Critical evaluation of current isolation, detection, and genotyping methods of Cryptosporidium species and future direction†
Received
26th June 2023
, Accepted 15th April 2024
First published on 24th April 2024
Abstract
Globally, Cryptosporidium continues to pose a significant health and economic burden despite significant efforts to develop effective on-site biosecurity and best management practices. According to the Global Burden of Disease Study (2016), this parasitic protozoan is responsible for more than 48 thousand deaths in children under the age of five and 7.2 million disability-adjusted life-years worldwide. Additionally, most Cryptosporidium species (e.g., Cryptosporidium hominis, Cryptosporidium parvum, etc.) are resistant to the majority of common disinfectants and can survive outside the body for extended periods. Consequently, it is crucial to establish a prompt and accurate diagnosis as well as the severity of the disease to prevent the spread of parasites and to enable effective management strategies. USEPA Method 1623 is currently used in centralized laboratories to perform routine diagnostic tests for this parasite. This process is laborious, expensive, and time-consuming. In the past ten years, a multitude of techniques based on conventional molecular biology techniques, such as polymerase chain reaction (PCR), nested-PCR, loop-mediated isothermal amplification (LAMP), recombinase polymerase amplification (RPA), and nucleic acid sequence-based amplification, have been utilized to analyze Cryptosporidium species. Similarly, several biosensing techniques have been developed in recent years, including electrochemical biosensors and the CRISPR-Cas system in lateral flow assays. This review discusses the most frequently employed techniques for isolating, quantifying, and genotyping Cryptosporidium species, and their implication on the diagnostic landscape. A subsequent evaluation of the most significant technical and biological challenges and limitations of these techniques is also undertaken.
Water impact
Cryptosporidium poses a significant health and economic burden despite efforts in diagnostics, biosecurity and best management practices. To ensure safe water access, independent evaluation of existing and emerging technologies for isolating, quantifying, and genotyping Cryptosporidium species is crucial. This review examines these techniques, discussing their impact on diagnostics. Evaluation of technical and biological challenges and limitations is also included.
|
1. Introduction
Cryptosporidium species is an obligate intracellular intestinal parasite that infects a wide range of living hosts such as mammals, vertebrates, and avian species.1 Accidental ingestion of Cryptosporidium oocysts causes cryptosporidiosis, characterized by moderate-to-severe diarrhea (MSD) and diarrhea-associated diseases in individuals. Generally, infections are self-limiting in otherwise healthy adults, however, this could be severe, prolonged, and life-threatening for those who are immunocompromised, malnourished, elderly, or under five years of age.2,3 The global prevalence (∼7.6%) of Cryptosporidium varied in developed and developing countries, 4.3% and 10.4%, respectively.4 According to the Global Burden of Diseases (GBD) 2016, cryptosporidiosis was ranked as the fifth leading cause of diarrhea-related mortality in children under 5 with over 48
000 deaths and 7.2 million disability-adjusted life-years (DALYs) worldwide.5 Both symptomatic and asymptomatic cryptosporidiosis in early childhood lead to malnutrition, stunted growth, and poor cognitive development.6,7 A recent multisite study revealed that Cryptosporidium causes severe episodes of diarrhea in the first year of birth.8 However, there is no vaccine available9 and nitazoxanide is the only FDA-approved drug used for the treatment of cryptosporidiosis which is restricted to patients with ≥1 year of age.10 This drug is effective in immunocompetent adults and appears to be ineffective for malnourished children.11–13
Once the infection is established in a host, Cryptosporidium oocysts undergo alternating asexual and sexual reproduction inside the apical surfaces of the host gastrointestinal tract and produce thick-walled oocysts which are shed in feces (Fig. 1).14,15 Public water supplies such as catchments and watersheds may easily become contaminated either from direct contact with manure from the animal farm or from pathogen-loaded compost that is applied to fields as a fertilizer and subsequently carried away by heavy precipitation.16,17 The thickened outer layer of the oocyst protects it from environmental stress and remains infectious in the aquatic environment for months.18 Moreover, conventional water treatment using chemical disinfection (such as chlorination) has proven to be ineffective, therefore, poorly maintained or operational failure of filtration systems in the public water supply is one of the prime concerns for plausible human exposure to this parasite.19 One of the first recorded, and most significant Cryptosporidium water incidents happened during 1993 in Milwaukee which directly affected approximately 403
000 people and cited drinking water treatment deficiency as the most likely cause of the outbreak.20,21 Since then waterborne outbreaks have continued to happen despite all the state-of-art technologies and management practices in place. According to a recent report, Cryptosporidium spp. has been the etiological agent for 60.3% (120) and 63% (381) of waterborne parasitic outbreaks that happened during 2004–2010 and 2011–2016, respectively.22 Although the infection dose varies depending on the species and isolates (10 oocysts for C. hominis TU502,23 30 for C. parvum IOWA isolate24), it is considerably very low. Considering all these with the resistant nature of oocysts, and fecal-to-oral route of transmission, this organism is marked as a reference for protozoan pathogen for monitoring drinking water safety by the World Health Organisation (WHO).25,26
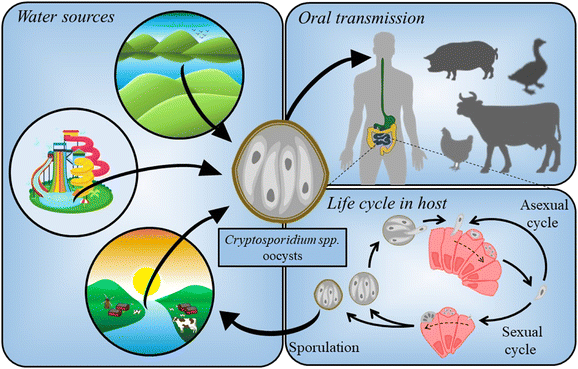 |
| Fig. 1 Simplified life cycle of Cryptosporidium spp. | |
Waterborne outbreaks directly impact the water industry, particularly in terms of managing a safe municipal water supply and the increased medical expenses incurred by government agencies to ensure public health and wellbeing. However, there are no standardized criteria for measuring economic losses in such cases. Corso et al.27 estimated the medical costs and lost productivity to be $96.2 million for the 1993 Milwaukee outbreak, while Chyzheuskaya et al.28 estimated that the 2007 Cryptosporidium hominis outbreak in Ireland cost the public and private sectors approximately $22.44 million. The long-term impact of community outbreaks is much more detrimental than the direct economic losses. Therefore, in addition to promoting good hygiene practices, prioritizing the sensitive, rapid, and on-site detection of this parasite is crucial as a preventive measure for prompt decision-making and mitigating the devastating public health and economic consequences.
Over the years, isolation and detection have been the main focal points in the analysis of Cryptosporidium from water samples. Various filtration methods, such as membrane,29–31 cartridge,32–35 ultrafiltration,36–39 flocculation, and sedimentation,40–42 have widely been explored for concentrating and isolating Cryptosporidium from diverse water sources. The use of direct continuous flow centrifugation has made the initial isolation and concentration of oocysts rapid and cost-effective.43,44 These concentration methods suffer from significant material loss or low recoveries, which can ultimately lead to false-negative detections depending on the sensitivity of the downstream detection methods. The utilization of polymeric materials in fabricating engineered micro and nano-filters has resulted in improved filter durability and enhanced effectiveness in removing debris, thereby aiding the recovery of Cryptosporidium during filtration-based isolation processes.45–47 In terms of detection, apart from immunofluorescence and nested PCR, other amplification techniques, including loop-mediated isothermal amplification,48 and nucleic acid sequence-based amplification,49–52 have been widely employed for on-site viability analysis of the parasite. Nanotechnology-based methods have also been introduced in recent years for Cryptosporidium detection.53–57 For instance, CRISPR-Cas12a diagnostics,53 nanoparticle-assisted electrochemical ELISA integration,55,58 aptamer-based electrochemical assays,56 and the use of micromesh57 in microfluidic-based capturing of Cryptosporidium have contributed to the advancement of sensitive parasite detection.
It is important to note that approximately 95 percent of human cases are caused by two Cryptosporidium spp., namely C. hominis and C. parvum.2 However, there are 44 known species and over 120 genotypes of Cryptosporidium spp. that can infect mammals, birds, fishes, reptiles, and amphibians.2 Therefore, genotyping plays a pivotal role in surveillance to identify contamination sources and understand the zoonotic potential of the infecting species. In recent years, an open-source software called CryptoGenotyper has been developed and released which allows users to analyze sequencing data to accurately identify the genotypes of Cryptosporidium spp.59 Another community-driven bioinformatics resource and database named CryptoDB60 (http://CryptoDB.org) has been launched. This database contains manually curated reference genomes, whole genome sequences, and annotations, along with supplemental bioinformatics analysis and data-mining tools.
