Search PubMed.
- M. K. Chug and E. J. Brisbois, ACS Mater. Au, 2022, 2, 525–551 Search PubMed.
- Y. Deng, F. Jia, S. Chen, Z. Shen, Q. Jin, G. Fu and J. Ji, Biomaterials, 2018, 187, 55–65 Search PubMed.
- E. Lubos, D. E. Handy and J. Loscalzo, Front. Biosci.-Landmark, 2008, 13, 5323–5344 Search PubMed.
- C. Schöneich, Molecules, 2019, 24(23), 4357 Search PubMed.
- Y. M. Lee, W. He and Y.-C. Liou, Cell Death Dis., 2021, 12, 58 Search PubMed.
- T. Nauser, W. H. Koppenol and C. Schöneich, Free Radicals Biol. Med., 2015, 80, 158–163 Search PubMed.
- D. M. Lynch and E. M. Scanlan, Molecules, 2020, 25, 3094 Search PubMed.
- M. Janků, L. Luhová and M. Petřivalský, Antioxidants, 2019, 8, 105 Search PubMed.
- T. Kietzmann, A. Petry, A. Shvetsova, J. M. Gerhold and A. Görlach, Br. J. Pharmacol., 2017, 174, 1533–1554 Search PubMed.
- B. Chance, H. Sies and A. Boveris, Physiol. Rev., 1979, 59, 527–605 Search PubMed.
- J. F. Turrens, J. Physiol., 2003, 552, 335–344 Search PubMed.
- R. Z. Zhao, S. Jiang, L. Zhang and Z. B. Yu, Int. J. Mol. Med., 2019, 44, 3–15 Search PubMed.
- H. Y. Chung, G. S. Lee, S. H. Nam, J. H. Lee, J. P. Han, S. Song, G.-D. Kim, C. Jung, D. Y. Hyeon, D. Hwang, B.-O. Choi and S. C. Yeom, Brain, 2024, 147, 2114–2127 Search PubMed.
- S. Lenzen, V. I. Lushchak and F. Scholz, Arch. Toxicol., 2022, 96, 1915–1920 Search PubMed.
- J. P. Crow and J. S. Beckman, in The Role of Nitric Oxide in Physiology and Pathophysiology, ed. H. Koprowski and H. Maeda, Springer Berlin Heidelberg, Berlin, Heidelberg, 1995, pp. 57–73 DOI:10.1007/978-3-642-79130-7_7.
- R. Radi, Proc. Natl. Acad. Sci. U. S. A., 2018, 115, 5839–5848 Search PubMed.
- C. M. C. Andrés, J. M. Pérez de la Lastra, C. Andrés Juan, F. J. Plou and E. Pérez-Lebeña, Int. J. Mol. Sci., 2023, 24, 1841 Search PubMed.
- R. Campos Chisté, M. Freitas, A. Zerlotti Mercadante and E. Fernandes, Curr. Med. Chem., 2015, 22, 4234–4256 Search PubMed.
- J. H. Kramer, B. F. Dickens, V. Mišík and W. B. Weglicki, J. Mol. Cell. Cardiol., 1995, 27, 371–381 Search PubMed.
- N. A. Popova, G. M. Sysoeva, V. P. Nikolin, V. I. Kaledin, E. V. Tretyakov, M. V. Edeeva, S. M. Balakhnin, E. L. Lushnikova, G. Audran and S. Mark, Bull. Exp. Biol. Med., 2017, 164, 49–53 Search PubMed.
- D. S. Dimić, D. A. Milenković, E. H. Avdović, J. Nakarada, J. M. Dimitrić Marković and Z. S. Marković, Chem. Eng. J., 2021, 424, 130331 Search PubMed.
- Z. Hao, J. Ma, C. Miao, Y. Song, L. Lian, S. Yan and W. Song, Environ. Sci. Technol., 2020, 54, 10118–10127 Search PubMed.
- D. B. Medinas, G. Cerchiaro, D. F. Trindade and O. Augusto, IUBMB Life, 2007, 59, 255–262 Search PubMed.
- B. N. Gantner, K. M. LaFond and M. G. Bonini, Redox Biol., 2020, 34, 101550 Search PubMed.
- Y. Liu, G. Fiskum and D. Schubert, J. Neurochem., 2002, 80, 780–787 Search PubMed.
- S. Chenna, W. J. H. Koopman, J. H. M. Prehn and N. M. C. Connolly, Am. J. Physiol.: Cell Physiol., 2022, 323, C69–C83 Search PubMed.
- Z. Yang and D. J. Klionsky, Curr. Opin. Cell Biol., 2010, 22, 124–131 Search PubMed.
- V. P. Tan and S. Miyamoto, J. Mol. Cell. Cardiol., 2016, 95, 31–41 Search PubMed.
- C. C. Dibble and B. D. Manning, Nat. Cell Biol., 2013, 15, 555–564 Search PubMed.
- G. Filomeni, D. De Zio and F. Cecconi, Cell Death Differ., 2015, 22, 377–388 Search PubMed.
- G. Filomeni, D. De Zio and F. Cecconi, Cell Death Differ., 2015, 22, 377–388 Search PubMed.
- B. Groitl and U. Jakob, Biochim. Biophys. Acta, Proteins Proteomics, 2014, 1844, 1335–1343 Search PubMed.
- T. M. Buttke and P. A. Sandstrom, Immunol. Today, 1994, 15, 7–10 Search PubMed.
- A. Nugud, D. Sandeep and A. T. El-Serafi, J. Adv. Res., 2018, 14, 73–79 Search PubMed.
- Z. Ghanbari Movahed, M. Rastegari-Pouyani, M. H. Mohammadi and K. Mansouri, Biomed. Pharmacother., 2019, 112, 108690 Search PubMed.
- R. Bhowmick and R. R. Sarkar, PLoS One, 2020, 15, e0235204 Search PubMed.
- C. E. Murry, M. H. Soonpaa, H. Reinecke, H. Nakajima, H. O. Nakajima, M. Rubart, K. B. S. Pasumarthi, J. Ismail Virag, S. H. Bartelmez, V. Poppa, G. Bradford, J. D. Dowell, D. A. Williams and L. J. Field, Nature, 2004, 428, 664–668 Search PubMed.
- R. D. Unwin, D. L. Smith, D. Blinco, C. L. Wilson, C. J. Miller, C. A. Evans, E. Jaworska, S. A. Baldwin, K. Barnes and A. Pierce, Blood, 2006, 107, 4687–4694 Search PubMed.
- J. M. Funes, M. Quintero, S. Henderson, D. Martinez, U. Qureshi, C. Westwood, M. O. Clements, D. Bourboulia, R. B. Pedley and S. Moncada, Proc. Natl. Acad. Sci. U. S. A., 2007, 104, 6223–6228 Search PubMed.
- J.-H. Paik, Z. Ding, R. Narurkar, S. Ramkissoon, F. Muller, W. S. Kamoun, S.-S. Chae, H. Zheng, H. Ying and J. Mahoney, Cell Stem Cell, 2009, 5, 540–553 Search PubMed.
- F. Kocabas, J. Zheng, S. Thet, N. G. Copeland, N. A. Jenkins, R. J. DeBerardinis, C. Zhang and H. A. Sadek, Blood, 2012, 120, 4963–4972 Search PubMed.
- T. Suda, K. Takubo and G. L. Semenza, Cell Stem Cell, 2011, 9, 298–310 Search PubMed.
- J. M. Ryu, H. J. Lee, Y. H. Jung, K. H. Lee, D. I. Kim, J. Y. Kim, S. H. Ko, G. E. Choi, I. I. Chai, E. J. Song, J. Y. Oh, S.-J. Lee and H. J. Han, Int. J. Stem Cells, 2015, 8, 24–35 Search PubMed.
- M. L. Circu and T. Y. Aw, Free Radical Biol. Med., 2010, 48, 749–762 Search PubMed.
- S. V. Avery, Biochem. J., 2011, 434, 201–210 Search PubMed.
- P. Storz, Front. Biosci., 2005, 10, 1881–1896 Search PubMed.
- D. Trachootham, Y. Zhou, H. Zhang, Y. Demizu, Z. Chen, H. Pelicano, P. J. Chiao, G. Achanta, R. B. Arlinghaus, J. Liu and P. Huang, Cancer Cell, 2006, 10, 241–252 Search PubMed.
- M. Azmanova and A. Pitto-Barry, ChemBioChem, 2022, 23, e202100641 Search PubMed.
- E. Panieri and M. M. Santoro, Cell Death Dis., 2016, 7, e2253–e2253 Search PubMed.
- R. K. Singh, K. D. Patel, C. Mahapatra, S. P. Parthiban, T.-H. Kim and H.-W. Kim, ACS Appl. Mater. Interfaces, 2019, 11, 288–299 Search PubMed.
- G. Y. Liou and P. Storz, Free Radical Res., 2010, 44, 479–496 Search PubMed.
- P. Storz, in Oxidative Stress and Redox Regulation, ed. U. Jakob and D. Reichmann, Springer, Netherlands, Dordrecht, 2013, pp. 427–447 DOI:10.1007/978-94-007-5787-5_15.
- S. Shibuya, Y. Ozawa, K. Watanabe, N. Izuo, T. Toda, K. Yokote and T. Shimizu, PLoS One, 2014, 9, e109288 Search PubMed.
- D. Yang, S. G. Elner, Z.-M. Bian, G. O. Till, H. R. Petty and V. M. Elner, Exp. Eye Res., 2007, 85, 462–472 Search PubMed.
- J. Krstic, D. Trivanovic, S. Mojsilovic and J. F. Santibanez, Oxid. Med. Cell. Longevity, 2015, 2015, 654594 Search PubMed.
- L. E. Huang, J. Gu, M. Schau and H. F. Bunn, Proc. Natl. Acad. Sci. U. S. A., 1998, 95, 7987–7992 Search PubMed.
- M. R. Abid, K. C. Spokes, S.-C. Shih and W. C. Aird, J. Biol. Chem., 2007, 282, 35373–35385 Search PubMed.
- Y. Higaki, T. Mikami, N. Fujii, M. F. Hirshman, K. Koyama, T. Seino, K. Tanaka and L. J. Goodyear, Am. J. Physiol.: Endocrinol. Metab., 2008, 294, E889–E897 Search PubMed.
- T. Minamoto, M. Mai and Z. Ronai, Cancer Detect. Prev., 2000, 24, 1–12 Search PubMed.
- T. Minamoto, A. V. Ougolkov and M. Mai, Expert Rev. Mol. Diagn., 2002, 2, 565–575 Search PubMed.
- D. Ferraro, S. Corso, E. Fasano, E. Panieri, R. Santangelo, S. Borrello, S. Giordano, G. Pani and T. Galeotti, Oncogene, 2006, 25, 3689–3698 Search PubMed.
- V. Chavda, B. Chaurasia, K. Garg, H. Deora, G. E. Umana, P. Palmisciano, G. Scalia and B. Lu, Brain Disord., 2022, 5, 100029 Search PubMed.
- B. S. Berlett and E. R. Stadtman, J. Biol. Chem., 1997, 272, 20313–20316 Search PubMed.
- M. Mittal, M. R. Siddiqui, K. Tran, S. P. Reddy and A. B. Malik, Antioxid. Redox Signaling, 2014, 20, 1126–1167 Search PubMed.
- S. Salzano, P. Checconi, E.-M. Hanschmann, C. H. Lillig, L. D. Bowler, P. Chan, D. Vaudry, M. Mengozzi, L. Coppo, S. Sacre, K. R. Atkuri, B. Sahaf, L. A. Herzenberg, L. A. Herzenberg, L. Mullen and P. Ghezzi, Proc. Natl. Acad. Sci. U. S. A., 2014, 111, 12157–12162 Search PubMed.
- M. Schäfer and S. Werner, Pharmacol. Res., 2008, 58, 165–171 Search PubMed.
- I. Liguori, G. Russo, F. Curcio, G. Bulli, L. Aran, D. Della-Morte, G. Gargiulo, G. Testa, F. Cacciatore, D. Bonaduce and P. Abete, Clin. Interventions Aging, 2018, 13, 757–772 Search PubMed.
- M. Cano Sanchez, S. Lancel, E. Boulanger and R. Neviere, Antioxidants, 2018, 7(8), 98 Search PubMed.
- R. Moseley, J. E. Stewart, P. Stephens, R. J. Waddington and D. W. Thomas, Br. J. Dermatol., 2004, 150, 401–413 Search PubMed.
- C. Caspersen, N. Wang, J. Yao, A. Sosunov, X. Chen, J. W. Lustbader, H. W. Xu, D. Stern, G. McKhann and S. D. Yan, FASEB J., 2005, 19, 2040–2041 Search PubMed.
- C. S. Casley, L. Canevari, J. M. Land, J. B. Clark and M. A. Sharpe, J. Neurochem., 2002, 80, 91–100 Search PubMed.
- L. S. Forno, J. Neuropathol. Exp. Neurol., 1996, 55, 259–272 Search PubMed.
- M. G. Spillantini, R. A. Crowther, R. Jakes, M. Hasegawa and M. Goedert, Proc. Natl. Acad. Sci. U. S. A., 1998, 95, 6469–6473 Search PubMed.
- E. M. Valente, P. M. Abou-Sleiman, V. Caputo, M. M. K. Muqit, K. Harvey, S. Gispert, Z. Ali, D. Del Turco, A. R. Bentivoglio, D. G. Healy, A. Albanese, R. Nussbaum, R. González-Maldonado, T. Deller, S. Salvi, P. Cortelli, W. P. Gilks, D. S. Latchman, R. J. Harvey, B. Dallapiccola, G. Auburger and N. W. Wood, Science, 2004, 304, 1158–1160 Search PubMed.