Several excellent reviews on the biology, pathology, sequencing, biochemical properties, and detection methodologies of Cryptosporidium spp. can be found in the literature. For instance, Bouzid et al.,61 Guérin et al.,62 and Pinto et al.63 reviewed host–parasite interaction, virulence factor for pathogenesis, and Cryptosporidium life cycle. Feng et al.64 have focused on the genetic diversity and population structure of Cryptosporidium, while Certad et al.65 have extensively discussed the pathogenic mechanism. Ryan et al.66 have reviewed the life cycle, genomics, and vaccine research and later produced a more comprehensive and updated review on the topic.67 Khan et al.68 have extended the scope of their review and covered the molecular epidemiology of the species. In the review by O'Leary et al.,69 the topic was broadened to provide a thorough breakdown of the advantages and disadvantages associated with various methods for analyzing Cryptosporidium species. This review was the first attempt to consider the field from a broader perspective, critically evaluating major breakthroughs in tractable C. parvum oocyst diagnostics and indicating tentative steps toward advancing Cryptosporidium research. Bridle et al.70 have reviewed the implementation of miniaturized total analysis systems in Cryptosporidium analysis, while Jain et al.19 and Luka et al.71 have focused on current and emerging state-of-the-art methods/tools for the analysis of protozoan cysts and oocysts in water samples. Although most of these review papers summarise isolation72 and detection19,69–71 of Cryptosporidium spp. individually or concurrently, no single review paper has discussed the concurrent isolation, detection, and genotyping of Cryptosporidium spp.
In this review, we first focus on the latest advancements in the isolation and detection of Cryptosporidium following USEPA guidelines. We then shift our focus towards the current demonstrations of genotyping and subtyping techniques for Cryptosporidium species. Finally, we highlight the major challenges involved in concentrating, isolating, detecting, and genotyping Cryptosporidium species in water samples. We also discuss the future directions and perspectives needed to achieve on-site isolation, quantitative detection, viability assessment, and genotyping of Cryptosporidium spp.
2. Conventional methods for Cryptosporidium isolation from water sample
Generally, all USEPA methods involve three significant steps: (i) sample concentration through filtration and elution, (ii) selective separation of oocysts from other debris using Percoll sucrose density gradient centrifugation or immunomagnetic separation (IMS), and (iii) fluorescence staining and detection using fluorescence microscopy (see Fig. 2).73–76 The filtration of water samples can be carried out in laboratory settings or in the field using a portable pump and filter to concentrate Cryptosporidium and remove unwanted substances from the water sample. Over the years, cartridge (capsule) and membrane filters have been widely used. The cartridge filtration approach has gained popularity due to its association with the early USEPA method. Cartridge filters are membrane filters that are commercially available under various brands, such as Filta-Max™ and Filta-Max Xpress by IDEXX,77,78 Envirochek™ Polyethersulfone and Envirochek™ Polyester HV by Pall,34,35 Whatman® CrypTest™ Polycarbonate track-etched membrane (a discontinued product) by Whatman,79 microporous polypropylene based Micro Wynd II by AMF/Cuno.80
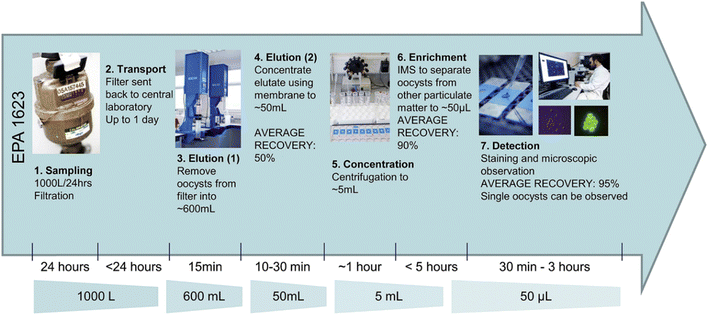 |
| Fig. 2 Sampling, process, and time required to execute USEPA 1623 (reprinted with permission from ref. 70). | |
Apart from these filters, various polymeric materials such as polycarbonate,81–83 cellulose acetate,29–31 cellulose nitrate,31,84 mixed-cellulose ester77,85,86 have been used to make the membrane of variable pore sizes between 0.45 μm and 5.0 μm to concentrate Cryptosporidium oocysts from the water sample. The choice of filter depends on the recovery efficiency (RE) of the filter, which refers to the proportion of organisms recovered compared to the total number initially added in a seeded positive control.87 Multiple factors, including the filter's pore size, water quality (turbidity), and elution process of filtered parasites, influence the RE. Furthermore, the recovery rate can vary across different sampling sites, sampling times, and sample volumes to be analyzed.88 For instance, Lee et al.89 demonstrated that all four filters (Filtra-Max filter, Envirochek HV™, CrypTest™, and Sigma+™) exhibited higher RE in source water compared to finished water. Higher seeding amounts of Cryptosporidium in 10 L of water led to improved RE for each filter cartridge, indicating the impact of different sample loads on the RE. In another study by Karim et al.,79 the Filtra-Max filter showed the lowest RE when employed for surface and drinking waters, while Envirochek HV and CrypTest™ demonstrated higher RE.79,89 Furthermore, Kim et al.90 found that incomplete elution following filtration accounted for 70% of the loss of oocysts. To address this issue, Inoue et al.91 proposed the use of a specialized eluting filter solution called PET, which contained sodium pyrophosphate (0.02%), Tween 80 (0.01%), and trisodium EDTA (0.03%). They suggested that regardless of the filtration method, PET improved the dispersion of the filtrate and reduced the adhesion of clay particles to the oocysts, resulting in enhanced capture efficiency and target recovery during immunomagnetic separation.91 Backwashing the filter after filtration is also an effective strategy to achieve better recovery, although this is limited to the filter design of the Envirochek HV™ filter. In essence, while cartridge filtration offers superior RE compared to membrane filtration, it is relatively expensive and impractical for regular monitoring due to the bulky instrumentation involved. On the other hand, membrane filtration reduces costs but may face clogging issues when processing more than 10 liters of water samples.88
Ultrafiltration is another mode of membrane filtration that offers satisfactory RE. For instance, dead-end ultrafiltration (RE, 49–87%)92,93 and hollow-fiber ultrafiltration (RE, 51–65%)36,93 have demonstrated superior performance compared to Envirochek HV™, serving as a substitute for USEPA 1623 filtration approaches.36–39,92,93 Recently few promising nanofabricated filters have been reported with the higher recovery of oocysts, such as microporous polymeric microfilters (RE 95–99%),45 high-flux metallic micro/nano-filtration membrane (RE 85%),46 Counter-Flow Micro-Refinery (CFMR) systems (RE 81.3%),47 and a metallic filter (RE 84·9%) with an ultrasound transducer for eluting oocysts.94 Filtration-free concentration method, like continuous flow centrifugation (CFC), has gained attention due to comparative simplicity, single-step operation, rapidity, and relative affordability.95–97 Zuckerman et al.43 conducted a cross-laboratory validation of portable continuous flow centrifugation (PCFC) of oocysts and compared it with USEPA method 1623. A recovery rate ranging from 35% to 66.8% was reported depending on the water type, matrices, and volume (10 L to 1000 L) of the water processed. In general, average recovery drops for PCFC when tested water volume increases.43 PCFC received USEPA approval due to its ability to centrifuge over 50 liters of water and concentrate oocysts while maintaining the same or higher level of RE compared to capsule filtration (see Table S1† for comparison).74 However, recent research has revealed discrepancies between the field-level performance of CFC and other recognized techniques.72
Overall, USEPA method 1623.1 has been validated for detecting Cryptosporidium spp. (and Giardia) in surface water and water from other sources. The detection of Cryptosporidium in these samples poses extreme analytical challenges since it often exists below the detection limit of the method, typically around 0.2 to 1 oocyst per L (when using 10 L samples).97 During the pre-concentration of oocysts from large volumes of water (usually ranging from 10 L to 100 L), oocysts can become contaminated with organic debris, particulate matter, and algal cells of the same size and specific gravity as oocysts. Depending on the water source, more than 106 particles between 2.0 and 15 μm have been reported.88 Although IMS helps selectively isolate Cryptosporidium oocysts, it can also be compromised by the dissolved iron content in the water source.98
Microscopy-based detection in the final step of this method takes advantage of the specificity of monoclonal antibodies. However, currently available antibodies cannot distinguish species differences on the oocyst wall surface.99 Autofluorescence from debris and false-positive responses from oocyst-sized particles continue to complicate the detection process, requiring trained professionals to identify oocysts by observing the DAPI-stained nuclei of the oocyst.97 The expensive analysis cost (US $700) per sample, lengthy sample preparation time, and bulky instrumentation make it unsuitable for in-field applications.70 Consequently, the USEPA method does not truly fulfill the intended purpose of surveillance, as it lacks prompt sample analysis and instead provides information on outbreaks that have already occurred. While increasing the frequency of sample collection and testing does achieve the objective of monitoring the safety of the drinking water supply in developed countries, the ideal option, regardless of a country's economic status, would be an affordable and effective portable concentration technique combined with rapid molecular-based detection.
3. Nucleic acid-based detection methods
Nucleic acid-based identification of Cryptosporidium involves targeting critical genes, such as the small subunit 18S (SSU) rRNA gene, Cryptosporidium outer wall protein (COWP), 70-kDa heat shock protein (hsp 70), thrombospondin-related adhesive protein (TRAP-C2), and actin gene.100 These genes are highly suitable for identifying Cryptosporidium species due to the presence of numerous interspecific polymorphisms. Among these genes, the 18S rRNA gene stands out due to its multi-copy nature. 20 copies of the target locus of the 18S rRNA whereas 4 copies hsp 70, COWP, TRAPC-2, and actin gene exist in each oocyst.101,102 Consequently, targeting this gene provides superior performance (see Table S2† for comparison of molecular methods) in molecular detection compared to other genes.101 Nucleic acid-based detection and identification can be categorized (Fig. 3) into the following two, (i) without molecular amplification such as fluorescent in situ hybridization (FISH), (ii) molecular amplification based such as PCR, qPCR, RT-qPCR, LAMP, RPA, NASBA etc. (iii) sequencing such as Sanger and next generation sequencing. Amplification-based methods predominantly utilizing the 18S rRNA gene, offer several advantages. It provides better sensitivity and specificity, the ability to target different genes through multiplexing, and it is comparatively faster than conventional microscopy or immunoassay methods.103 Particularly in water sample analysis, oocysts isolated through IMS can undergo DNA extraction either before or after microscopic examination for molecular identification.
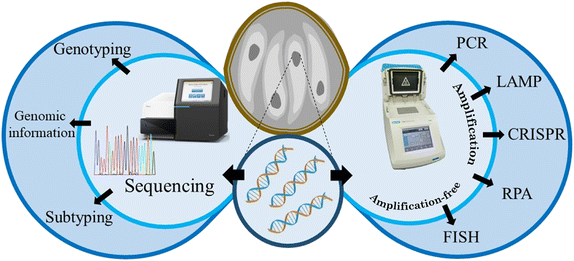 |
| Fig. 3 Nucleic acid-based identification methods for Cryptosporidium oocyst detection consist of two main groups which are molecular detection and sequencing techniques. | |
Fluorescent in situ hybridisation (FISH) was developed by Delong et al.104 to detect formaldehyde-fixed intact microbial cells. It uses fluorescently labelled oligodeoxynucleotides complementary to 16S ribosomal RNA (rRNA) in situ and visualised (detected) by fluorescent microscopy.104 FISH has proved to be useful for microbial identification from mixed populations.105 Vesey et al. is one of the pioneers who designed a FISH probe named CRY1 probe (see Table S1† for primers and probe information), for simultaneous detection and analysis of the viability of C. parvum oocysts. CRY1 probe selectivity was tested against C. parvum, C. baileyi, and C. muris oocysts and found to be specific for C. parvum.106 18S rRNA copies (3.5 × 105) per oocyst is the limiting factor for FISH and Deere et al.107 concluded that fluorescence signal intensities cannot be increased beyond the levels reached by Vesey et al.106 by optimizing the permeability of oocysts. Following this, they evaluated different fluorochromes for FISH labelling toward flow cytometric detection of C. parvum oocysts. According to them, FITC is the most promising fluorochrome when it is bound to the CRY1 probe with 18-carbon spacer arm with six ethylene glycol moieties which resulted in a fivefold increase in signal intensities compared to FITC directly conjugated to 5′ position of the probe. The exact reason for increased signal intensities was poorly understood and commented that stoichiometry and the physiochemical environment after probe binding could assist in sensitivity.108 To identify the human infectious Cryptosporidium spp. (C. parvum and C. hominis), Alagappan et al.109,110 validated two probes (Cpar677 for C. parvum, Chrom253 for C. hominis,). Specificity and cross-reactivity of the probes were evaluated against 8 Cryptosporidium spp. (C. parvum, C. hominis, C. andersoni, C. muris, C. meleagridis, C. felis, C. cervine genotype, and C. rabbit genotype). While Cpar677 did not show any response other than C. parvum, Chrom253 was found to be positive for C. hominis and C. rabbit genotype due to 100 percent 18S rDNA sequence homology between them. These two probes were able to identify more than 80% of C. parvum and C. hominis in a mixture of these two spp. and results agreed with PCR-RFLP of 18S rDNA.109,110
PCR-based analysis and identification of the pathogen in water originated in the late 1990s.111–113 Despite the sensitivity, the biggest pitfall for PCR is that it cannot determine the infectivity of the oocyst. Rochelle et al.114 used cell culture and reverse transcriptase PCR of HSP 70 mRNA to assess viability. As mRNA quickly degrades within the cell, it has been established as a reliable marker for testing viability and infectivity. In this assessment, oocysts were inoculated into 70–100 percent confluent monolayer of the Caco-2 cell line. Before extraction of mRNA, cells were incubated at 35 °C for 96 hours. Subsequently, hsp 70 transcript was detected by reverse transcriptase PCR (RT-PCR) with C. parvum-specific primers from extracted mRNA. This approach detected a single oocyte. Later, Di Giovanni et al.115 integrated PCR with in vitro culture (HCT-8 cell line), termed CC-PCR, to test the infectivity of the oocyst by detecting the HSP 70 gene directly from cell lysate without nucleic acid purification. The study was conducted with a total of 122 raw and 121 filter-backwashed water samples, and the results were compared to the immune-fluorescence assay (IFA). While CC-PCR results align with the results of oocyst recoveries from all types of water samples measured by IFA (both IMS-IFA and floatation-IFA), it slightly deviates from the flotation-IFA result when testing environmental samples. CC-PCR detected infectious C. parvum in the 7.4% of total filter-backwash water samples as opposed to 5.8% for flotation-IFA. This discrepancy is unexpected considering flotation-IFA detects all types of oocysts, i.e., live, dead, or infections. Possible reasons for this difference could be the splitting of samples with a small number of oocysts for IFA and CC-PCR, or incomplete dissociation of oocysts in IMS before inoculating cells.115 Although both techniques are comparatively laborious and time-consuming, it has been proposed that CC-PCR, in conjunction with IFA, can be used for routine monitoring of oocysts in drinking water.116,117
PCR is prone to false-negative responses due to the presence of contaminants in the water sample, such as humic acids, clay, debris, and polyphenols.118,119 Such outcomes pose a significant health risk as the pathogen may be present but remain undetected. To address this, an internal control, also known as an internal positive control, should be employed to monitor the successful molecular amplification.120 This control is a synthetic target distinct from the primary target that is amplified using the same primer set or a different one. Negative amplification of the primary target alone is considered acceptable if the internal control amplifies successfully. To enhance the specificity of the PCR reaction, nested PCR was developed. This technique utilizes two sets of primers to amplify a specific DNA region. The first set of primers binds to the outer region of the target, and the resulting product serves as a template for the second primer pair. By employing nested PCR, any non-specific amplification from the first set of primers will fail to amplify with the second set, thereby increasing the specificity of the reaction. As a result, nested PCR has become a common practice for Cryptosporidium detection in wastewater and catchment water across different regions of the world since the early 2000s.121–123
By enhancing sensitivity and reducing assay time, quantitative PCR (qPCR) has become an essential tool for environmental pathogen screening.124–126 One of the key advantages of qPCR is its quantitative nature, providing more than just a presence-or-absence result. Unlike conventional PCR, which mainly analyses the endpoint, qPCR offers a wide dynamic range of detection, enabling the identification of a few copies of the target gene due to its high sensitivity to DNA-binding dyes. Analysis can also be carried out during the assay itself, significantly reducing the total assay time.127 Multiplexing capability is another significant benefit of qPCR. For instance, Guy et al.124 designed a TaqMan probe-based multiplex assay where simultaneous detection of Cryptosporidium and Giardia spp. In subsequent studies, Hadfield et al.100 developed a TaqMan probe-based qPCR assay targeting the 18S rRNA gene, which successfully detected all Cryptosporidium spp. regardless of the intraspecies genetic variability in human clinical samples. It is now well known that through proper primer design and melting curve analysis, pathogenic Cryptosporidium species can be differentiated from non-pathogenic ones. Li et al.128 demonstrated this by using fluorescence resonance energy transfer probes and melting curve analysis in the 18S-LC1 and hsp 90 assays, allowing the distinction of common human-pathogenic species (C. parvum, C. hominis, and C. meleagridis). Similarly, the 18S-LC2 assay enabled the differentiation of non-pathogenic species (such as C. andersoni) from human-pathogenic species commonly found in the source water. Quantitative detection by qPCR depends on the accuracy of the standard curve, which can be affected by pipetting error and DNA losses. In contrast, droplet digital PCR (ddPCR) provides absolute quantification of DNA/gene copy numbers without the need for a standard curve. Yang et al.129 applied and validated ddPCR for the detection of Cryptosporidium spp. (18S rRNA and actin gene) from sheep, cattle, and humans, comparing its performance with qPCR along with a comprehensive cost analysis of these two methods. According to their study, the sensitivity of both methods is comparable. However, ddPCR offers precision at a higher price and is not susceptible to the inhibitors present in feces. Authors suggested calibrating qPCR standards using ddPCR to enhance precision, a method later applied by Zahedi et al.130 in analysing Cryptosporidium spp. (18S and gp60 loci) in dam water.