- W. C. Nichols, N. Pankratz, D. Hernandez, C. Paisán-Ruíz, S. Jain, C. A. Halter, V. E. Michaels, T. Reed, A. Rudolph, C. W. Shults, A. Singleton and T. Foroud, Lancet, 2005, 365, 410–412 Search PubMed.
- B. Xiao, J.-Y. Goh, L. Xiao, H. Xian, K.-L. Lim and Y.-C. Liou, J. Biol. Chem., 2017, 292, 16697–16708 Search PubMed.
- W. J. Szlachcic, P. M. Switonski, W. J. Krzyzosiak, M. Figlerowicz and M. Figiel, Dis. Models Mech., 2015, 8, 1047 Search PubMed.
- F. O. Walker, Lancet, 2007, 369, 218–228 Search PubMed.
- B. Rotblat, A. L. Southwell, D. E. Ehrnhoefer, N. H. Skotte, M. Metzler, S. Franciosi, G. Leprivier, S. P. Somasekharan, A. Barokas, Y. Deng, T. Tang, J. Mathers, N. Cetinbas, M. Daugaard, B. Kwok, L. Li, C. J. Carnie, D. Fink, R. Nitsch, J. D. Galpin, C. A. Ahern, G. Melino, J. M. Penninger, M. R. Hayden and P. H. Sorensen, Proc. Natl. Acad. Sci. U. S. A., 2014, 111, 3032–3037 Search PubMed.
- A. Johri and M. F. Beal, Biochim. Biophys. Acta, Mol. Basis Dis., 2012, 1822, 664–674 Search PubMed.
- C. Giorgi, S. Marchi, I. C. M. Simoes, Z. Ren, G. Morciano, M. Perrone, P. Patalas-Krawczyk, S. Borchard, P. Jędrak, K. Pierzynowska, J. Szymański, D. Q. Wang, P. Portincasa, G. Węgrzyn, H. Zischka, P. Dobrzyn, M. Bonora, J. Duszynski, A. Rimessi, A. Karkucinska-Wieckowska, A. Dobrzyn, G. Szabadkai, B. Zavan, P. J. Oliveira, V. A. Sardao, P. Pinton and M. R. Wieckowski, Int. Rev. Cell Mol. Biol., 2018, 340, 209–344 Search PubMed.
- A. N. Kolodkin, R. P. Sharma, A. M. Colangelo, A. Ignatenko, F. Martorana, D. Jennen, J. J. Briedé, N. Brady, M. Barberis, T. D. G. A. Mondeel, M. Papa, V. Kumar, B. Peters, A. Skupin, L. Alberghina, R. Balling and H. V. Westerhoff, NPJ Syst. Biol. Appl., 2020, 6, 34 Search PubMed.
- G.-Y. Liou and P. Storz, Free Radical Res., 2010, 44, 479–496 Search PubMed.
- M. I. Anik, N. Mahmud, A. A. Masud, M. I. Khan, M. N. Islam, S. Uddin and M. K. Hossain, ACS Appl. Bio Mater., 2022, 5, 4028–4054 Search PubMed.
- J. M. Lü, P. H. Lin, Q. Yao and C. Chen, J. Cell. Mol. Med., 2010, 14, 840–860 Search PubMed.
- L. W. Zheng, H. Chung and Y.-S. Kim, Food Res. Int., 2015, 75, 328–336 Search PubMed.
- K. U. Ingold and D. A. Pratt, Chem. Rev., 2014, 114, 9022–9046 Search PubMed.
- N. R. Perron and J. L. Brumaghim, Cell Biochem. Biophys., 2009, 53, 75–100 Search PubMed.
- J. Lu and A. Holmgren, Free Radicals Biol. Med., 2014, 66, 75–87 Search PubMed.
- M. D. Brand, C. Affourtit, T. C. Esteves, K. Green, A. J. Lambert, S. Miwa, J. L. Pakay and N. Parker, Free Radical Biol. Med., 2004, 37, 755–767 Search PubMed.
- R. Amorati and L. Valgimigli, Free Radical Res., 2015, 49, 633–649 Search PubMed.
- L. Valgimigli, A. Baschieri and R. Amorati, J. Mater. Chem. B, 2018, 6, 2036–2051 Search PubMed.
- A. Aranda, L. Sequedo, L. Tolosa, G. Quintas, E. Burello, J. V. Castell and L. Gombau, Toxicol. In Vitro, 2013, 27, 954–963 Search PubMed.
- D. Yu, Y. Zha, Z. Zhong, Y. Ruan, Z. Li, L. Sun and S. Hou, Sens. Actuators, B, 2021, 339, 129878 Search PubMed.
- A. Kessler, P. Huang, E. Blomberg and I. Odnevall, Chem. Res. Toxicol., 2023, 36, 1891–1900 Search PubMed.
- T. M. Potter, B. W. Neun and S. T. Stern, in Characterization of Nanoparticles Intended for Drug Delivery, ed. S. E. McNeil, Humana Press, Totowa, NJ, 2011, pp. 181–189 DOI:10.1007/978-1-60327-198-1_19.
- S. Sadeghian, G. A. Kojouri and A. Mohebbi, Biol. Trace Elem. Res., 2012, 146, 302–308 Search PubMed.
- W. Zhang, J. Gao, L. Lu, T. Bold, X. Li, S. Wang, Z. Chang, J. Chen, X. Kong, Y. Zheng, M. Zhang and J. Tang, NanoImpact, 2021, 23, 100338 Search PubMed.
- C. Jiang, C. Zhang, J. Song, X. Ji and W. Wang, Spectrochim. Acta, Part A, 2021, 250, 119316 Search PubMed.
- Y. Jv, B. Li and R. Cao, Chem. Commun., 2010, 46, 8017–8019 Search PubMed.
- W. He, X. Han, H. Jia, J. Cai, Y. Zhou and Z. Zheng, Sci. Rep., 2017, 7, 40103 Search PubMed.
- S.-B. He, L. Yang, X.-L. Lin, L.-M. Chen, H.-P. Peng, H.-H. Deng, X.-H. Xia and W. Chen, Talanta, 2020, 211, 120707 Search PubMed.
- G.-J. Cao, X. Jiang, H. Zhang, T. R. Croley and J.-J. Yin, RSC Adv., 2017, 7, 52210–52217 Search PubMed.
- S. V. Otari, M. Kumar, M. Z. Anwar, N. D. Thorat, S. K. S. Patel, D. Lee, J. H. Lee, J.-K. Lee, Y. C. Kang and L. Zhang, Sci. Rep., 2017, 7, 10980 Search PubMed.
- A. K. Keshari, R. Srivastava, P. Singh, V. B. Yadav and G. Nath, J. Ayurveda Integr. Med., 2020, 11, 37–44 Search PubMed.
- R. Prasad, J. Nanopart., 2014, 2014, 1–8 Search PubMed.
- P. V. AshaRani, G. Low Kah Mun, M. P. Hande and S. Valiyaveettil, ACS Nano, 2009, 3, 279–290 Search PubMed.
- A. Manke, L. Wang and Y. Rojanasakul, BioMed Res. Int., 2013, 2013, 942916 Search PubMed.
- K. D. Patel, A. K. Patel, P. Sawadkar, B. Singh and A. W. Perriman, in Multifunctional And Targeted Theranostic Nanomedicines: Formulation, Design And Applications, ed. K. Jain and N. K. Jain, Springer Nature, Singapore, 2023, pp. 97–117 DOI:10.1007/978-981-99-0538-6_5.
- A. Onodera, F. Nishiumi, K. Kakiguchi, A. Tanaka, N. Tanabe, A. Honma, K. Yayama, Y. Yoshioka, K. Nakahira, S. Yonemura, I. Yanagihara, Y. Tsutsumi and Y. Kawai, Toxicol. Rep., 2015, 2, 574–579 Search PubMed.
- C. Carlson, S. M. Hussain, A. M. Schrand, L. K. Braydich-Stolle, K. L. Hess, R. L. Jones and J. J. Schlager, J. Phys. Chem. B, 2008, 112, 13608–13619 Search PubMed.
- M. A. Elfaky, A. Sirwi, S. H. Ismail, H. H. Awad and S. S. Gad, Curr. Issues Mol. Biol., 2022, 44, 2923–2938 Search PubMed.
- B. Dąbrowska-Bouta, G. Sulkowski, W. Strużyński and L. Strużyńska, Neurotoxic. Res., 2019, 35, 495–504 Search PubMed.
- Y. Qing, L. Cheng, R. Li, G. Liu, Y. Zhang, X. Tang, J. Wang, H. Liu and Y. Qin, Int. J. Nanomed., 2018, 13, 3311–3327 Search PubMed.
- Y.-H. Hsin, C.-F. Chen, S. Huang, T.-S. Shih, P.-S. Lai and P. J. Chueh, Toxicol. Lett., 2008, 179, 130–139 Search PubMed.
- M. J. Piao, K. A. Kang, I. K. Lee, H. S. Kim, S. Kim, J. Y. Choi, J. Choi and J. W. Hyun, Toxicol. Lett., 2011, 201, 92–100 Search PubMed.
- D. He, A. M. Jones, S. Garg, A. N. Pham and T. D. Waite, J. Phys. Chem. C, 2011, 115, 5461–5468 Search PubMed.
- D. He, S. Garg and T. D. Waite, Langmuir, 2012, 28, 10266–10275 Search PubMed.
- E. Mendis, N. Rajapakse, H.-G. Byun and S.-K. Kim, Life Sci., 2005, 77, 2166–2178 Search PubMed.
- Q. Chang, L. Yan, M. Chen, H. He and J. Qu, Langmuir, 2007, 23, 11197–11199 Search PubMed.
- Y. Inoue, M. Hoshino, H. Takahashi, T. Noguchi, T. Murata, Y. Kanzaki, H. Hamashima and M. Sasatsu, J. Inorg. Biochem., 2002, 92, 37–42 Search PubMed.
- J. R. Hom, R. A. Quintanilla, D. L. Hoffman, K. L. de Mesy Bentley, J. D. Molkentin, S.-S. Sheu and G. A. Porter Jr., Dev. Cell, 2011, 21, 469–478 Search PubMed.
- A. Henglein, J. Phys. Chem., 1979, 83, 2209–2216 Search PubMed.
- A. Henglein and J. Lilie, J. Am. Chem. Soc., 1981, 103, 1059–1066 Search PubMed.
- L. Z. Flores-López, H. Espinoza-Gómez and R. Somanathan, J. Appl. Toxicol., 2019, 39, 16–26 Search PubMed.
- M. E. Hamdy, M. Del Carlo, H. A. Hussein, T. A. Salah, A. H. El-Deeb, M. M. Emara, G. Pezzoni and D. Compagnone, J. Nanobiotechnol., 2018, 16, 48 Search PubMed.
- M. U. Farooq, V. Novosad, E. A. Rozhkova, H. Wali, A. Ali, A. A. Fateh, P. B. Neogi, A. Neogi and Z. Wang, Sci. Rep., 2018, 8, 2907 Search PubMed.
- Y. Liu, W. Ma and J. Wang, Curr. Pharm. Des., 2018, 24, 2719–2728 Search PubMed.
- P. C. Nagajyothi, T. V. M. Sreekanth, C. O. Tettey, Y. I. Jun and S. H. Mook, Bioorg. Med. Chem. Lett., 2014, 24, 4298–4303 Search PubMed.
- P. Lau, N. Bidin, S. Islam, W. Shukri, N. Zakaria, N. Musa and G. Krishnan, Lasers Surg. Med., 2017, 49, 380–386 Search PubMed.
- M. G. Arafa, R. F. El-Kased and M. M. Elmazar, Sci. Rep., 2018, 8, 13674 Search PubMed.
- D. A. Giljohann, D. S. Seferos, W. L. Daniel, M. D. Massich, P. C. Patel and C. A. Mirkin, Angew. Chem., Int. Ed., 2010, 49, 3280–3294 Search PubMed.
- R. G. Saratale, I. Karuppusamy, G. D. Saratale, A. Pugazhendhi, G. Kumar, Y. Park, G. S. Ghodake, R. N. Bharagava, J. R. Banu and H. S. Shin, Colloids Surf., B, 2018, 170, 20–35 Search PubMed.
- N. A. Sutan, D. S. Manolescu, I. Fierascu, A. M. Neblea, C. Sutan, C. Ducu, L. C. Soare, D. Negrea, S. M. Avramescu and R. C. Fierascu, Mater. Sci. Eng., C, 2018, 93, 746–758 Search PubMed.
- M. I. Din, F. Arshad, Z. Hussain and M. Mukhtar, Nanoscale Res. Lett., 2017, 12, 638 Search PubMed.
- H. Joy Prabu and I. Johnson, Karbala Int. J. Mod. Sci., 2015, 1, 237–246 Search PubMed.
- K. B. Ayaz Ahmed, S. Subramanian, A. Sivasubramanian, G. Veerappan and A. Veerappan, Spectrochim. Acta, Part A, 2014, 130, 54–58 Search PubMed.
- N. Y. Stozhko, M. A. Bukharinova, E. I. Khamzina, A. V. Tarasov, M. B. Vidrevich and K. Z. Brainina, Nanomaterials, 2019, 9, 1655 Search PubMed.
- M. Hamelian, K. Varmira and H. Veisi, J. Photochem. Photobiol., B, 2018, 184, 71–79 Search PubMed.
- F. G. Milanezi, L. M. Meireles, M. M. de Christo Scherer, J. P. de Oliveira, A. R. da Silva, M. L. de Araujo, D. C. Endringer, M. Fronza, M. C. C. Guimarães and R. Scherer, Saudi Pharm. J., 2019, 27, 968–974 Search PubMed.
- L. M. Nieves, K. Mossburg, J. C. Hsu, A. D. A. Maidment and D. P. Cormode, Nanoscale, 2021, 13, 19306–19323 Search PubMed.
- Y. Mantri, B. Davidi, J. E. Lemaster, A. Hariri and J. V. Jokerst, Nanoscale, 2020, 12, 10511–10520 Search PubMed.