The sensitivity of PCR can be compromised by inhibitors present in the water sample. The loop-mediated isothermal amplification (LAMP) method offers a solution to this challenge. The method utilizes the strand displacement activity of Bst polymerase and detects a target genes within 30–60 minutes at isothermal condition (60–65 °C). This method exhibits unparalleled sensitivity, selectivity, and robustness when it comes to handling environmental samples, surpassing other molecular techniques.131,132 In the pioneering work by Karanis et al.,48 LAMP primers targeting the 60 kDa glycoprotein (gp60) were designed and applied for the detection of Cryptosporidium spp. in fecal and water samples. The researchers achieved an impressive sensitivity that enabled the detection of just 1 oocyst. Subsequent studies have compared LAMP with PCR/nested-PCR, IFA, and other methods for detecting Cryptosporidium in water samples.133–137 For example, Bakheit et al.133 demonstrated that LAMP could detect Cryptosporidium in samples that tested negative with nested PCR, reaffirming the superiority of LAMP over the more sensitive version of PCR. In their study, LAMP employed primers targeting the SAM-1 and gp60 genes, while nested-PCR targeted the 18S rRNA gene. Mahmoudi et al.134 and Gallas-Lindemann et al.135 conducted similar investigations, which confirmed the superior performance of LAMP (targeting the SAM-1 gene) in detecting Cryptosporidium when compared to IFA and nested-PCR (targeting the 18S rRNA gene).
Another isothermal amplification process known as nucleic acid sequence-based amplification (NASBA) is used to amplify single-stranded RNA by mimicking retroviral RNA replication by avian myeloblastosis virus reverse transcriptase (AMV RT), RNase H, and T7 RNA polymerase. The RNA serves as a precursor for AMV RT, ensuring the continuous cyclic phase of the reaction at 41 °C for 1.5–2 hours to amplify.138,139 During RNA amplification by NASBA, potentially contaminating DNA does not interfere amplification condition is set below the melting point of genomic or double-stranded DNA.140 As it amplifies RNA, it has been used to differentiate live and dead oocysts by amplifying mRNA markers. Most NASBA-based detection and viability testing targeted the hsp70 mRNA.50–52 Baeumner et al.50 were the first to utilize NASBA for detecting viable oocysts in environmental water samples. In their optimized technique, oocysts were isolated using vortex flow filtration and IMS, followed by a 20-minute heat treatment at 45 °C. This heat stimulation induced the expression of the hsp70 gene, which allowed subsequent RNA extraction and NASBA reaction. The authors employed an electrochemiluminescence probe for detecting the amplified products and achieved a limit of detection (LOD) of 10 mRNA molecules (approximately 5 viable oocysts) when IMS was conducted on spiked oocysts in 10 mL of contaminated water. Hønsvall et al.141 used MIC1 mRNA to assess viability and differentiate between C. parvum and C. hominis. The MIC1 gene encodes a protein essential for host cell attachment and invasion, reflecting the infectivity of oocysts. C. parvum possesses two exons in the MIC1 gene, while C. hominis only has the first exon. For the real-time NASBA assay, a specific set of primers and fluorescently labelled probes were designed to target the second exon, while another set targeted the first exon. Differential analysis of the results obtained from both primer amplifications determined whether the sample contained C. parvum or C. hominis. This method detected MIC1 mRNA isolated from as low as 5 oocysts (LOD). mRNA was isolated according to the NucliSens® miniMAG® protocol (Biomérieux, Lyon, France), modified with boiling and proteinase K treatment of oocysts.141 According to a previous report, MIC1 mRNA was detected from both live and inactivated oocysts, which contradicts the reliability of the mRNA-based viability assessment.49–52,141,142 The authors argued that RNA remained protected from degradation even after oocyst inactivation.141 RNA analysis continues to be used as a viability marker for Cryptosporidium oocysts, despite reliability concerns. Therefore, more reliable and sensitive methods are needed to expand the options for a comprehensive analysis of Cryptosporidium viability markers.
Until the early 2010s, Sanger sequencing (SgS) was the only tool to be used for specific identification of Cryptosporidium spp. SgS unable to resolve mixed infection or complex DNA mixtures having low-abundance variants without additional molecular cloning methods.143,144 On the contrary, next-generation sequencing (NGS) has the sensitivity to detect low-abundant DNA copies or amplicons which makes it suitable for epidemiological monitoring. Recently, Braima et al.145 analyzed cryptosporidiosis cases reported in 2019 and 2020 in Western Australia by SgS and NGS of the gp60 gene. While SgS underestimated the mixed infection, about 28% (5/18) and 5% (4/83) stool samples were reported to be positive for mixed infection by NGS in 2019 and 2020, respectively.145 Similarly, Bailly et al.143 reinvestigated the 102 DNA samples from the Grasse outbreak (French), which happened in 2019. They performed NGS on the gp60 locus and detected a minority subtype (C. parvum-IIaA11G2R1) in a sample, originally undetected by SgS during the initial investigation.143 18S rRNA and gp60 amplicon NGS have been reported for monitoring of Cryptosporidium spp. from river water and riverbed,146 and wastewater treatment plants.144,147 A study performed by Zahedi et al.147 detected no Cryptosporidium spp. with eukaryotic 18S (polymorphic V9 region) NGS but 12/26 were positive with targeted Cryptosporidium-specific NGS, suggesting that the V9 region lacks sensitivity to detect low abundance intestinal species in wastewater. NGS could identify the sample as a mixed infection (false positive) due to polymerase slippage. The use of high-fidelity Taq polymerase, designing primers without degenerate bases, removing chimeric sequences, ensuring strict laboratory practices, and selecting an optimal cutoff value for NGS data processing could reduce the possibility of such identification.143,145,148
4. Current biosensing methods
In biomedical sciences, point-of-care (POC) devices are used to quickly identify and analyze substances at the patient's location, including within their homes. When it comes to environmental sample analysis, the more appropriate term is “point-of-need” (PON) testing.149 The advancement of nanotechnology and material science has facilitated the development of efficient, sensitive, and rapid innovative approaches for managing microbial outbreaks. These approaches aim to address the limitations of traditional detection systems and meet some of the ASSURED criteria set by the World Health Organization (WHO): affordability, sensitivity, specificity, user-friendliness, robustness, rapidity, equipment-free operation, and deliverability to those in need.150,151 In recent years, nanotechnology-based PON platforms, with their rapid, selective, inexpensive, and customizable detection strategies, have gained popularity for water pathogen detection. The following section provides a description of the major sensor types currently utilized for Cryptosporidium oocysts detection. A table (Table S3†) has been provided to understand the strengths and weaknesses of the currently developed sensor.