- N. Sarfraz and I. Khan, Chem. – Asian J., 2021, 16, 720–742 Search PubMed.
- I. B. Becerril-Castro, I. Calderon, N. Pazos-Perez, L. Guerrini, F. Schulz, N. Feliu, I. Chakraborty, V. Giannini, W. J. Parak and R. A. Alvarez-Puebla, Analysis Sensing, 2022, 2, e202200005 Search PubMed.
- J. K. Patra, G. Das, L. F. Fraceto, E. V. R. Campos, M. D. P. Rodriguez-Torres, L. S. Acosta-Torres, L. A. Diaz-Torres, R. Grillo, M. K. Swamy, S. Sharma, S. Habtemariam and H.-S. Shin, J. Nanobiotechnol., 2018, 16, 71 Search PubMed.
- G. Ajnai, A. Chiu, T. Kan, C.-C. Cheng, T.-H. Tsai and J. Chang, J. Exp. Clin. Med., 2014, 6, 172–178 Search PubMed.
- T. Xia, M. Kovochich, J. Brant, M. Hotze, J. Sempf, T. Oberley, C. Sioutas, J. I. Yeh, M. R. Wiesner and A. E. Nel, Nano Lett., 2006, 6, 1794–1807 Search PubMed.
- T. Xia, M. Kovochich, M. Liong, L. Madler, B. Gilbert, H. Shi, J. I. Yeh, J. I. Zink and A. E. Nel, ACS Nano, 2008, 2, 2121–2134 Search PubMed.
- A. Sasidharan and N. A. Monteiro-Riviere, Wiley Interdiscip. Rev.: Nanomed. Nanobiotechnol., 2015, 7, 779–796 Search PubMed.
- M. Holzinger, A. Le Goff and S. Cosnier, Front. Chem., 2014, 2, 63 Search PubMed.
- I. Willner, R. Baron and B. Willner, Biosens. Bioelectron., 2007, 22, 1841–1852 Search PubMed.
- K. Lee, H. Lee, K. W. Lee and T. G. Park, Biomaterials, 2011, 32, 2556–2565 Search PubMed.
- Y. Higashi, J. Mazumder, H. Yoshikawa, M. Saito and E. Tamiya, Anal. Chem., 2018, 90, 5773–5780 Search PubMed.
- H. Niu, R. Yuan, Y. Chai, L. Mao, Y. Yuan, Y. Zhuo, S. Yuan and X. Yang, Biosens. Bioelectron., 2011, 26, 3175–3180 Search PubMed.
- L. Mao, R. Yuan, Y. Chai, Y. Zhuo and Y. Xiang, Biosens. Bioelectron., 2011, 26, 4204–4208 Search PubMed.
- Y. Cui, Y. Zhao, Y. Tian, W. Zhang, X. Lü and X. Jiang, Biomaterials, 2012, 33, 2327–2333 Search PubMed.
- H. Lee and D. G. Lee, Colloids Surf., B, 2018, 167, 1–7 Search PubMed.
- Q. Zhang, V. M. Hitchins, A. M. Schrand, S. M. Hussain and P. L. Goering, Nanotoxicology, 2011, 5, 284–295 Search PubMed.
- D. Mateo, P. Morales, A. Ávalos and A. I. Haza, Toxicol. Mech. Methods, 2014, 24, 161–172 Search PubMed.
- A. Watanabe, M. Kajita, J. Kim, A. Kanayama, K. Takahashi, T. Mashino and Y. Miyamoto, Nanotechnology, 2009, 20, 455105 Search PubMed.
- K. Tahir, S. Nazir, A. Ahmad, B. Li, S. A. Ali Shah, A. U. Khan, G. M. Khan, Q. U. Khan, Z. U. Haq Khan and F. U. Khan, RSC Adv., 2016, 6, 85903–85916 Search PubMed.
- N. A. S. Ismail, J. X. Lee and F. Yusof, Antioxidants, 2022, 11, 986 Search PubMed.
- A. F. Elhusseiny and H. H. A. M. Hassan, Spectrochim. Acta, Part A, 2013, 103, 232–245 Search PubMed.
- A. J. Kora and L. Rastogi, Arabian J. Chem., 2018, 11, 1097–1106 Search PubMed.
- L. N. Lewis and N. Lewis, J. Am. Chem. Soc., 1986, 108, 7228–7231 Search PubMed.
- A. Roucoux, J. Schulz and H. Patin, Chem. Rev., 2002, 102, 3757–3778 Search PubMed.
- Y. Yoshihisa, Q.-L. Zhao, M. A. Hassan, Z.-L. Wei, M. Furuichi, Y. Miyamoto, T. Kondo and T. Shimizu, Free Radical Res., 2011, 45, 326–335 Search PubMed.
- Y. Yoshihisa, A. Honda, Q.-L. Zhao, T. Makino, R. Abe, K. Matsui, H. Shimizu, Y. Miyamoto, T. Kondo and T. Shimizu, Exp. Dermatol., 2010, 19, 1000–1006 Search PubMed.
- P. K. Gupta and L. Mishra, Nanoscale Adv., 2020, 2, 1774–1791 Search PubMed.
- C. Mu, K. E. Prosser, S. Harrypersad, G. A. MacNeil, R. Panchmatia, J. R. Thompson, S. Sinha, J. J. Warren and C. J. Walsby, Inorg. Chem., 2018, 57, 15247–15261 Search PubMed.
- S. K. Srivastava and M. Constanti, J. Nanopart. Res., 2012, 14, 831 Search PubMed.
- G. J. Cao, X. Jiang, H. Zhang, J. Zheng, T. R. Croley and J. J. Yin, J. Environ. Sci. Health, Part C: Environ. Carcinog. Ecotoxicol. Rev., 2017, 35, 223–238 Search PubMed.
- R. Boyd, Nat. Chem., 2011, 3, 570 Search PubMed.
- M. E. Weeks, J. Chem. Educ., 1932, 9, 474 Search PubMed.
- M. Kieliszek, I. Bano and H. Zare, Biol. Trace Elem. Res., 2022, 200, 971–987 Search PubMed.
- J. Zhao, R. Zhou, K. Hui, Y. Yang, Q. Zhang, Y. Ci, L. Shi, C. Xu, F. Huang and Y. Hu, Oncotarget, 2017, 8, 18832–18847 Search PubMed.
- B. Lipinski, Anticancer Agents Med. Chem., 2017, 17, 658–661 Search PubMed.
- F. Maiyo and M. Singh, Nanomedicine, 2017, 12, 1075–1089 Search PubMed.
- J. C. Avery and P. R. Hoffmann, Nutrients, 2018, 10, 1203 Search PubMed.
- R. A. Sunde and A. M. Raines, Adv. Nutr., 2011, 2, 138–150 Search PubMed.
- Y. Wang, W. Kong, L. Wang, J. Z. Zhang, Y. Li, X. Liu and Y. Li, Phys. Chem. Chem. Phys., 2019, 21, 1336–1343 Search PubMed.
- O. K. Chun, A. Floegel, S. J. Chung, C. E. Chung, W. O. Song and S. I. Koo, J. Nutr., 2010, 140, 317–324 Search PubMed.
- A. P. Kipp, D. Strohm, R. Brigelius-Flohe, L. Schomburg, A. Bechthold, E. Leschik-Bonnet and H. Heseker, J. Trace Elem. Med. Biol., 2015, 32, 195–199 Search PubMed.
- Z. Huang, A. H. Rose and P. R. Hoffmann, Antioxid. Redox Signaling, 2012, 16, 705–743 Search PubMed.
- H. Gill and G. Walker, Nutr. Diet., 2008, 65, S41–S47 Search PubMed.
- K. H. Winther, M. P. Rayman, S. J. Bonnema and L. Hegedüs, Nat. Rev. Endocrinol., 2020, 16, 165–176 Search PubMed.
- T. Nauser, S. Dockheer, R. Kissner and W. H. Koppenol, Biochemistry, 2006, 45, 6038–6043 Search PubMed.
- N. K. Kildahl, J. Chem. Educ., 1995, 72, 423 Search PubMed.
- S. Zhai, X. Hu, Y. Hu, B. Wu and D. Xing, Biomaterials, 2017, 121, 41–54 Search PubMed.
- J. Mu, L. Zhang, M. Zhao and Y. Wang, ACS Appl. Mater. Interfaces, 2014, 6, 7090–7098 Search PubMed.
- X. Miao, W. Cao, W. Zheng, J. Wang, X. Zhang, J. Gao, C. Yang, D. Kong, H. Xu, L. Wang and Z. Yang, Angew. Chem., Int. Ed., 2013, 52, 7781–7785 Search PubMed.
- O. Brodin, S. Eksborg, M. Wallenberg, C. Asker-Hagelberg, E. H. Larsen, D. Mohlkert, C. Lenneby-Helleday, H. Jacobsson, S. Linder, S. Misra and M. Björnstedt, Nutrients, 2015, 7, 4978–4994 Search PubMed.
- S. J. Knox, P. Jayachandran, C. A. Keeling, K. J. Stevens, N. Sandhu, S. L. Stamps-DeAnda, R. Savic, L. Shura, M. K. Buyyounouski and K. Grimes, Transl. Oncol., 2019, 12, 1525–1531 Search PubMed.
- H. K. Rooprai, I. Kyriazis, R. K. Nuttall, D. R. Edwards, D. Zicha, D. Aubyn, D. Davies, R. Gullan and G. J. Pilkington, Int. J. Oncol., 2007, 30, 1263–1271 Search PubMed.
- S. Y. Cui, H. Jin, S. J. Kim, A. P. Kumar and Y. I. Lee, J. Biochem., 2008, 143, 685–693 Search PubMed.
- H. Amani, R. Habibey, F. Shokri, S. J. Hajmiresmail, O. Akhavan, A. Mashaghi and H. Pazoki-Toroudi, Sci. Rep., 2019, 9, 6044 Search PubMed.
- E. H. Lo, T. Dalkara and M. A. Moskowitz, Nat. Rev. Neurosci., 2003, 4, 399–414 Search PubMed.
- S. Yang, J. Sun, P. He, X. Deng, Z. Wang, C. Hu, G. Ding and X. Xie, Chem. Mater., 2015, 27, 2004–2011 Search PubMed.
- H. W. Tan, H.-Y. Mo, A. T. Y. Lau and Y.-M. Xu, Int. J. Mol. Sci., 2018, 20, 75 Search PubMed.
- S. Khandelwal, M. Boylan, J. E. Spallholz and L. Gollahon, Int. J. Mol. Sci., 2018, 19(11), 3352 Search PubMed.
- C. M. Weekley, J. B. Aitken, S. Vogt, L. A. Finney, D. J. Paterson, M. D. de Jonge, D. L. Howard, P. K. Witting, I. F. Musgrave and H. H. Harris, J. Am. Chem. Soc., 2011, 133, 18272–18279 Search PubMed.
- Y. Wang, J. Wang, H. Hao, M. Cai, S. Wang, J. Ma, Y. Li, C. Mao and S. Zhang, ACS Nano, 2016, 10, 9927–9937 Search PubMed.
- T. Li, F. Li, W. Xiang, Y. Yi, Y. Chen, L. Cheng, Z. Liu and H. Xu, ACS Appl. Mater. Interfaces, 2016, 8, 22106–22112 Search PubMed.
- W. Zheng, T. Yin, Q. Chen, X. Qin, X. Huang, S. Zhao, T. Xu, L. Chen and J. Liu, Acta Biomater., 2016, 31, 197–210 Search PubMed.
- W. Zheng, C. Cao, Y. Liu, Q. Yu, C. Zheng, D. Sun, X. Ren and J. Liu, Acta Biomater., 2015, 11, 368–380 Search PubMed.
- J. Sun, C. Wei, Y. Liu, W. Xie, M. Xu, H. Zhou and J. Liu, Biomaterials, 2019, 197, 417–431 Search PubMed.
- M. S. Wason, J. Colon, S. Das, S. Seal, J. Turkson, J. Zhao and C. H. Baker, Nanomedicine, 2013, 9, 558–569 Search PubMed.
- J.-D. Cafun, K. O. Kvashnina, E. Casals, V. F. Puntes and P. Glatzel, ACS Nano, 2013, 7, 10726–10732 Search PubMed.
- N. Sutradhar, A. Sinhamahapatra, S. K. Pahari, P. Pal, H. C. Bajaj, I. Mukhopadhyay and A. B. Panda, J. Phys. Chem. C, 2011, 115, 12308–12316 Search PubMed.
- R. Dobrucka, Iran. J. Sci. Technol., Trans. A: Sci., 2018, 42, 547–555 Search PubMed.
- N. John Sushma, D. Prathyusha, G. Swathi, T. Madhavi, B. Deva Prasad Raju, K. Mallikarjuna and H.-S. Kim, Appl. Nanosci., 2016, 6, 437–444 Search PubMed.
- G. Kiranmai and A. R. Reddy, Toxicol. Ind. Health, 2013, 29, 897–903 Search PubMed.
- Y.-C. Kuo, S.-P. Lin, C.-W. Lin, Y.-C. Tsai, T.-S. Wu and I. L. Hsiao, ACS Appl. Nano Mater., 2023, 6, 19915–19925 Search PubMed.
- A. Sinha and S. K. Khare, Bioresour. Technol., 2011, 102, 4281–4284 Search PubMed.
- A. M. Allahverdiyev, E. S. Abamor, M. Bagirova and M. Rafailovich, Future Microbiol., 2011, 6, 933–940 Search PubMed.
- J. Matena, S. Petersen, M. Gieseke, A. Kampmann, M. Teske, M. Beyerbach, H. Escobar, H. Haferkamp, N.-C. Gellrich and I. Nolte, Int. J. Mol. Sci., 2015, 16, 7478 Search PubMed.