4.1 Electrochemical detection
In general, the electrochemical biosensor is a type of sensor in which biochemical events, such as antigen–antibody interactions, are transduced into electrical signals. These signals can manifest as changes in current, capacitance, or resistance.152 In this sensing method, nanomaterials are often used both as tracer tags or host elements that hold recognition components and catalytic moieties.153 More recently, direct adsorption of biomolecules onto the electrode surface has also shown great promise in the electrochemical biosensing of various biomolecules.154 While electrochemical sensors are known for their rapidity, portability, and cost-effectiveness, they often encounter issues with non-specific adsorption. This can result in erratic system behaviour if not handled with the necessary precautions. To address this, measures such as using bovine serum albumin (BSA) to block the exposed electrode surface or purifying the target magnetically before introducing it to the electrode surface are commonly employed.154,155
When the principle of an electrochemical immunosensor is employed, sensing can be achieved through either label-free methods or enzyme-mediated electron transfer-based approaches. Label-free detection, by definition, does not require any enzyme or nanoparticle-labelled secondary antibody/nanoconjugates. Instead, it depends on the intrinsic properties of the target antigen/organism captured on the primary antibody. For example, in the case of quartz crystal microbalance (QCM), which depends on mass, impedimetric sensors depend on resistance or capacitance.54,55,156 Luka et al.54 presented a label-free capacitive biosensor, as illustrated in Fig. 4(A). They fabricated interdigitated gold electrodes through standard photolithography. Each sensor consisted of 18 pairs of interdigitated electrodes, with a width of 30 μm and length of 500 μm. A self-assembled monolayer (SAM) of recombinant protein G/thiol formed on the gold electrode due to gold-thiol interactions, followed by the modification of anti-Cryptosporidium antibody. The modified electrode successfully captured spiked Cryptosporidium, leading to an increase in relative capacitance proportional to the amount of captured Cryptosporidium oocysts. This sensor exhibited a linear detection range of 15 to 153 oocysts, with a detection limit of 10 oocyst per μL (40 oocysts/5 μL). The same group recently reported an impedimetric sensor based on the previously explored principle of immunosensing.43 This sensor demonstrated increased sensitivity (LOD 4 oocyst per μL), which can be attributed to the larger electrode area of 6.3 mm2 compared to 0.27 mm2 in the previous report, as well as the careful optimization of experimental parameters.55 Although the authors envision that both of these sensors could potentially replace immune-fluorescent measurements performed in USEPA 1623, their performance needs to be reassessed in the presence of an inhibitory matrix of environmental water to establish their efficacy in the field.
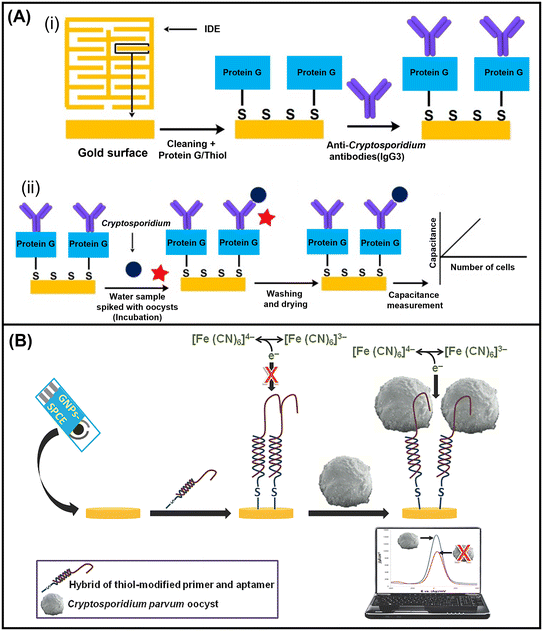 |
| Fig. 4 (A) (i) Surface functionalization of interdigitated gold electrodes with mAb and (ii) application for capacitive detection of Cryptosporidium oocysts (reprinted with permission from ref. 54). (B) Schematic representation of an electrochemical apta-sensor for Cryptosporidium detection (reprinted with permission from ref. 56). | |
The use of nanomaterials as electrode surface modifiers has become a common choice due to their larger surface area, which enhances the binding of recognition molecules on the electrode surface, thereby increasing the chances of binding to the target molecule and amplifying the signal. For instance, Thiruppathiraja et al.58 reported a sensitive Cryptosporidium detection method using an electrochemical sandwich ELISA on an Indium tin oxide (ITO) electrode. They functionalized the ITO electrode with (3-aminopropyl) triethoxysilane and AuNPs, enabling surface conductivity and hosting of anti-Cryptosporidium antibodies. Upon recognition of Cryptosporidium on the electrode surface, a dual-labeled AuNP probe (modified with alkaline phosphatase and anti-Cryptosporidium antibodies) formed a sandwich structure. Alkaline phosphatase oxidized p-nitrophenyl phosphate (p-NPP) to p-nitrophenol (p-NP), and the differential pulse voltammetry (DPV) signal was measured.58 By comparing this study with Laczka et al.,157 the impact of nanoparticles on sensitivity becomes evident. Although the two platforms were independent of each other, with the former utilizing AuNP-modified ITO and the latter using only a screen-printed carbon electrode (SPCE) as a transduction element, the high sensitivity of the AuNP-modified ITO electrode (LOD = 3 oocysts per mL) likely resulted from the metallic nanoparticle-assisted surface conductivity and the dual-labeled AuNP probe, in contrast to the SPCE (LOD = 5 × 102 oocysts per mL).
A study conducted by Houssin et al.156 proposed the use of impedance spectroscopy to analyze the viability of oocysts. According to their findings, a 15% difference in conductance between dead and live oocysts was observed, likely due to metabolic activities. This passive approach aimed to gain insights into the infectivity of the parasite. In a related study, Dibao-Dina et al.158 investigated the infectivity of Cryptosporidium on HCT-8 cells by measuring impedance. HCT-8 cells were cultivated on an interdigitated microelectrode array (IMA) followed by ensuring infection with sporozoites. Once infection was set, impedance signals were recorded every 7 minutes at frequencies of 1 kHz and 100 kHz. Between 9 and 40 hours after infection, three distinct peaks within the impedance spectra were observed compared to the control (freeze-dried oocysts). These peaks were attributed to the propagation of different stages of the life cycle and the interaction between the host and the parasite, serving as an indicator of infectivity.158
Due to the high cost and challenges associated with producing and purifying antibodies, DNA/RNA aptamers have emerged as a more affordable alternative in electrochemical biosensing methods. Their ease of synthesis in ubiquitous oligonucleotide facilities makes them highly accessible. Iqbal et al.56 developed a library of DNA aptamers and utilized the SELEX (systematic evolution of ligands by exponential enrichment) process to select aptamers with the best affinity for Cryptosporidium. For electrochemical detection, they have immobilised thiolated DNA aptamers onto gold electrodes, allowing interaction with Cryptosporidium. When successful binding occurs between the aptamers and Cryptosporidium, results in change in the conformation of the attached aptamer on the electrode surface. As a result of this electroactive mediator ([Fe(CN)6]4−/[Fe(CN)6]3−) can easily be oxidized to the electrode surface, resulting in a change in relative current (Fig. 4(B)). Although this sensor had a detection limit of almost 3 oocysts per μL (100 oocytes/30 μL) and was not as sensitive as reported by Thiruppathiraja et al.58 (LOD 3 oocysts per mL), the authors suggested that the sensor holds potential for differentiating between different genotypes, which necessitates careful consideration of aptamer design.56
4.2 Optical biosensors
4.2.1 Colorimetric detection.
Colorimetric-based detection strategies with color-forming mechanisms are primarily categorized based on the type of analyte being detected. When the analyte consists of the entire oocyst, the concept of ELISA is predominantly employed.159,160 The colorimetric response typically arises from an enzyme, nanozyme, or nanoparticle-labeled antibody with an appropriate substrate. In the case of detecting analyte-specific nucleic acid sequences, it relies on enzyme-labeled oligonucleotide probes or the specific interaction between nucleic acids and nanoparticles or colorimetric dyes. This type of detection offers the crucial advantages of rapid decision-making through naked-eye observation. They are relatively cost-effective compared to other type of sensors. The enhanced surface area of nanomaterials and their synergistic effects with enzymes or catalytic nanomaterials have significantly facilitated the efficient design of nanoparticle probes for this sensor type. Thiruppathiraja et al.160 demonstrated this by developing a colorimetric Cryptosporidium immuno-dot blot assay that utilized a dual-labeled AuNPs for antigen recognition and colorimetric signal generation. Initially, AuNPs were modified with C. parvum detection antibody and alkaline phosphatase (ALP) enzyme. Then, naked eye detection of C. parvum was performed using this dual-labeled AuNP probe in a direct ELISA format. This approach exhibited a 500-fold increase in sensitivity compared to conventional ELISA, which employs a secondary antibody modified with ALP, and achieved a detection limit of 10 oocysts per mL.160 This result aligns with the previously reported electrochemical sensor (LOD = 3 oocysts per mL) using the same procedure.58,160 However, since Cryptosporidium oocysts are directly adsorbed onto the substrate, the method requires cleaning and purification steps to minimize nonspecific adsorption.