- O. Lucaciu, O. Soriţău, D. Gheban, D. R. Ciuca, O. Virtic, A. Vulpoi, N. Dirzu, R. Câmpian, G. Băciuţ, C. Popa, S. Simon, P. Berce, M. Băciuţ and B. Crisan, BMC Biotechnol., 2015, 15, 1–18 Search PubMed.
- D. Kuroda, M. Niinomi, M. Morinaga, Y. Kato and T. Yashiro, Mater. Sci. Eng., A, 1998, 243, 244–249 Search PubMed.
- B. J. Farrell, B. I. Prilutsky, J. M. Ritter, S. Kelley, K. Popat and M. Pitkin, J. Biomed. Mater. Res., Part A, 2014, 102, 1305–1315 Search PubMed.
- T. K. Ahn, D. H. Lee, T. S. Kim, G. C. Jang, S. Choi, J. B. Oh, G. Ye and S. Lee, Adv. Exp. Med. Biol., 2018, 1077, 355–368 Search PubMed.
- F. U. Rehman, C. Zhao, H. Jiang and X. Wang, Biomater. Sci., 2016, 4, 40–54 Search PubMed.
- M. Song, R. Zhang, Y. Dai, F. Gao, H. Chi, G. Lv, B. Chen and X. Wang, Biomaterials, 2006, 27, 4230–4238 Search PubMed.
- J. Wei, H. Shi, M. Zhou, D. Song, Y. Zhang, X. Pan, J. Zhou and T. Wang, Appl. Catal., A, 2015, 499, 101–108 Search PubMed.
- R. Cai, Y. Kubota, T. Shuin, H. Sakai, K. Hashimoto and A. Fujishima, Cancer Res., 1992, 52, 2346–2348 Search PubMed.
- N. Lagopati, E.-P. Tsilibary, P. Falaras, P. Papazafiri, E. A. Pavlatou, E. Kotsopoulou and P. Kitsiou, Int. J. Nanomed., 2014, 9, 3219–3230 Search PubMed.
- P. Boffetta, V. Gaborieau, L. Nadon, M. F. Parent, E. Weiderpass and J. Siemiatycki, Scand. J. Work, Environ. Health, 2001, 27, 227–232 Search PubMed.
- Y. Wang, H. Cui, J. Zhou, F. Li, J. Wang, M. Chen and Q. Liu, Environ. Sci. Pollut. Res., 2015, 22, 5519–5530 Search PubMed.
- K. Niska, K. Pyszka, C. Tukaj, M. Wozniak, M. W. Radomski and I. Inkielewicz-Stepniak, Int. J. Nanomed., 2015, 10, 1095–1107 Search PubMed.
- S. K. Verma, E. Jha, P. K. Panda, A. Thirumurugan, S. K. S. Parashar, S. Patro and M. Suar, ACS Omega, 2018, 3, 1244–1262 Search PubMed.
- C. Jin, Y. Tang, F. G. Yang, X. L. Li, S. Xu, X. Y. Fan, Y. Y. Huang and Y. J. Yang, Biol. Trace Elem. Res., 2011, 141, 3–15 Search PubMed.
- C. Angelé-Martínez, C. Goodman and J. Brumaghim, Metallomics, 2014, 6, 1358–1381 Search PubMed.
- X. Chen and S. S. Mao, Chem. Rev., 2007, 107, 2891–2959 Search PubMed.
- P.-C. Maness, S. Smolinski, D. M. Blake, Z. Huang, E. J. Wolfrum and W. A. Jacoby, Appl. Environ. Microbiol., 1999, 65, 4094–4098 Search PubMed.
- J. Cai, J. Peng, J. Feng, R. Li, P. Ren, X. Zang, Z. Wu, Y. Lu, L. Luo, Z. Hu, J. Wang, X. Dai, P. Zhao, J. Wang, M. Yan, J. Liu, R. Deng and D. Wang, Nat. Commun., 2023, 14, 3643 Search PubMed.
- J. Chu, Z. Kong, D. Lu, W. Zhang, X. Wang, Y. Yu, S. Li, X. Wang, S. Xiong and J. Ma, Mater. Lett., 2016, 166, 179–182 Search PubMed.
- J. Kysar and P. K. Sekhar, Appl. Nanosci., 2016, 6, 959–964 Search PubMed.
- A. Liu, M. Ichihara, I. Honma and H. Zhou, Electrochem. Commun., 2007, 9, 1766–1771 Search PubMed.
- R. Berenguer, M. O. Guerrero-Pérez, I. Guzmán, J. Rodríguez-Mirasol and T. Cordero, ACS Omega, 2017, 2, 7739–7745 Search PubMed.
- K. K. Dey, S. Jha, A. Kumar, G. Gupta, A. K. Srivastava and P. P. Ingole, Electrochim. Acta, 2019, 312, 89–99 Search PubMed.
- R. K. Sharma, P. Kumar and G. B. Reddy, J. Alloys Compd., 2015, 638, 289–297 Search PubMed.
- X. Zhou, G. Wu, J. Wu, H. Yang, J. Wang and G. Gao, Phys. Chem. Chem. Phys., 2014, 16, 3973–3982 Search PubMed.
- S. Pang, G. Li and Z. Zhang, Macromol. Rapid Commun., 2005, 26, 1262–1265 Search PubMed.
- J. Zhu, L. Cao, Y. Wu, Y. Gong, Z. Liu, H. E. Hoster, Y. Zhang, S. Zhang, S. Yang, Q. Yan, P. M. Ajayan and R. Vajtai, Nano Lett., 2013, 13, 5408–5413 Search PubMed.
- A. Makarevich, O. Makarevich, A. Ivanov, D. Sharovarov, A. Eliseev, V. Amelichev, O. Boytsova, A. Gorodetsky, M. Navarro-Cía and A. Kaul, CrystEngComm, 2020, 22, 2612–2620 Search PubMed.
- A. Pan, H. B. Wu, L. Yu, T. Zhu and X. W. Lou, ACS Appl. Mater. Interfaces, 2012, 4, 3874–3879 Search PubMed.
- F. Natalio, R. André, A. F. Hartog, B. Stoll, K. P. Jochum, R. Wever and W. Tremel, Nat. Nanotechnol., 2012, 7, 530–535 Search PubMed.
- A. A. Vernekar, D. Sinha, S. Srivastava, P. U. Paramasivam, P. D’Silva and G. Mugesh, Nat. Commun., 2014, 5, 5301 Search PubMed.
- S. Das, A. Roy, A. K. Barui, M. M. A. Alabbasi, M. Kuncha, R. Sistla, B. Sreedhar and C. R. Patra, Nanoscale, 2020, 12, 7604–7621 Search PubMed.
- B. L. Zhu, Q. Qiherima, L. Lv and X. J. Wang, Key Eng. Mater., 2012, 492, 264–267 Search PubMed.
- Y. Du and Y. Zhang, J. Exp. Nanosci., 2014, 9, 120–125 Search PubMed.
- S. Rong, P. Zhang, F. Liu and Y. Yang, ACS Catal., 2018, 8, 3435–3446 Search PubMed.
- T. Soejima, K. Nishizawa and R. Isoda, J. Colloid Interface Sci., 2018, 510, 272–279 Search PubMed.
- V. Kumar, K. Singh, S. Panwar and S. K. Mehta, Int. Nano Lett., 2017, 7, 123–131 Search PubMed.
- J. Shin, R. M. Anisur, M. K. Ko, G. H. Im, J. H. Lee and I. S. Lee, Angew. Chem., Int. Ed., 2009, 48, 321–324 Search PubMed.
- Y. Chen, H. Chen, S. Zhang, F. Chen, S. Sun, Q. He, M. Ma, X. Wang, H. Wu, L. Zhang, L. Zhang and J. Shi, Biomaterials, 2012, 33, 2388–2398 Search PubMed.
- H. Zhang, F. Xu, J. Xue, S. Chen, J. Wang and Y. Yang, Sci. Rep., 2020, 10, 6067 Search PubMed.
- S. Fairose, S. Ernest and Ashna, Mater. Today: Proc., 2017, 4, 12085–12093 Search PubMed.
- Y. Zhang, L. Chen, R. Sun, R. Lv, T. Du, Y. Li, X. Zhang, R. Sheng and Y. Qi, ACS Biomater. Sci. Eng., 2022, 8, 638–648 Search PubMed.
- J. Kim, H. Y. Kim, S. Y. Song, S.-H. Go, H. S. Sohn, S. Baik, M. Soh, K. Kim, D. Kim, H.-C. Kim, N. Lee, B.-S. Kim and T. Hyeon, ACS Nano, 2019, 13, 3206–3217 Search PubMed.
- Y. Wu, Z. Zhou, M. Zhang, S. Li, M. Sun and Z. Song, J. Nanobiotechnol., 2023, 21, 432 Search PubMed.
- F. Qian, Z. Huang, W. Liu, Y. Liu and X. He, J. Biomed. Mater. Res., Part A, 2023, 111, 1678–1691 Search PubMed.
- S. Alarifi, D. Ali and S. Alkahtani, BioMed Res. Int., 2017, 2017, 5478790 Search PubMed.
- N. Singh, M. Geethika, S. M. Eswarappa and G. Mugesh, Chem. – Eur. J., 2018, 24, 8393–8403 Search PubMed.
- N. Singh, M. A. Savanur, S. Srivastava, P. D'Silva and G. Mugesh, Nanoscale, 2019, 11, 3855–3863 Search PubMed.
- M. H. Tootoonchi, M. Hashempour, P. L. Blackwelder and C. A. Fraker, Acta Biomater., 2017, 59, 327–337 Search PubMed.
- C. R. Gordijo, K. Koulajian, A. J. Shuhendler, L. D. Bonifacio, H. Y. Huang, S. Chiang, G. A. Ozin, A. Giacca and X. Y. Wu, Adv. Funct. Mater., 2011, 21, 73–82 Search PubMed.
- C. R. Gordijo, A. J. Shuhendler and X. Y. Wu, Adv. Funct. Mater., 2010, 20, 1404–1412 Search PubMed.
- P. Prasad, C. R. Gordijo, A. Z. Abbasi, A. Maeda, A. Ip, A. M. Rauth, R. S. DaCosta and X. Y. Wu, ACS Nano, 2014, 8, 3202–3212 Search PubMed.
- I. Rabani, J. Yoo, H.-S. Kim, D. V. Lam, S. Hussain, K. Karuppasamy and Y.-S. Seo, Nanoscale, 2021, 13, 355–370 Search PubMed.
- R. Shanmuganathan, S. Sathiyavimal, Q. Hoang Le, M. M. Al-Ansari, L. A. Al-Humaid, G. K. Jhanani, J. Lee and S. Barathi, Environ. Res., 2023, 236, 116747 Search PubMed.
- D. Das and B. J. Saikia, Chem. Phys. Impact, 2023, 6, 100137 Search PubMed.
- L. Díez-Tercero, L. M. Delgado, E. Bosch-Rué and R. A. Perez, Sci. Rep., 2021, 11, 11707 Search PubMed.
- N. Mandakhbayar, Y. Ji, A. El-Fiqi, K. D. Patel, D. S. Yoon, K. Dashnyam, O. Bayaraa, G. Jin, K. Tsogtbaatar, T.-H. Kim, J.-H. Lee and H.-W. Kim, Bioact. Mater., 2024, 31, 298–311 Search PubMed.
- S. E. Kim, L. Zhang, K. Ma, M. Riegman, F. Chen, I. Ingold, M. Conrad, M. Z. Turker, M. Gao, X. Jiang, S. Monette, M. Pauliah, M. Gonen, P. Zanzonico, T. Quinn, U. Wiesner, M. S. Bradbury and M. Overholtzer, Nat. Nanotechnol., 2016, 11, 977–985 Search PubMed.
- L. Jiang, N. Kon, T. Li, S. J. Wang, T. Su, H. Hibshoosh, R. Baer and W. Gu, Nature, 2015, 520, 57–62 Search PubMed.
- C. Luo, Y. Li, L. Yang, X. Wang, J. Long and J. Liu, Arch. Toxicol., 2015, 89, 357–369 Search PubMed.
- Y. Ichikawa, M. Ghanefar, M. Bayeva, R. Wu, A. Khechaduri, S. V. Naga Prasad, R. K. Mutharasan, T. J. Naik and H. Ardehali, J. Clin. Invest., 2014, 124, 617–630 Search PubMed.
- L. S. Lin, J. Song, L. Song, K. Ke, Y. Liu, Z. Zhou, Z. Shen, J. Li, Z. Yang, W. Tang, G. Niu, H. H. Yang and X. Chen, Angew. Chem., Int. Ed., 2018, 57, 4902–4906 Search PubMed.
- S. Niu, L. K. Zhang, L. Zhang, S. Zhuang, X. Zhan, W. Y. Chen, S. Du, L. Yin, R. You, C. H. Li and Y. Q. Guan, Theranostics, 2017, 7, 344–356 Search PubMed.
- B. Torres-Herrero, I. Armenia, M. Alleva, L. Asín, S. Correa, C. Ortiz, Y. Fernández-Afonso, L. Gutiérrez, J. M. de la Fuente, L. Betancor and V. Grazú, ACS Nano, 2023, 17, 12358–12373 Search PubMed.
- Y. Zhang, Y. Wang, Q. Zhou, X. Chen, W. Jiao, G. Li, M. Peng, X. Liu, Y. He and H. Fan, ACS Appl. Mater. Interfaces, 2021, 13, 52395–52405 Search PubMed.
- Y. Hao, Z. Dong, M. Chen, Y. Chao, Z. Liu, L. Feng, Y. Hao, Z. L. Dong, M. C. Chen, Y. Chao, Z. Liu and L. Z. Feng, Biomaterials, 2020, 228, 119568 Search PubMed.
- A. K. Hauser, M. I. Mitov, E. F. Daley, R. C. McGarry, K. W. Anderson and J. Z. Hilt, Biomaterials, 2016, 105, 127–135 Search PubMed.
- M. I. Khan, A. Mohammad, G. Patil, S. A. H. Naqvi, L. K. S. Chauhan and I. Ahmad, Biomaterials, 2012, 33, 1477–1488 Search PubMed.
- J. Liu, L. Wang, J. Cao, Y. Huang, Y. Lin, X. Wu, Z. Wang, F. Zhang, X. Xu and G. Liu, Nanoscale, 2014, 6, 9025–9033 Search PubMed.
- E.-J. Park, D.-H. Choi, Y. Kim, E.-W. Lee, J. Song, M.-H. Cho, J.-H. Kim and S.-W. Kim, Toxicol. In Vitro, 2014, 28, 1402–1412 Search PubMed.
- H. Y. Tan, N. Wang, S. Li, M. Hong, X. Wang and Y. Feng, Oxid. Med. Cell. Longevity, 2016, 2016, 2795090 Search PubMed.
- J. Sarkar, N. Chakraborty, A. Chatterjee, A. Bhattacharjee, D. Dasgupta and K. Acharya, Nanomaterials, 2020, 10(2), 312 Search PubMed.
- S. Sukumar, A. Rudrasenan and D. Padmanabhan Nambiar, ACS Omega, 2020, 5, 1040–1051 Search PubMed.
- A. Ananth, S. Dharaneedharan, M.-S. Heo and Y. S. Mok, Chem. Eng. J., 2015, 262, 179–188 Search PubMed.
- M. Nasrollahzadeh, M. Maham and S. Mohammad Sajadi, J. Colloid Interface Sci., 2015, 455, 245–253 Search PubMed.
- D. Rehana, D. Mahendiran, R. S. Kumar and A. K. Rahiman, Biomed. Pharmacother., 2017, 89, 1067–1077 Search PubMed.
- C. Angelé-Martínez, K. V. T. Nguyen, F. S. Ameer, J. N. Anker and J. L. Brumaghim, Nanotoxicology, 2017, 11, 278–288 Search PubMed.
- A. M. Studer, L. K. Limbach, L. Van Duc, F. Krumeich, E. K. Athanassiou, L. C. Gerber, H. Moch and W. J. Stark, Toxicol. Lett., 2010, 197, 169–174 Search PubMed.
- H. L. Karlsson, P. Cronholm, J. Gustafsson and L. Möller, Chem. Res. Toxicol., 2008, 21, 1726–1732 Search PubMed.
- J. P. Kehrer, Toxicology, 2000, 149, 43–50 Search PubMed.
- H. Ashrafizadeh, S. R. Abtahi and A. A. Oroojan, Diabetes Metab. Syndr., 2020, 14, 443–445 Search PubMed.
- B. Stieberova, M. Zilka, M. Ticha, F. Freiberg, P. Caramazana-González, J. McKechnie and E. Lester, ACS Sustainable Chem. Eng., 2017, 5, 2493–2500 Search PubMed.
- S. Y. Kim, S. H. Jeong, E. Y. Lee, Y.-H. Park, H. C. Bae, Y. S. Jang, E. H. Maeng, M.-K. Kim and S. W. Son, J. Toxicol. Environ. Health Sci., 2011, 3, 258–261 Search PubMed.
- C. García-Gómez, A. Obrador, D. González, M. Babín and M. D. Fernández, Sci. Total Environ., 2017, 589, 11–24 Search PubMed.
- Y. K. Mishra and R. Adelung, Mater. Today, 2018, 21, 631–651 Search PubMed.
- M. Martínez-Carmona, Y. Gun’ko and M. Vallet-Regí, Nanomaterials, 2018, 8, 268 Search PubMed.
- J. W. Rasmussen, E. Martinez, P. Louka and D. G. Wingett, Expert Opin. Drug Delivery, 2010, 7, 1063–1077 Search PubMed.
- R. Lahri, M. Rahman, M. Wright, P. Kosmas and M. Thanou, Med. Phys., 2018, 45, 3820–3830 Search PubMed.
- P. Ruenraroengsak, D. Kiryushko, I. G. Theodorou, M. M. Klosowski, E. R. Taylor, T. Niriella, C. Palmieri, E. Yagüe, M. P. Ryan, R. C. Coombes, F. Xie and A. E. Porter, Nanoscale, 2019, 11, 12858–12870 Search PubMed.
- J. Wang, J. S. Lee, D. Kim and L. Zhu, ACS Appl. Mater. Interfaces, 2017, 9, 39971–39984 Search PubMed.
- S. Chaudhary, A. Umar, K. K. Bhasin and S. Baskoutas, Materials, 2018, 11(2), 287 Search PubMed.
- J. Singh, T. Dutta, K.-H. Kim, M. Rawat, P. Samddar and P. Kumar, J. Nanobiotechnol., 2018, 16, 84 Search PubMed.
- J. A. Hernandez-Viezcas, H. Castillo-Michel, A. D. Servin, J. R. Peralta-Videa and J. L. Gardea-Torresdey, Chem. Eng. J., 2011, 170, 346–352 Search PubMed.
- G. Chichiriccò and A. Poma, Nanomaterials, 2015, 5(2), 851–873 Search PubMed.
- P. C. Nagajyothi, S. J. Cha, I. J. Yang, T. V. M. Sreekanth, K. J. Kim and H. M. Shin, J. Photochem. Photobiol., B, 2015, 146, 10–17 Search PubMed.
- M. Shoeb, B. R. Singh, J. A. Khan, W. Khan, B. N. Singh, H. B. Singh and A. H. Naqvi, Adv. Nat. Sci., 2013, 4, 035015 Search PubMed.
- J.-H. Li, X.-R. Liu, Y. Zhang, F.-F. Tian, G.-Y. Zhao, Q.-L.-Y. Yu, F.-L. Jiang and Y. Liu, Toxicol. Res., 2012, 1, 137–144 Search PubMed.
- W. M. U. Daniels, J. Hendricks, R. Salie and S. J. van Rensburg, Metab. Brain Dis., 2004, 19, 79–88 Search PubMed.
- X. Xu, D. Chen, Z. Yi, M. Jiang, L. Wang, Z. Zhou, X. Fan, Y. Wang and D. Hui, Langmuir, 2013, 29, 5573–5580 Search PubMed.
- J. Gupta and D. Bahadur, ACS Omega, 2018, 3, 2956–2965 Search PubMed.
- M. Ahrén, L. Selegård, A. Klasson, F. Söderlind, N. Abrikossova, C. Skoglund, T. Bengtsson, M. Engström, P.-O. Käll and K. Uvdal, Langmuir, 2010, 26, 5753–5762 Search PubMed.
- S. J. Seo, S. M. Han, J. H. Cho, K. Hyodo, A. Zaboronok, H. You, K. Peach, M. A. Hill and J. K. Kim, Radiat. Environ. Biophys., 2015, 54, 423–431 Search PubMed.
- D. J. Todd and J. Kay, Curr. Rheumatol. Rep., 2008, 10, 195–204 Search PubMed.
- P. Sharma, S. Brown, G. Walter, S. Santra and B. Moudgil, Adv. Colloid Interface Sci., 2006, 123–126, 471–485 Search PubMed.
- A. Trovarelli and P. Fornasiero, Catalysis by Ceria and Related Materials, 2013 DOI:10.1142/9781848169647_fmatter.
- J. M. Dowding, S. Das, A. Kumar, T. Dosani, R. McCormack, A. Gupta, T. X. T. Sayle, D. C. Sayle, L. von Kalm, S. Seal and W. T. Self, ACS Nano, 2013, 7, 4855–4868 Search PubMed.
- I. Celardo, J. Z. Pedersen, E. Traversa and L. Ghibelli, Nanoscale, 2011, 3, 1411–1420 Search PubMed.
- M. S. Lord, J. F. Berret, S. Singh, A. Vinu and A. S. Karakoti, Small, 2021, 17, 2102342 Search PubMed.
- F. Corsi, F. Caputo, E. Traversa and L. Ghibelli, Front. Oncol., 2018, 8, 309 Search PubMed.
- D. Damatov and J. M. Mayer, Chem. Commun., 2016, 52, 10281–10284 Search PubMed.
- B. C. Nelson, M. E. Johnson, M. L. Walker, K. R. Riley and C. M. Sims, Antioxidants, 2016, 5(2), 15 Search PubMed.
- J. M. Dowding, S. Seal and W. T. Self, Drug Delivery Transl. Res., 2013, 3, 375–379 Search PubMed.
- W. He, Y. Liu, W. G. Wamer and J.-J. Yin, J. Food Drug Anal., 2014, 22, 49–63 Search PubMed.
- Y. Xue, Q. Luan, D. Yang, X. Yao and K. Zhou, J. Phys. Chem. C, 2011, 115, 4433–4438 Search PubMed.
- V. Patel, M. Singh, E. L. H. Mayes, A. Martinez, V. Shutthanandan, V. Bansal, S. Singh and A. S. Karakoti, Chem. Commun., 2018, 54, 13973–13976 Search PubMed.
- A. Srinivas, P. J. Rao, G. Selvam, P. B. Murthy and P. N. Reddy, Toxicol. Lett., 2011, 205, 105–115 Search PubMed.
- A. Filippi, F. Liu, J. Wilson, S. Lelieveld, K. Korschelt, T. Wang, Y. Wang, T. Reich, U. Pöschl, W. Tremel and H. Tong, RSC Adv., 2019, 9, 11077–11081 Search PubMed.
- C. Mahapatra, R. K. Singh, J.-H. Lee, J. Jung, J. K. Hyun and H.-W. Kim, Acta Biomater., 2017, 50, 142–153 Search PubMed.
- F. Corsi, F. Caputo, E. Traversa and L. Ghibelli, Front. Oncol., 2018, 8, 309 Search PubMed.
- I. Celardo, M. De Nicola, C. Mandoli, J. Z. Pedersen, E. Traversa and L. Ghibelli, ACS Nano, 2011, 5, 4537–4549 Search PubMed.
- F. Caputo, M. De Nicola, A. Sienkiewicz, A. Giovanetti, I. Bejarano, S. Licoccia, E. Traversa and L. Ghibelli, Nanoscale, 2015, 7, 15643–15656 Search PubMed.
- S.-H. Shin, O. Purevdorj, O. Castano, J. A. Planell and H.-W. Kim, J. Tissue Eng., 2012, 3, 2041731412443530 Search PubMed.
- A. Thill, O. Zeyons, O. Spalla, F. Chauvat, J. Rose, M. Auffan and A. M. Flank, Environ. Sci. Technol., 2006, 40, 6151–6156 Search PubMed.
- M. Culcasi, L. Benameur, A. Mercier, C. Lucchesi, H. Rahmouni, A. Asteian, G. Casano, A. Botta, H. Kovacic and S. Pietri, Chem. – Biol. Interact., 2012, 199, 161–176 Search PubMed.
- L. De Marzi, A. Monaco, J. De Lapuente, D. Ramos, M. Borras, M. Di Gioacchino, S. Santucci and A. Poma, Int. J. Mol. Sci., 2013, 14, 3065–3077 Search PubMed.
- G. Pota, B. Silvestri, G. Vitiello, N. Gallucci, R. Di Girolamo, S. Scialla, M. G. Raucci, L. Ambrosio, M. Di Napoli, A. Zanfardino, M. Varcamonti, A. Pezzella and G. Luciani, Biomater. Adv., 2023, 153, 213558 Search PubMed.
- K. D. Patel, A. K. Patel, A. G. Kurian, R. K. Singh and H.-W. Kim, in Nanotheranostics for Treatment and Diagnosis of Infectious Diseases, ed. K. Jain and J. Ahmad, Academic Press, 2022, pp. 319–352 DOI:10.1016/B978-0-323-91201-3.00011-6.
- X. Yuan, X. Zhang, L. Sun, Y. Wei and X. Wei, Part. Fibre Toxicol., 2019, 16, 18 Search PubMed.
- J. Meng, X. Li, C. Wang, H. Guo, J. Liu and H. Xu, ACS Appl. Mater. Interfaces, 2015, 7, 3180–3188 Search PubMed.
- E. J. Comparetti, V. d A. Pedrosa and R. Kaneno, Bioconjugate Chem., 2018, 29, 709–718 Search PubMed.
- B. D. Holt, P. A. Short, A. D. Rape, Y.-L. Wang, M. F. Islam and K. N. Dahl, ACS Nano, 2010, 4, 4872–4878 Search PubMed.
- B. D. Holt, H. Shams, T. A. Horst, S. Basu, A. D. Rape, Y.-L. Wang, G. K. Rohde, M. R. K. Mofrad, M. F. Islam and K. N. Dahl, J. Funct. Biomater., 2012, 3, 398–417 Search PubMed.
- B. D. Holt, K. N. Dahl and M. F. Islam, Small, 2011, 7, 2348–2355 Search PubMed.
- M. R. McDevitt, D. Chattopadhyay, B. J. Kappel, J. S. Jaggi, S. R. Schiffman, C. Antczak, J. T. Njardarson, R. Brentjens and D. A. Scheinberg, J. Nucl. Med., 2007, 48, 1180–1189 Search PubMed.
- B. L. Allen, P. D. Kichambare, P. Gou, I. I. Vlasova, A. A. Kapralov, N. Konduru, V. E. Kagan and A. Star, Nano Lett., 2008, 8, 3899–3903 Search PubMed.
- V. E. Kagan, N. V. Konduru, W. Feng, B. L. Allen, J. Conroy, Y. Volkov, I. I. Vlasova, N. A. Belikova, N. Yanamala, A. Kapralov, Y. Y. Tyurina, J. Shi, E. R. Kisin, A. R. Murray, J. Franks, D. Stolz, P. Gou, J. Klein-Seetharaman, B. Fadeel, A. Star and A. A. Shvedova, Nat. Nanotechnol., 2010, 5, 354–359 Search PubMed.