A quartz glass plate was utilized in the sandwich ELISA where the plate was treated with 3-glycidoxypropyl methyl-diethoxysilane (GPTMS) to immobilize the anti-oocyst monoclonal antibody (mAb).159 Then, the core–shell Ag2S@silica nanospheres were functionalized with the mAb and employed to recognize the target oocysts captured on the surface of the quartz glass plates. The photoluminescence responses produced by the core–shell Ag2S@silica nanospheres' inherent properties were measured using UV-visible spectroscopy to quantify the captured oocysts. Both the previously described immuno-dot blot assay and this method exhibited the same level of sensitivity. However, the latter method requires two antibodies and a complex material synthesis protocol, in contrast to the relatively simple nano-conjugation process employed in the dot blot assay process.159,160
Integrating colorimetric sensors with smartphones enables a direct interface, enhancing user-friendliness and portability of the sensor. Moreover, it facilitates the monitoring of environmental samples in remote locations and the seamless transmission of data to a central database, enabling quick decision-making. Luka et al.161 used this feature of the colorimetric sensors and developed a portable smartphone-based Cryptosporidium RNA detection system (Fig. 5(A)). They used the target-induced color change of AuNPs (gold nanoparticles). Initially, thiolated oligonucleotides were used to modify the AuNPs, which then underwent hybridization with the target in a 3D-printed hydrophobic circular sensing area on a glass slide. Upon recognition of the target by the AuNPs probe, an RNA-linked oligonucleotide network formed, leading to a change in the surface plasmon of the AuNPs. This resulted in a noticeable color change, which was explained elsewhere, specifically for a red-to-blue shift.162 The sensor demonstrated the capability to detect Cryptosporidium RNA at concentrations as low as 5 μM, without requiring PCR amplification. However, it should be noted that the sensor required purified nucleic acid from a concentrated sample, which limits its suitability for point-of-need (PON) applications.161
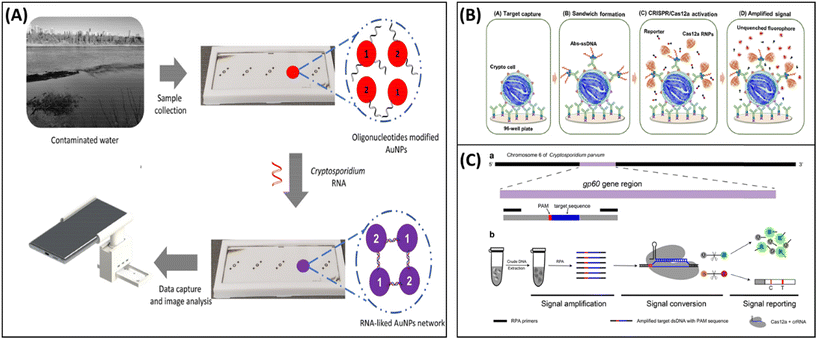 |
| Fig. 5 (A) Schematic illustration of label/PCR-free sensing platform by oligonucleotide modified AuNPs (reprinted with permission from ref. 161). (B) CRISPR-based sandwich ELISA (reprinted with permission from ref. 170) and (C) (a) showing the RPA primer binding site with PAM location in GP 60 gene (b) CRISPR-RPA dual mode (fluorescence and colorimetry) dual-mode detection of gp60 gene-based assay (reprinted with permission from ref. 53). | |
4.2.2 CRISPR-based detection.
CRISPR (clustered regularly interspaced short palindromic repeats) and CRISPR-associated (Cas) proteins have garnered significant attention for disease diagnosis and environmental sample monitoring.163,164 They have been found to cleave nucleic acid in a specific sequence, while certain Cas proteins, such as Cas12a, Cas12b, Cas13a, and Cas14, also exhibit nonspecific trans-cleavage activity.165–168 Both cis- and trans-cleavage activities have been utilized to detect pathogens, including Cryptosporidium oocysts.53,169,170 Li et al.170 developed a sandwich immunoassay based on a 96-well plate ELISA (Fig. 5(B)) to detect C. parvum. The ELISA plate was coated with anti-Cryptosporidium antibodies to capture Cryptosporidium oocysts. Then, an anti-Cryptosporidium antibody conjugated with single-stranded (ss) DNA was introduced to recognize the bound Cryptosporidium. The addition of a CRISPR/Cas12a reaction mixture triggered the activation of Cas12a ribonucleoprotein (RNP) and led to collateral trans-cleavage, releasing the fluorophore from the quencher. Consequently, the platform achieved the detection sensitivity of a single C. parvum oocyst. The authors emphasized that this assay is suitable for detecting Cryptosporidium in diluted mud samples. However, it should be noted that the cost associated with a CRISPR-based system is high despite its high sensitivity.
Very recently, Yu et al.53 reported a lateral flow assay (LFA) device utilizing the Cas12a/crRNA trans-cleavage system, referred to as ReCTC (trans-cleavage). According to their report, a specific region of the gp60 gene was first amplified using recombinase polymerase amplification (RPA), followed by Cas12a/crRNA trans-cleavage. To enable on-site diagnosis of C. parvum, the LFA was based on the cleavage of a specially designed FAM-TTATT-biotin single-stranded DNA (ssDNA) reporter. The FAM-TTATT-biotin ssDNA reporter has the ability to bind specifically to an anti-FITC antibody that is conjugated with AuNPs, forming a complex. In this assay design, if the ssDNA in the reporter remains intact and is not degraded by FnCas12a, the complex can be captured by the biotin ligand fixed at the control line. However, if the ssDNA is degraded, biotin is released from the complex. In this case, the complex without biotin cannot be captured by the biotin ligand at the control line. Instead, it can be captured by the IgG antibody at the test line. During the assay, the ReCTC reaction system is incubated for 1 hour. After that, it is mixed with ddH2O and loaded onto the LFS pad. The mixture is then incubated at room temperature for a short period of time. Finally, the LFA strips are photographed using a smartphone camera. This method has the capability to detect even a single copy of the cloned C. parvum gp60 gene without the need for tedious extraction of genomic DNA. Importantly, it exhibits no cross-reactivity with other subtypes of C. parvum or other common enteric parasitic protozoa.53
5. On-chip/microfluidics integrated sensor
Microfluidic devices offer the advantage of integrating multiple components, allowing for the occurrence of multiple chemical processes within a single device. This system integration is particularly beneficial for sample isolation, reducing errors caused by human contact and potential contamination, while also improving operational safety. By centralizing all processes within a single device, the time required for sample processing can be significantly reduced, enabling rapid analysis. It has to be considered that the application of microfluidics application to environmental samples often fails due to clogging from suspended colloidal particles or unwanted microbes of bigger sizes than the intended ones.171 Besides, challenges arise when processing large sample volumes (>10 L) to efficiently identify Cryptosporidium oocysts using microfluidic devices, as these devices typically handle sample volumes ranging from μL to mL. Therefore, a pre-enrichment step becomes necessary before loading the sample into the main device. Alternatively, direct sample loading can be achieved if the devices are modified with a filtration system or designed for post-filtrate material analysis. An alternative and improved method to the IMS coupled direct immunofluorescence assay (DFA-IMS) was reported by Taguchi et al.57 They utilized cone-shaped microcavities (2 μm and 10 μm diameters on the top and bottom surfaces, respectively) on a special-use stainless 304 (SUS) wafer known as SUS micromesh. When a sample is injected into the microfluidic channel, oocysts are trapped in the micromesh due to the negative pressure applied by adjusting the vacuum at the bottom of the micromesh. Each cavity in the micromesh accommodates only a single oocyst, ensuring aggregation-free conditions and assisting in parasite quantification. This system minimizes non-specific adsorption through the continuous flow of simple antibody solutions, outperforming DFA-IMS by recovering 93.1% of Cryptosporidium oocysts from tap water compared to DFA-IMS's 90.5%. The system offers a shorter detection time (1 hour) compared to DFA-IMS (2.5 hours) and simplified steps, making it an attractive alternative.57
Huang et al.172,173 reported a label-free method with microfluidic multi-angle laser scattering, which is relatively rapid (approximately 30 minutes). Efforts have also been made to sort and pre-concentrate Cryptosporidium using electrohydrodynamic concentration,174,175 dielectrophoresis-mediated contact-less separation of Cryptosporidium oocysts,176–178 disposable microfluidic micromixers179 and hydrodynamic trapping-based microscopy.178,180 Each method has its advantages: micromixers showed capture efficiency up to 96%, exceeding that of IMS,162 hydrodynamic trappings demonstrated promise in art-effect free imaging,179 hydrodynamic trappings showed promise in art-effect free imaging,180 and dielectrophoretic separation can differentiate between oocysts.176–178 However, precise fluid control is necessary for optimal functioning, suggesting that these methods are unlikely to be suitable for PON testing. The electrohydrodynamic and dielectrophoretic systems require perfectly buffered conditions to create a dipole within Cryptosporidium, which is likely to be affected by ions from filter-eluted samples.