- F. T. Andón, A. A. Kapralov, N. Yanamala, W. Feng, A. Baygan, B. J. Chambers, K. Hultenby, F. Ye, M. S. Toprak, B. D. Brandner, A. Fornara, J. Klein-Seetharaman, G. P. Kotchey, A. Star, A. A. Shvedova, B. Fadeel and V. E. Kagan, Small, 2013, 9, 2721–2729 Search PubMed.
- K. Bhattacharya, R. El-Sayed, F. T. Andón, S. P. Mukherjee, J. Gregory, H. Li, Y. Zhao, W. Seo, A. Fornara, B. Brandner, M. S. Toprak, K. Leifer, A. Star and B. Fadeel, Carbon, 2015, 91, 506–517 Search PubMed.
- M. Yang and M. Zhang, Front. Mater., 2019, 6, 225 Search PubMed.
- B. L. Allen, G. P. Kotchey, Y. Chen, N. V. K. Yanamala, J. Klein-Seetharaman, V. E. Kagan and A. Star, J. Am. Chem. Soc., 2009, 131, 17194–17205 Search PubMed.
- M. Zhang, M. Yang, H. Nakajima, M. Yudasaka, S. Iijima and T. Okazaki, ACS Appl. Nano Mater., 2019, 2, 4293–4301 Search PubMed.
- J. Ju, S. Regmi, A. Fu, S. Lim and Q. Liu, J. Biophotonics, 2019, 12, e201800367 Search PubMed.
- Z. Jin, N. Dridi, G. Palui, V. Palomo, J. V. Jokerst, P. E. Dawson, Q.-X. A. Sang and H. Mattoussi, Anal. Chem., 2023, 95, 2713–2722 Search PubMed.
- I. L. Christensen, Y.-P. Sun and P. Juzenas, J. Biomed. Nanotechnol., 2011, 7, 667–676 Search PubMed.
- V. Ruiz, L. Yate, I. García, G. Cabanero and H.-J. Grande, Carbon, 2017, 116, 366–374 Search PubMed.
- L. Nilewski, K. Mendoza, A. S. Jalilov, V. Berka, G. Wu, W. K. A. Sikkema, A. Metzger, R. Ye, R. Zhang, D. X. Luong, T. Wang, E. McHugh, P. J. Derry, E. L. Samuel, T. A. Kent, A.-L. Tsai and J. M. Tour, ACS Appl. Mater. Interfaces, 2019, 11, 16815–16821 Search PubMed.
- Y. Li, S. Li, Y. Wang, J. Wang, H. Liu, X. Liu, L. Wang, X. Liu, W. Xue and N. Ma, Phys. Chem. Chem. Phys., 2017, 19, 11631–11638 Search PubMed.
- W. Stahl and H. Sies, Mol. Aspects Med., 2003, 24, 345–351 Search PubMed.
- K. Okuda, T. Hirota, M. Hirobe, T. Nagano, M. Mochizuki and T. Mashino, Fullerene Sci. Technol., 2000, 8, 127–142 Search PubMed.
- K. D. Patel, T.-H. Kim, N. Mandakhbayar, R. K. Singh, J.-H. Jang, J.-H. Lee and H.-W. Kim, Acta Biomater., 2020, 108, 97–110 Search PubMed.
- Y.-M. Li, K. D. Patel, Y.-K. Han, S.-M. Hong, Y.-X. Meng, H.-H. Lee, J. H. Park, J. C. Knowles, J. K. Hyun, J.-H. Lee and H.-W. Kim, J. Chem. Eng., 2023, 466, 143125 Search PubMed.
- I. Fenoglio, M. Tomatis, D. Lison, J. Muller, A. Fonseca, J. B. Nagy and B. Fubini, Free Radicals Biol. Med., 2006, 40, 1227–1233 Search PubMed.
- R. M. Lucente-Schultz, V. C. Moore, A. D. Leonard, B. K. Price, D. V. Kosynkin, M. Lu, R. Partha, J. L. Conyers and J. M. Tour, J. Am. Chem. Soc., 2009, 131, 3934–3941 Search PubMed.
- Y. Qiu, Z. Wang, A. C. E. Owens, I. Kulaots, Y. Chen, A. B. Kane and R. H. Hurt, Nanoscale, 2014, 6, 11744–11755 Search PubMed.
- G. de la Torre, G. Bottari and T. Torres, Adv. Energy Mater., 2017, 7, 1601700 Search PubMed.
- I. L. Christensen, Y. P. Sun and P. Juzenas, J. Biomed. Nanotechnol., 2011, 7, 667–676 Search PubMed.
- W. Zhang, J. Chavez, Z. Zeng, B. Bloom, A. Sheardy, Z. Ji, Z. Yin, D. H. Waldeck, Z. Jia and J. Wei, ACS Appl. Nano Mater., 2018, 1, 2699–2708 Search PubMed.
- G. Huang, Y. Lin, L. Zhang, Z. Yan, Y. Wang and Y. Liu, Sci. Rep., 2019, 9, 19651 Search PubMed.
- Y. Chong, C. Ge, G. Fang, X. Tian, X. Ma, T. Wen, W. G. Wamer, C. Chen, Z. Chai and J. J. Yin, ACS Nano, 2016, 10, 8690–8699 Search PubMed.
- S. S. Maktedar, G. Avashthi and M. Singh, RSC Adv., 2016, 6, 114264 Search PubMed.
- H.-Y. Chou, H.-M. D. Wang, C.-H. Kuo, P.-H. Lu, L. Wang, W. Kang and C.-L. Sun, ACS Omega, 2020, 5, 6588–6597 Search PubMed.
- G. Choe, S.-W. Kim, J. Park, J. Park, S. Kim, Y. S. Kim, Y. Ahn, D.-W. Jung, D. R. Williams and J. Y. Lee, Biomaterials, 2019, 225, 119513 Search PubMed.
- N. Baali, A. Khecha, A. Bensouici, G. Speranza and N. Hamdouni, C, 2019, 5, 75 Search PubMed.
- C. S. Sharma, S. Sarkar, A. Periyakaruppan, J. Barr, K. Wise, R. Thomas, B. L. Wilson and G. T. Ramesh, J. Nanosci. Nanotechnol., 2007, 7, 2466–2472 Search PubMed.
- S. K. Manna, S. Sarkar, J. Barr, K. Wise, E. V. Barrera, O. Jejelowo, A. C. Rice-Ficht and G. T. Ramesh, Nano Lett., 2005, 5, 1676–1684 Search PubMed.
- S. Alarifi and D. Ali, Int. J. Toxicol., 2015, 34, 258–265 Search PubMed.
- S. Rasras, H. Kalantari, M. Rezaei, M. A. Dehghani, L. Zeidooni, K. Alikarami, F. Dehghani and S. Alboghobeish, Toxicol. Ind. Health, 2019, 35, 497–506 Search PubMed.
- W.-C. Hou, S. BeigzadehMilani, C. T. Jafvert and R. G. Zepp, Environ. Sci. Technol., 2014, 48, 3875–3882 Search PubMed.
- A. V. Singh, K. K. Mehta, K. Worley, J. S. Dordick, R. S. Kane and L. Q. Wan, ACS Nano, 2014, 8, 2196–2205 Search PubMed.
- J. Zhang, H. Yang, G. Shen, P. Cheng, J. Zhang and S. Guo, Chem. Commun., 2010, 46, 1112–1114 Search PubMed.
- G. P. Kotchey, B. L. Allen, H. Vedala, N. Yanamala, A. A. Kapralov, Y. Y. Tyurina, J. Klein-Seetharaman, V. E. Kagan and A. Star, ACS Nano, 2011, 5, 2098–2108 Search PubMed.
- Y. Zhu, S. Murali, W. Cai, X. Li, J. W. Suk, J. R. Potts and R. S. Ruoff, Adv. Mater., 2010, 22, 3906–3924 Search PubMed.
- M. C. Duch, G. R. S. Budinger, Y. T. Liang, S. Soberanes, D. Urich, S. E. Chiarella, L. A. Campochiaro, A. Gonzalez, N. S. Chandel, M. C. Hersam and G. M. Mutlu, Nano Lett., 2011, 11, 5201–5207 Search PubMed.
- X. Yang, Y. Wang, X. Huang, Y. Ma, Y. Huang, R. Yang, H. Duan and Y. Chen, J. Mater. Chem., 2011, 21, 3448–3454 Search PubMed.
- M. Kalbacova, A. Broz, J. Kong and M. Kalbac, Carbon, 2010, 48, 4323–4329 Search PubMed.
- T. R. Nayak, H. Andersen, V. S. Makam, C. Khaw, S. Bae, X. Xu, P.-L. R. Ee, J.-H. Ahn, B. H. Hong, G. Pastorin and B. Özyilmaz, ACS Nano, 2011, 5, 4670–4678 Search PubMed.
- Z. Liu, J. T. Robinson, X. Sun and H. Dai, J. Am. Chem. Soc., 2008, 130, 10876–10877 Search PubMed.
- J. T. Robinson, S. M. Tabakman, Y. Liang, H. Wang, H. Sanchez Casalongue, D. Vinh and H. Dai, J. Am. Chem. Soc., 2011, 133, 6825–6831 Search PubMed.
- T. Parasassi, R. Brunelli, G. Costa, M. De Spirito, E. Krasnowska, T. Lundeberg, E. Pittaluga and F. Ursini, Sci. World J., 2010, 10, 1192–1202 Search PubMed.
- L. Feng, S. Zhang and Z. Liu, Nanoscale, 2011, 3, 1252–1257 Search PubMed.
- K. Yang, S. Zhang, G. Zhang, X. Sun, S. T. Lee and Z. Liu, Nano Lett., 2010, 10, 3318–3323 Search PubMed.
- H. Hong, K. Yang, Y. Zhang, J. W. Engle, L. Feng, Y. Yang, T. R. Nayak, S. Goel, J. Bean, C. P. Theuer, T. E. Barnhart, Z. Liu and W. Cai, ACS Nano, 2012, 6, 2361–2370 Search PubMed.
- Y. Wang, J. Lu, L. Tang, H. Chang and J. Li, Anal. Chem., 2009, 81, 9710–9715 Search PubMed.
- Z. Zhu, J. Qian, X. Zhao, W. Qin, R. Hu, H. Zhang, D. Li, Z. Xu, B. Z. Tang and S. He, ACS Nano, 2016, 10, 588–597 Search PubMed.
- B. Tian, C. Wang, S. Zhang, L. Feng and Z. Liu, ACS Nano, 2011, 5, 7000–7009 Search PubMed.
- H.-S. Hsieh and R. G. Zepp, Environ. Sci.: Nano, 2019, 6, 3734–3744 Search PubMed.
- X. Niu, J. Chen and J. Gao, Asian J. Pharm. Sci., 2019, 14, 480–496 Search PubMed.
- Y. Liu, G. Li, L. Huan and S. Cao, Nanoscale, 2024, 16, 504–526 Search PubMed.
- T. K. Nguyen, S. Aberoumand and D. V. Dao, Small, 2021, 17, 2101775 Search PubMed.
- X. Li, W. Li, Q. Liu, S. Chen, L. Wang, F. Gao, G. Shao, Y. Tian, Z. Lin and W. Yang, Adv. Funct. Mater., 2021, 31, 2008901 Search PubMed.
- F. Chen, G. Li, E. R. Zhao, J. Li, G. Hableel, J. E. Lemaster, Y. Bai, G. L. Sen and J. V. Jokerst, Biomaterials, 2018, 179, 60–70 Search PubMed.
- A. Borkowski, M. Szala, P. Kowalczyk, T. Cłapa, D. Narożna and M. Selwet, Chemosphere, 2015, 135, 233–239 Search PubMed.
- J. Pourchez, V. Forest, N. Boumahdi, D. Boudard, M. Tomatis, B. Fubini, N. Herlin-Boime, Y. Leconte, B. Guilhot, M. Cottier and P. Grosseau, J. Nanopart. Res., 2012, 14, 1143 Search PubMed.
- M. Schieber and N. S. Chandel, Curr. Biol., 2014, 24, R453–R462 Search PubMed.
- Z. Yi, H. I. Hussain, C. Feng, D. Sun, F. She, J. E. Rookes, D. M. Cahill and L. Kong, ACS Appl. Mater. Interfaces, 2015, 7, 9937–9946 Search PubMed.
- L. Chen, X. Zhou, W. Nie, Q. Zhang, W. Wang, Y. Zhang and C. He, ACS Appl. Mater. Interfaces, 2016, 8, 33829–33841 Search PubMed.
- Q. Zhao, H. Geng, Y. Wang, Y. Gao, J. Huang, Y. Wang, J. Zhang and S. Wang, ACS Appl. Mater. Interfaces, 2014, 6, 20290–20299 Search PubMed.
- R. Cheng, F. Feng, F. Meng, C. Deng, J. Feijen and Z. Zhong, J. Controlled Release, 2011, 152, 2–12 Search PubMed.
- S. Zanganeh, G. Hutter, R. Spitler, O. Lenkov, M. Mahmoudi, A. Shaw, J. S. Pajarinen, H. Nejadnik, S. Goodman, M. Moseley, L. M. Coussens and H. E. Daldrup-Link, Nat. Nanotechnol., 2016, 11, 986–994 Search PubMed.
- J.-W. Kim, C. Mahapatra, J.-Y. Hong, M. S. Kim, K. W. Leong, H.-W. Kim and J. K. Hyun, Adv. Sci., 2017, 4, 1700034 Search PubMed.
- C. Nathan and A. Cunningham-Bussel, Nat. Rev. Immunol., 2013, 13, 349–361 Search PubMed.
- T.-C. Cheong, E. P. Shin, E.-K. Kwon, J.-H. Choi, K.-K. Wang, P. Sharma, K. H. Choi, J.-M. Lim, H.-G. Kim, K. Oh, J.-H. Jeon, I. So, I.-G. Kim, M.-S. Choi, Y. K. Kim, S.-Y. Seong, Y.-R. Kim and N.-H. Cho, ACS Chem. Biol., 2015, 10, 757–765 Search PubMed.
- B. Zhu, Y. Li, Z. Lin, M. Zhao, T. Xu, C. Wang and N. Deng, Nanoscale Res. Lett., 2016, 11, 198 Search PubMed.
- R. Pal, B. Chakraborty, A. Nath, L. M. Singh, M. Ali, D. S. Rahman, S. K. Ghosh, A. Basu, S. Bhattacharya, R. Baral and M. Sengupta, Int. Immunopharmacol., 2016, 38, 332–341 Search PubMed.
- Y. Lu, Y. Yang, Z. Gu, J. Zhang, H. Song, G. Xiang and C. Yu, Biomaterials, 2018, 175, 82–92 Search PubMed.
- M. Schieber and N. S. Chandel, Curr. Biol., 2014, 24, R453–R462 Search PubMed.
- C. Gorrini, I. S. Harris and T. W. Mak, Nat. Rev. Drug Discovery, 2013, 12, 931–947 Search PubMed.
- G. Cui, H. Zhang, Q. Guo, S. Shan, S. Chen, C. Li, X. Yang, Z. Li, Y. Mu, H. Shao and Z. Du, Toxicol. Mech. Methods, 2020, 30, 646–655 Search PubMed.
- G. N. Joshi, A. M. Goetjen and D. A. Knecht, Mol. Biol. Cell, 2015, 26, 3150–3164 Search PubMed.
- Y.-J. Lin, C.-C. Yang, I.-T. Lee, W.-B. Wu, C.-C. Lin, L.-D. Hsiao and C.-M. Yang, Biomedicines, 2023, 11, 2628 Search PubMed.
- S. N. Petrache Voicu, D. Dinu, C. Sima, A. Hermenean, A. Ardelean, E. Codrici, M. S. Stan, O. Zărnescu and A. Dinischiotu, Int. J. Mol. Sci., 2015, 16, 29398–29416 Search PubMed.
- C. Marques, P. Maroni, L. Maurizi, O. Jordan and G. Borchard, Int. J. Biol. Macromol., 2024, 256, 128339 Search PubMed.
- S. Tenzer, D. Docter, J. Kuharev, A. Musyanovych, V. Fetz, R. Hecht, F. Schlenk, D. Fischer, K. Kiouptsi, C. Reinhardt, K. Landfester, H. Schild, M. Maskos, S. K. Knauer and R. H. Stauber, Nat. Nanotechnol., 2013, 8, 772–781 Search PubMed.
- Y.-M. Go and D. P. Jones, J. Biol. Chem., 2013, 288, 26512–26520 Search PubMed.
- R. Kumar, G. R. Pulikanti, K. R. Shankar, D. Rambabu, V. Mangili, L. R. Kumbam, P. S. Sagara, N. Nakka and M. Yogesh, in Metal Oxides for Biomedical and Biosensor Applications, ed. K. Mondal, Elsevier, 2022, pp. 205–231 DOI:10.1016/B978-0-12-823033-6.00007-7.
- R. K. Singh, K. D. Patel, K. W. Leong and H.-W. Kim, ACS Appl. Mater. Interfaces, 2017, 9, 10309–10337 Search PubMed.
- A. Luchini and G. Vitiello, Front. Chem., 2019, 7, 343 Search PubMed.
- S. F. Lim and R. H. Austin, in Applications of Nanoscience in Photomedicine, ed. M. R. Hamblin and P. Avci, Chandos Publishing, Oxford, 2015, pp. 377–391 DOI:10.1533/9781908818782.377.
- S. Wen, J. Zhou, K. Zheng, A. Bednarkiewicz, X. Liu and D. Jin, Nat. Commun., 2018, 9, 2415 Search PubMed.
- A. Priyam, N. M. Idris and Y. Zhang, J. Mater. Chem., 2012, 22, 960–965 Search PubMed.
- Y. He, S. Guo, L. Wu, P. Chen, L. Wang, Y. Liu and H. Ju, Biomaterials, 2019, 225, 119501 Search PubMed.
- W.-H. Chen, G.-F. Luo, W.-X. Qiu, Q. Lei, S. Hong, S.-B. Wang, D.-W. Zheng, C.-H. Zhu, X. Zeng, J. Feng, S.-X. Cheng and X.-Z. Zhang, Small, 2016, 12, 733–744 Search PubMed.
- L. Diebold and N. S. Chandel, Free Radicals Biol. Med., 2016, 100, 86–93 Search PubMed.
- S. Kumari, A. K. Badana, M. M. G, S. G and R. Malla, Biomarker Insights, 2018, 13, 1177271918755391 Search PubMed.
- A. D. Pandya, E. Jager, S. Bagheri Fam, A. Hocherl, A. Jager, V. Sincari, B. Nystrom, P. Stepanek, T. Skotland, K. Sandvig, M. Hruby and G. M. Maelandsmo, Int. J. Nanomed., 2019, 14, 6269–6285 Search PubMed.
- P. Sonkusre and S. S. Cameotra, J. Nanobiotechnol., 2017, 15, 43 Search PubMed.
- A. Khurana, S. Tekula, M. A. Saifi, P. Venkatesh and C. Godugu, Biomed. Pharmacother., 2019, 111, 802–812 Search PubMed.
- A. P. Bidkar, P. Sanpui and S. S. Ghosh, Nanomedicine, 2017, 12, 2641–2651 Search PubMed.
- C.-W. Chen, Y.-C. Chan, M. Hsiao and R.-S. Liu, ACS Appl. Mater. Interfaces, 2016, 8, 32108–32119 Search PubMed.
- H. Luo, F. Wang, Y. Bai, T. Chen and W. Zheng, Colloids Surf., B, 2012, 94, 304–308 Search PubMed.
- L. Kong, Q. Yuan, H. Zhu, Y. Li, Q. Guo, Q. Wang, X. Bi and X. Gao, Biomaterials, 2011, 32, 6515–6522 Search PubMed.
- Y. Zhuang, L. Li, L. Feng, S. Wang, H. Su, H. Liu, H. Liu and Y. Wu, Nanoscale, 2020, 12, 1389–1396 Search PubMed.
- L. Chan, L. He, B. Zhou, S. Guan, M. Bo, Y. Yang, Y. Liu, X. Liu, Y. Zhang, Q. Xie and T. Chen, Chem. – Asian J., 2017, 12, 3053–3060 Search PubMed.
- F. L. Heppner, R. M. Ransohoff and B. Becher, Nat. Rev. Neurosci., 2015, 16, 358–372 Search PubMed.
- F. Fanizza, M. Campanile, G. Forloni, C. Giordano and D. Albani, J. Tissue Eng., 2022, 13, 1–20, DOI:10.1177%2F20417314221095339.
- O. Devinsky, A. Vezzani, S. Najjar, N. C. De Lanerolle and M. A. Rogawski, Trends Neurosci., 2013, 36, 174–184 Search PubMed.
- D. Kempuraj, R. Thangavel, P. A. Natteru, G. P. Selvakumar, D. Saeed, H. Zahoor, S. Zaheer, S. S. Iyer and A. Zaheer, J. Neurol. Neurosurg. Spine, 2016, 1, 1003 Search PubMed.
- M. T. Lin and M. F. Beal, Nature, 2006, 443, 787–795 Search PubMed.
- S. Gandhi and A. Y. Abramov, Oxid. Med. Cell. Longevity, 2012, 2012, 428010 Search PubMed.
- E. Radi, P. Formichi, C. Battisti and A. Federico, J. Alzheimer's Dis., 2014, 42(Suppl 3), S125–S152 Search PubMed.
- A. Federico, E. Cardaioli, P. Da Pozzo, P. Formichi, G. N. Gallus and E. Radi, J. Neurol. Sci., 2012, 322, 254–262 Search PubMed.
- P. J. Landrigan, B. Sonawane, R. N. Butler, L. Trasande, R. Callan and D. Droller, Environ. Health Perspect., 2005, 113, 1230–1233 Search PubMed.
- L. M. Billings, S. Oddo, K. N. Green, J. L. McGaugh and F. M. LaFerla, Neuron, 2005, 45, 675–688 Search PubMed.
- E. D. Roberson, K. Scearce-Levie, J. J. Palop, F. Yan, I. H. Cheng, T. Wu, H. Gerstein, G.-Q. Yu and L. Mucke, Science, 2007, 316, 750–754 Search PubMed.
- T. Yin, L. Yang, Y. Liu, X. Zhou, J. Sun and J. Liu, Acta Biomater., 2015, 25, 172–183 Search PubMed.
- L. Yang, Q. Chen, Y. Liu, J. Zhang, D. Sun, Y. Zhou and J. Liu, J. Mater. Chem. B, 2014, 2, 1977–1987 Search PubMed.
- N. Huang, X. Chen, X. Zhu, M. Xu and J. Liu, Biomaterials, 2017, 141, 296–313 Search PubMed.
- S. Mukherjee, V. S. Madamsetty, D. Bhattacharya, S. Roy Chowdhury, M. K. Paul and A. Mukherjee, Adv. Funct. Mater., 2020, 30, 2003054 Search PubMed.
- G. H. Kim, J. E. Kim, S. J. Rhie and S. Yoon, Exp. Neurobiol., 2015, 24, 325–340 Search PubMed.
- H. E. Moon and S. H. Paek, Exp. Neurobiol., 2015, 24, 103–116 Search PubMed.
- J. Blesa, I. Trigo-Damas, A. Quiroga-Varela and V. R. Jackson-Lewis, Front. Neuroanat., 2015, 9, 91 Search PubMed.
- W. J. Szlachcic, P. M. Switonski, W. J. Krzyzosiak, M. Figlerowicz and M. Figiel, Dis. Models Mech., 2015, 8, 1047–1057 Search PubMed.
- G. Cenini, A. Lloret and R. Cascella, Oxid. Med. Cell. Longevity, 2019, 2019, 2105607 Search PubMed.
- S. Kausar, F. Wang and H. Cui, Cells, 2018, 7(12), 274 Search PubMed.
- A. Grzelak, M. Wojewodzka, S. Meczynska-Wielgosz, M. Zuberek, D. Wojciechowska and M. Kruszewski, Redox Biol., 2018, 15, 435–440 Search PubMed.
- A. Gupta, S. Das and S. Seal, Nanomedicine, 2014, 9, 2725–2728 Search PubMed.
- S. Naz, J. Beach, B. Heckert, T. Tummala, O. Pashchenko, T. Banerjee and S. Santra, Nanomedicine, 2017, 12, 545–553 Search PubMed.
- H. Zhou, Y. Gong, Y. Liu, A. Huang, X. Zhu, J. Liu, G. Yuan, L. Zhang, J.-A. Wei and J. Liu, Biomaterials, 2020, 237, 119822 Search PubMed.
- H. J. Kwon, D. Kim, K. Seo, Y. G. Kim, S. I. Han, T. Kang, M. Soh and T. Hyeon, Angew. Chem., Int. Ed., 2018, 57, 9408–9412 Search PubMed.
- L. Zhang, P.-F. Wei, Y.-H. Song, L. Dong, Y.-D. Wu, Z.-Y. Hao, S. Fan, S. Tai, J.-L. Meng, Y. Lu, J. Xue, C.-Z. Liang and L.-P. Wen, Biomaterials, 2019, 216, 119248 Search PubMed.
- X. Li, J. Tsibouklis, T. Weng, B. Zhang, G. Yin, G. Feng, Y. Cui, I. N. Savina, L. I. Mikhalovska, S. R. Sandeman, C. A. Howel and S. V. Mikhalovsky, J. Drug Targeting, 2017, 25, 17–28 Search PubMed.
- D. K. Sarko and C. E. McKinney, Front. Neurosci., 2017, 11, 82 Search PubMed.
- S. M. ElMorsy, D. A. Gutierrez, S. Valdez, J. Kumar, R. J. Aguilera, M. Noufal, H. Sarma, S. Chinnam and M. Narayan, J. Colloid Interface Sci., 2024, 670, 357–363 Search PubMed.
- R.-R. Lin, L.-L. Jin, Y.-Y. Xue, Z.-S. Zhang, H.-F. Huang, D.-F. Chen, Q. Liu, Z.-W. Mao, Z.-Y. Wu and Q.-Q. Tao, Adv. Sci., 2024, 11, 2306675 Search PubMed.
- H. Tan, Y. Huang, S. Dong, Z. Bai, C. Chen, X. Wu, M. Chao, H. Yan, S. Wang, D. Geng and F. Gao, Small, 2023, 19, 2303530 Search PubMed.
- X. Song, Q. Ding, W. Wei, J. Zhang, R. Sun, L. Yin, S. Liu and Y. Pu, Small, 2023, 19, 2206959 Search PubMed.
- R. Ruotolo, G. De Giorgio, I. Minato, M. G. Bianchi, O. Bussolati and N. Marmiroli, Nanomaterials, 2020, 10(2), 235 Search PubMed.
- W. DeCoteau, K. L. Heckman, A. Y. Estevez, K. J. Reed, W. Costanzo, D. Sandford, P. Studlack, J. Clauss, E. Nichols, J. Lipps, M. Parker, B. Hays-Erlichman, J. C. Leiter and J. S. Erlichman, Nanomedicine, 2016, 12, 2311–2320 Search PubMed.
- W. Cong, R. Bai, Y.-F. Li, L. Wang and C. Chen, ACS Appl. Mater. Interfaces, 2019, 11, 34725–34735 Search PubMed.
- A. M. Khan, B. Korzeniowska, V. Gorshkov, M. Tahir, H. Schrøder, L. Skytte, K. L. Rasmussen, S. Khandige, J. Møller-Jensen and F. Kjeldsen, Nanotoxicology, 2019, 13, 221–239 Search PubMed.