Instead of detecting the whole Cryptosporidium oocyst, Angus et al.181 focused on detecting COWP (Cryptosporidium oocyst wall protein) using a microfluidic chip coupled with a miniature spectrophotometer. This system utilizes Mie light scattering to detect the immuno-agglutination of COWP protein to antibody-conjugated beads. However, the size of the oocyst (4–5 μm) is not ideal for this type of light scattering. To overcome this, the sample (2 mL) was sonicated to release the target protein, such as COWP. Subsequently, 15 μL of the sonicated sample and antibody-conjugated beads were introduced into two inlets, and a syringe pump created negative pressure, drawing them into the microfluidic channel where immuno-agglutination occurred. The limit of detection for this assay was 10 oocysts per mL of water. Considering the small amount of sample used (15 μL from a 2 mL sample) for analysis, this sensitivity corresponds to detecting sub-single-oocyst levels per sample. The authors envisioned that with proper filtration, this method could potentially detect ≤1 oocyst in a large volume of water sample (e.g., 10 L), which is comparable to the EPA method.36,181
Microfluidic-based nanosensors have garnered attention for their ability to isolate and detect target nucleic acids on-chip. Nucleic acid sequence-based amplification (NASBA) has proven to be a powerful tool for on-chip analysis of Cryptosporidium mRNA.138,182,183 In a recent study, Reinholt et al.182 developed a microfluidic system where mRNA isolation and NASBA-based amplification were conducted in a straight channel (Fig. 6(B)). They explored different surface chemistries and found that polyamidoamine dendrimers provided the best performance for both isolation and in situ NASBA reaction. To detect the amplified NASBA, a Lateral Flow Assay was employed. The NASBA product was hybridized with a reporter probe attached to sulforhodamine B-encapsulating liposomes, and the resulting solution flowed vertically to form a sandwich with C. parvum capture probes, generating a colorimetric signal. This system successfully isolated and amplified mRNA from 30 C. parvum oocysts. This sensitivity is comparable to the sensitivity of the NASBA reaction performed in standard laboratory benchtop devices for molecular amplification. The high immobilization efficiency of the thymidine oligonucleotide oligo (dT)25 on the PAMAM dendrimers' surface contributed to the device's sensitivity by enabling maximum mRNA capture. However, this device relies on a separate detection system for NASBA products.182,184 In a previous study, Nugen et al.183 fabricated a polymethyl methacrylate (PMMA) fluidic device with an integrated electrochemical module containing interdigitated ultramicroelectrode arrays (IDUA) (Fig. 6(C)). NASBA-based amplification of hsp 70 mRNA was performed outside of the PMMA chip, and the product was mixed with microbeads, potassium ferri/ferrocyanide-encapsulated liposomes, and a hybridization solution. The mixture was then pumped in a reciprocating motion through the PMMA chip for efficient mixing and hybridization on magnetic beads. Finally, the magnetic beads were accumulated on the IDUA by placing an external magnet, followed by the lysis of the liposomes with a detergent to release the electroactive substances. Unlike the previous method, the detection step was performed on-chip.182,183 Both of these methods do not constitute fully integrated systems since the processing and detection steps were conducted externally to the chip. However, by performing specific tasks outside the chip, these systems offer simplicity and efficiency while remaining within the scope of the designed fluidic chip.
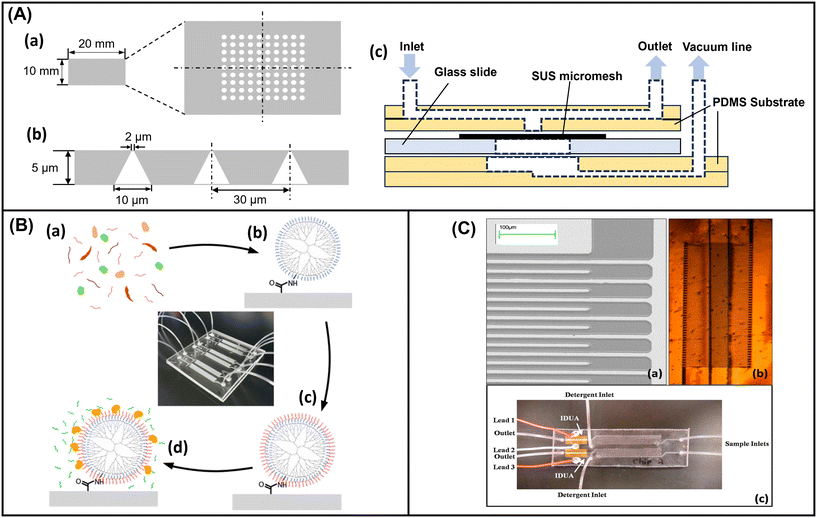 |
| Fig. 6 (A) (a) Special use stainless 304 (SUS) micromesh, (b) cross-sectional view of cone-shaped cavity of micromesh, and (c) microfluidic SUS micromesh assembly for Cryptosporidium trapping (redrawn and reprinted with permission from ref. 57). (B) Schematic of mRNA isolation in a straight microfluidic channel ((a) Cryptosporidium lysate, (b) PAMAM dendrimer for surface modification of microfluidic channel, (c) isolated mRNA in dendrimer surface) for (d) in situ NASBA reaction (reprinted with permission from ref. 182), and (C) (a) SEM of IDUA and PDMS channel, (b) a PMMA sheet containing a hot-embossed channel was then bonded to the PMMA containing the IDUA, and (c) assembled chip containing two channels (reprinted with permission from ref. 183). | |
6. Genotyping and subtyping of Cryptosporidium spp.
Genotyping is a molecular approach used to differentiate samples at the species level, such as distinguishing between C. hominis and C. parvum by analyzing DNA sequence variations in specific genes. This approach is distinct from subtyping, which focuses on variations within a species. While several molecular tools have been developed, there is currently no standardized procedure.185 Generally, the 18S rRNA and gp60 gene are extensively used as the optimal markers for speciation and subtyping, respectively.68 Complete 18S rRNA gene is around 1620–1746 bp (AY954884.1, AY954885.1) long. Within this gene, three domains exhibit significant polymorphism, and one of these domains is key to distinguishing various Cryptosporidium species. Specifically, the discriminating domain lies between nucleotide positions 479–928 (with reference to C. felis AF108862).186 Nested PCR is employed to amplify this region, generating amplicons of approximately 830 base pairs in size. Following amplification of the ∼830-bp segment of the 18S rRNA gene through nested PCR, the resulting product is digested with two restriction enzymes (e.g., SspI and VspI). The resulting restriction pattern is then compared using gel electrophoresis, as described by Xiao et al., to determine whether it corresponds to C. parvum or C. hominis.187,188 In cases where the observed restriction pattern does not match any previously reported patterns, the amplicons are sequenced and compared to reference sequences to identify the genotype.189 Nested PCR is highly sensitive and capable of amplifying DNA from a single purified oocyst, enabling the detection of different species by analyzing PCR product fragments.190 However, PCR amplification tends to favor the most abundant species, making it challenging to identify rare genotypes in cases of mixed infections. In such instances, deep sequencing of samples can reveal the presence of relatively rare genotypes within the mixed infections.