- H. J. Kwon, M.-Y. Cha, D. Kim, D. K. Kim, M. Soh, K. Shin, T. Hyeon and I. Mook-Jung, ACS Nano, 2016, 10, 2860–2870 Search PubMed.
- Z. Gao, Y. Nakanishi, S. Noda, H. Omachi, H. Shinohara, H. Kimura and Y. Nagasaki, J. Biomater. Sci., Polym. Ed., 2017, 28, 1036–1050 Search PubMed.
- Y. Shen, B. Cao, N. R. Snyder, K. M. Woeppel, J. R. Eles and X. T. Cui, J. Nanobiotechnol., 2018, 16, 13 Search PubMed.
- P. Boonruamkaew, P. Chonpathompikunlert, L. B. Vong, S. Sakaue, Y. Tomidokoro, K. Ishii, A. Tamaoka and Y. Nagasaki, Sci. Rep., 2017, 7, 3785 Search PubMed.
- L. Ling, Y. Jiang, Y. Liu, H. Li, A. Bari, R. Ullah and J. Xue, J. Photochem. Photobiol., B, 2019, 201, 111657 Search PubMed.
- A. V. Ivanov, B. Bartosch and M. G. Isaguliants, Oxid. Med. Cell. Longevity, 2017, 2017, 3496043 Search PubMed.
- U. Z. Paracha, K. Fatima, M. Alqahtani, A. Chaudhary, A. Abuzenadah, G. Damanhouri and I. Qadri, Virol. J., 2013, 10, 251 Search PubMed.
- S. Li, H. Li, X. Xu, P. E. Saw and L. Zhang, Theranostics, 2020, 10, 1262–1280 Search PubMed.
- N. Thakur, P. Manna and J. Das, J. Nanobiotechnol., 2019, 17, 84 Search PubMed.
- Y. Huang, M. Zhang, M. Jin, T. Ma, J. Guo, X. Zhai and Y. Du, Adv. Healthcare Mater., 2023, 12, 2300748 Search PubMed.
- M. G. Schappi, V. Jaquet, D. C. Belli and K. H. Krause, Semin. Immunopathol., 2008, 30, 255–271 Search PubMed.
- Q. Ren, S. Sun and X.-D. Zhang, Nano Res., 2021, 14, 2535–2557 Search PubMed.
- T. Kuijpers and R. Lutter, Cell. Mol. Life Sci., 2012, 69, 7–15 Search PubMed.
- K. S. Kim, D. Lee, C. G. Song and P. M. Kang, Nanomedicine, 2015, 10, 2709–2723 Search PubMed.
- T. Hemnani and M. S. Parihar, Indian J. Physiol. Pharmacol., 1998, 42, 440–452 Search PubMed.
- A. Chompoosor, K. Saha, P. S. Ghosh, D. J. Macarthy, O. R. Miranda, Z. J. Zhu, K. F. Arcaro and V. M. Rotello, Small, 2010, 6, 2246–2249 Search PubMed.
- U. S. Srinivas, B. W. Q. Tan, B. A. Vellayappan and A. D. Jeyasekharan, Redox Biol., 2019, 25, 101084 Search PubMed.
- A. Grzelak, M. Wojewódzka, S. Meczynska-Wielgosz, M. Zuberek, D. Wojciechowska and M. Kruszewski, Redox Biol., 2018, 15, 435–440 Search PubMed.
- P. Patel, K. Kansara, R. Singh, R. K. Shukla, S. Singh, A. Dhawan and A. Kumar, Int. J. Nanomed., 2018, 13, 39–41 Search PubMed.
- K. Mortezaee, M. Najafi, H. Samadian, H. Barabadi, A. Azarnezhad and A. Ahmadi, Chem.-Biol. Interact., 2019, 312, 108814 Search PubMed.
- Y. Li, S. Yu, Q. Wu, M. Tang, Y. Pu and D. Wang, J. Hazard. Mater., 2012, 219–220, 221–230 Search PubMed.
- K. R. Smith, L. R. Klei and A. Barchowsky, Am. J. Physiol.: Lung Cell. Mol. Physiol., 2001, 280, L442–L449 Search PubMed.
- S. J. Soenen, P. Rivera-Gil, J.-M. Montenegro, W. J. Parak, S. C. De Smedt and K. Braeckmans, Nano Today, 2011, 6, 446–465 Search PubMed.
- K. Bedard, H. Attar, J. Bonnefont, V. Jaquet, C. Borel, O. Plastre, M. J. Stasia, S. E. Antonarakis and K. H. Krause, Hum. Mutat., 2009, 30, 1123–1133 Search PubMed.
- J. A. Nogueira-Machado and M. M. Chaves, Expert Opin. Ther. Targets, 2008, 12, 871–882 Search PubMed.
- Y. Zhang, T. Peng, H. Zhu, X. Zheng, X. Zhang, N. Jiang, X. Cheng, X. Lai, A. Shunnar, M. Singh, N. Riordan, V. Bogin, N. Tong and W. P. Min, J. Transl. Med., 2010, 8, 133 Search PubMed.
- R. F. Loeser, Trans. Am. Clin. Climatol. Assoc., 2017, 128, 44–54 Search PubMed.
- Y. Henrotin, G. Deby-Dupont, C. Deby, M. De Bruyn, M. Lamy and P. Franchimont, Br. J. Rheumatol., 1993, 32, 562–567 Search PubMed.
- M. L. Tiku, J. B. Liesch and F. M. Robertson, J. Immunol., 1990, 145, 690–696 Search PubMed.
- Y. E. Henrotin, P. Bruckner and J. P. L. Pujol, Osteoarthritis Cartilage, 2003, 11, 747–755 Search PubMed.
- M. A. Altay, C. Erturk, A. Bilge, M. Yapti, A. Levent and N. Aksoy, Rheumatol. Int., 2015, 35, 1725–1731 Search PubMed.
- C. Erturk, M. A. Altay, S. Selek and A. Kocyigit, Scand. J. Clin. Lab. Invest., 2012, 72, 433–439 Search PubMed.
- C. M. Davies, F. Guilak, J. B. Weinberg and B. Fermor, Osteoarthritis Cartilage, 2008, 16, 624–630 Search PubMed.
- V. N.-S. Lucas José Sá da Fonseca, M. Oliveira Fonseca Goulart and L. Antas Rabelo, Oxid. Med. Cell. Longevity, 2019, 2019, 16 Search PubMed.
- R. Bordy, P. Totoson, C. Prati, C. Marie, D. Wendling and C. Demougeot, Nat. Rev. Rheumatol., 2018, 14, 404–420 Search PubMed.
- D. Chen, J. Shen, W. Zhao, T. Wang, L. Han, J. L. Hamilton and H.-J. Im, Bone Res., 2017, 5, 16044 Search PubMed.
- J. S. Lee, M. L. Cho, J. Y. Jhun, S. Y. Min, J. H. Ju, C. H. Yoon, J. K. Min, S. H. Park, H. Y. Kim and Y. G. Cho, J. Clin. Immunol., 2006, 26, 204–212 Search PubMed.
- D. Jawaheer, M. F. Seldin, C. I. Amos, W. V. Chen, R. Shigeta, C. Etzel, A. Damle, X. Xiao, D. Chen, R. F. Lum, J. Monteiro, M. Kern, L. A. Criswell, S. Albani, J. L. Nelson, D. O. Clegg, R. Pope, H. W. Schroeder, Jr., S. L. Bridges, Jr., D. S. Pisetsky, R. Ward, D. L. Kastner, R. L. Wilder, T. Pincus, L. F. Callahan, D. Flemming, M. H. Wener and P. K. Gregersen, Arthritis Rheum., 2003, 48, 906–916 Search PubMed.
- A. Mirshafiey and M. Mohsenzadegan, Iran. J. Allergy, Asthma Immunol., 2008, 7, 195–202 Search PubMed.
- M. Hultqvist, P. Olofsson, J. Holmberg, B. T. Bäckström, J. Tordsson and R. Holmdahl, Proc. Natl. Acad. Sci. U. S. A., 2004, 101, 12646–12651 Search PubMed.
- S. Mangialaio, H. Ji, A. S. Korganow, V. Kouskoff, C. Benoist and D. Mathis, Arthritis Rheum., 1999, 42, 2517–2523 Search PubMed.
- A. Corthay, A. S. Hansson and R. Holmdahl, Arthritis Rheum., 2000, 43, 844–851 Search PubMed.
- K. Kannan, R. A. Ortmann and D. Kimpel, Pathophysiology, 2005, 12, 167–181 Search PubMed.
- K. A. Gelderman, M. Hultqvist, L. M. Olsson, K. Bauer, A. Pizzolla, P. Olofsson and R. Holmdahl, Antioxid. Redox Signaling, 2007, 9, 1541–1567 Search PubMed.
- S. Saxena, H. Vekaria, P. G. Sullivan and A. W. Seifert, Nat. Commun., 2019, 10, 4400 Search PubMed.
- C. Dunnill, T. Patton, J. Brennan, J. Barrett, M. Dryden, J. Cooke, D. Leaper and N. T. Georgopoulos, Int. Wound J., 2017, 14, 89–96 Search PubMed.
- H. Dou, S. Wang, J. Hu, J. Song, C. Zhang, J. Wang and L. Xiao, J. Tissue Eng., 2023, 14, 1–23, DOI:10.1177%2F20417314231172584.
- S. Zhou, M. Xie, J. Su, B. Cai, J. Li and K. Zhang, J. Tissue Eng., 2023, 14, 1–28, DOI:10.1177/20417314231185848.
- Y. Yao, H. Zhang, Z. Wang, J. Ding, S. Wang, B. Huang, S. Ke and C. Gao, J. Mater. Chem. B, 2019, 7, 5019–5037 Search PubMed.
- T. Wang, Y. Zheng, Y. Shen, Y. Shi, F. Li, C. Su and L. Zhao, Artif. Cells, Nanomed., Biotechnol., 2018, 46, 138–149 Search PubMed.
- T. Tang, H. Jiang, Y. Yu, F. He, S. Z. Ji, Y. Y. Liu, Z. S. Wang, S. C. Xiao, C. Tang, G. Y. Wang and Z. F. Xia, Int. J. Nanomed., 2015, 10, 6571–6585 Search PubMed.
- M. Nakayama, R. Sasaki, C. Ogino, T. Tanaka, K. Morita, M. Umetsu, S. Ohara, Z. Tan, Y. Nishimura, H. Akasaka, K. Sato, C. Numako, S. Takami and A. Kondo, Radiat. Oncol., 2016, 11, 91 Search PubMed.
- T. Thenmozhi, 3 Biotech., 2020, 10, 82 Search PubMed.
- H. M. Fahmy, N. M. Ebrahim and M. H. Gaber, J. Trace Elem. Med. Biol., 2020, 60, 126481 Search PubMed.
- K.-I. Lee, J.-W. Lin, C.-C. Su, K.-M. Fang, C.-Y. Yang, C.-Y. Kuo, C.-C. Wu, C.-T. Wu and Y.-W. Chen, Toxicol. In Vitro, 2020, 63, 104739 Search PubMed.
- S. R. Choudhury, J. Ordaz, C. L. Lo, N. P. Damayanti, F. Zhou and J. Irudayaraj, Toxicol. Sci, 2017, 156, 261–274 Search PubMed.
- S. May, C. Hirsch, A. Rippl, N. Bohmer, J.-P. Kaiser, L. Diener, A. Wichser, A. Bürkle and P. Wick, Nanoscale, 2018, 10, 15723–15735 Search PubMed.
- E. Abbasi-Oshaghi, F. Mirzaei and M. Pourjafar, Int. J. Nanomed., 2019, 14, 1919–1936 Search PubMed.
- O. M. Abd El-Moneim, A. H. Abd El-Rahim and N. A. Hafiz, Toxicol. Rep., 2018, 5, 771–776 Search PubMed.
- L. M. Rossbach, D. H. Oughton, E. Maremonti, C. Coutris and D. A. Brede, Sci. Total Environ., 2020, 721, 137665 Search PubMed.
- M. Ahamed, M. J. Akhtar, M. A. M. Khan, S. A. Alrokayan and H. A. Alhadlaq, Chemosphere, 2019, 216, 823–831 Search PubMed.
- X. Shi, Y. Tian, S. Zhai, Y. Liu, S. Chu and Z. Xiong, Front. Chem., 2023, 11, 1115440 Search PubMed.
- J. Bouayed and T. Bohn, Oxid. Med. Cell. Longevity, 2010, 3(4), 228–237 Search PubMed.
- A. Adhikari, S. Mondal, S. Darbar and S. Kumar Pal, Biomol. Concepts, 2019, 10(1), 160–174 Search PubMed.
- S. Hua, M. B. C. de Matos, J. M. Metselaar and G. Storm, Front. Pharmacol., 2018, 9, 790 Search PubMed.
- P. C. Naha, K. C. Lau, J. C. Hsu, M. Hajfathalian, S. Mian, P. Chhour, L. Uppuluri, E. S. McDonald, A. D. A. Maidment and D. P. Cormode, Nanoscale, 2016, 8(28), 13740–13754 Search PubMed.
- D. P. Cormode, L. Gao and H. Koo, Trends Biotechnol., 2018, 36(1), 15–29 Search PubMed.
- A. Nel, T. Xia, H. Meng, X. Wang, S. Lin, Z. Ji and H. Zhang, Acc. Chem. Res., 2013, 46(3), 607–621 Search PubMed.
- B. C. Nelson, C. W. Wright, Y. Ibuki, M. Moreno-Villanueva, H. L. Karlsson, G. Hendriks, C. M. Sims, N. Singh and S. H. Doak, Mutagenesis, 2017, 32(1), 215–232 Search PubMed.
|
This journal is © The Royal Society of Chemistry 2024 |