Historically, PCR-RFLP and more recently, qPCR with high resolution melting curve, Sanger sequencing of qPCR products have been extensively used for species identification and genotyping Cryptosporidium spp. from various sample types, including raw water, drinking water, stool, soil, vegetables, and more.191–194 Besides 18S rRNAgene, COWP and hsp70, have been explored for genotyping purposes. However, the multi-copy nature and semi-conserved/hypervariable characteristics of the 18S rRNA gene make it ideal for designing genus-specific primers.188,195,196 It is important to note that the 18S rRNA gene-based genotyping method cannot differentiate intra-species variations. In order to address this limitation, Strong et al.197 introduced gp60 (also known as gp40/15) gene-based subtyping, which offers high genetic resolution for efficient characterization of C. parvum and C. hominis. This gene is now commonly used to analyze transmission dynamics, including sources of infection, genetic diversity, and host adaptation.198 The gp60 gene contains a variable number of tandem repeats of serine coding trinucleotides TCA, TCG, or TCT at the 5′ end, which determines the subtypes. At the 3′ end, there is a repetitive sequence and a conserved region (Fig. 7) that distinguishes between C. hominis and C. parvum. According to the gp60 nomenclature, the subtype is indicated by a combination of the subtype family designation (Ia, Ib, Id, Ie, If for C. hominis, and IIa, IIb, IIc, IId for C. parvum) followed by the number of trinucleotide repeats (represented as A, G, or T for TCA, TCG, or TCT, respectively) in the gp60 gene. For example, IbA10G2 represents C. hominis with ten copies of TCA repeats and two copies of TCG repeats in the gp60 gene. Certain subtypes of C. parvum and C. hominis contain additional repetitive sequences, represented as ‘R’, after the trinucleotide repeats. For instance, IaA23R4 indicates a C. hominis subtype with four ‘AA/GGACGGTGGTAAGG’ repeats, while IIaA15G2R2 belongs to C. parvum with two ‘ACATCA’ repeats.64,195,199,200 The gp60 subtyping method has proven useful for allelic discrimination and tracing back to sources and transmission routes. It is applicable to the commonly found and closely related species of C. parvum and C. hominis.199,201 The recombination between different stages of the life cycle contributes to heterogeneity, as seen in the intragenic linkage disequilibrium of natural C. parvum populations.202,203 More recently, Huang et al.204 reported a complex population structure of C. hominis which is largely inconsistence with gp60 subtypes and supports the fact that gp60 is the region of high recombination. Thus raised a concern of using gp60 as a population marker.205 Environmental samples appear to harbor Cryptosporidium spp. other than C. parvum and C. hominis such as C. felis, C. cuniculus, C. meleagridis, C. canis, C. ubiquitum, which have the potential to infect human.206 As mentioned earlier, gp60 is limited to C. parvum and C. hominis, environmental samples need alternative genotyping methods. Efforts were made to establish gp60-based genotyping for C. felis. However, it turned out to be unsuccessful and suggested currently available low-resolution markers (18S rRNA, COWP and HSP70) for genotyping.207 Therefore, to gain more detailed and discriminatory information about the subtypes multilocus sequence typing (MLST) or multilocus genotyping (MLG) proved to be suitable.198
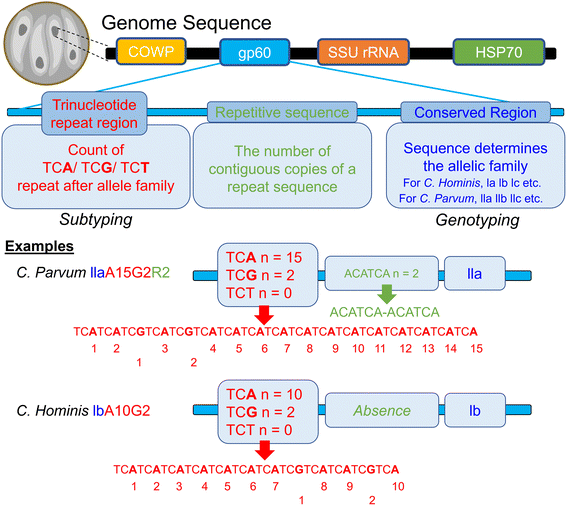 |
| Fig. 7 Derivation of gp60 nomenclature for C. hominis and C. parvum (modified and redrawn with permission from ref. 199). | |
Researchers have studied various loci that primarily contain microsatellites and minisatellite repeats, also known as variable-number tandem repeats (VNTR), for MLG. Fragment sizing or sequence analysis has been employed for this purpose. A systematic review has already been published, which reported a combination of 55 VNTR loci, although the rationale for selecting these loci was not explained.185 In a study by Nadon et al.208 criteria were defined for selecting loci for multilocus VNTR analysis. According to their report, the loci should have 100% conserved sequences as flanking regions, be distributed across the chromosome as extensively as possible, consist of perfect and homogeneous repeats of at least 5 base pairs, and should not exhibit any apparent insertions or deletions in the repeat unit.208 Pérez-Cordón et al.209 identified 28 loci from whole-genome sequences of C. parvum that met these criteria and were deemed suitable for in vitro evaluation. Recently, Robinson et al.198 re-evaluated these VNTR loci and proposed an improved set of loci. Compared to the currently used gp60-based genotyping method (which has a discriminatory power of 0.74), the newly proposed VNTR loci exhibited a superior discriminatory power of 0.99.
Besides monitoring the Cryptosporidium spp., whole genome or target amplicon NGS is crucial for identifying new markers for detecting and analyzing the diversity of this species. As mentioned earlier, NGS can resolve each species present in a DNA extract, it helps in finding the intra-host genetic diversity of gp60 subtypes. Grinberg et al.210 observed ten gp60 allelic subtypes by NGS in two Cryptosporidium-positive fecal samples in New Zealand, previously thought infections were due to a rare gp60 subtype (C. parvum IIaA21G4R1) during an outbreak in 2006. Similarly, Zahedi et al.148 also observed gp60 diversity in C. parvum in cattle and rabbits from Australia and China, but only a single subtype (IbA10G2) of C. hominis in Australian Kangaroo. Although subtype population structure is subjected to vary depending on the hosts and region, this observation indicates population structures of C. parvum and C. hominis are panmictic and clonal in gp60 allege respectively circulating in that region. To improve the genotyping resolution of C. hominis IbA10G2, Beser et al.211 analyzed 17 C. hominis (including nine C. hominis IbA10G2 isolate) whole genome sequencing data and identified 10 single nucleotide variation (SNV) in nine loci of sizes 268–349, spanning in 6 chromosomes. This typing panel has been validated against one-step PCR-based amplicon sequencing of 44 clinical IbA10G2 samples and three publicly available whole genome sequences. By setting a criterion of minimum 20× coverage of each locus, this study was able to group all the IbA10G2 into 10 different sequence types (named ST1–10). Despite having moderate sample sizes from Sweden, Spain, the USA, the UK, Peru, the UAE, Venezuela, Thailand, Tunisia, and Estonia, the true potential of such a marker panel can only be realized if samples from each continent including low- or middle-income countries were included.
7. Discussions and current challenges
Despite continuous efforts spanning over two decades to improve and advance the successful isolation of oocysts from water, there are still persistent challenges that need to be addressed.
(i) Extremely low abundance and recovery
The current gold standard detection approach, USEPA 1623.1, falls short of achieving 100% recovery, meaning that it cannot detect all the pathogens present in the collected water sample. Incomplete elusion of oocysts during the concentration stage often leads to a recovery rate of less than 15%, while in the later stage of IMS-FA, a recovery rate of over 95% is commonly achieved.212,213 The actual recovery rate varies depending on water quality and turbidity, but USEPA 1623.1 considers a 38% recovery rate acceptable. Depending on the sample to be analysed, and the actual concentration of the oocysts in a sample, this recovery will allow to detect or even quantify the oocysts. Given that high-quality nucleic acid preparation is available from a low number of oocysts, down to single oocysts can be detected (LAMP48) and quantified (ddPCR, nested-PCR).129 It is evident that achieving a high recovery rate during the concentration stage is crucial for successfully detecting oocysts in the later stage. It should be noted that the concentration of Cryptosporidium in surface water is typically low, usually around 0.1/L or 1 in 10 L. For example, if the oocyst concentration at a sampling point in a stream is 1 in 10 L, many 10 L volumes passing that point would not contain a single organism, while some may contain more than one. This variability greatly impacts the reproducibility of the analysis. In the worst-case scenario, it may result in false-negative identifications, increasing the relative risk of a potential outbreak through human exposure to the pathogen. While increasing the volume of water used for sampling could potentially address this issue, it is impractical as it often leads to filter clogging, depending on the water quality. Use of engineered polymer materials to prevent adhesion of oocyst to the filter/membrane,214 efficient design of filtration such as incorporating ultrasound source in filter94 may increase the oocyst recovery is the by eluting more trapped oocysts. Indeed, this cannot solve the issue of filter clogging when more water needs to be filtered. In this case, a passive sampling procedure with molecular detection might be suitable which has been reported for SARS-CoV-2 in wastewater surveillance.215
(ii) Cryptosporidium viability
In general, Cryptosporidium oocysts can remain viable and infectious for months under environmental conditions and more concerning during drinking and recreational water treatment processes. However, empty oocysts had been reported in varying degrees in environmental samples.107 If the sample is not analysed quickly after receiving, depending on the storage condition and length, sporozoites may be released from the oocysts.107 Such oocysts will generate a positive fluorescent signal. Vital dye staining or further, CC-PCR based infectivity test will solve this issue to some extent. Each of these has limitations, such as the study showed that fresh oocysts from feces were importable to vital dye216 and CC-PCR is not suitable to get a result within a day. To address this issue, the implementation of automatic image analysis for detection and viability testing holds promise. Not only could it help solve the problem, but it could also reduce the time-consuming nature of the analysis.217
(iii) Insufficient available biomarkers
The anti-Cryptosporidium monoclonal antibody combined with IMS is currently used as a routine method for isolating Cryptosporidium from water samples.37,74,79,97,117 However, using antibodies for isolation and detection does not allow for differentiation of the genus or subspecies of Cryptosporidium. As discussed earlier, previous studies have shown that C. parvum isolates from various sources, such as humans, calves, pigs, dogs, mice, ferrets, marsupials, and a monkey, exhibit significant genetic diversity, and the distribution of different genotypes in surface water can vary. While the 18S rRNA marker is useful for species identification, it can also be utilized, along with additional markers, for identifying subtypes primarily within C. parvum and C. hominis.218–220 Certain markers are also limited to analyzing local geographic diversity, and the global population structure may vary.218 Organoid model for infection studies,221 hollow fibre technology for continuous culture,222 single oocyst sequencing223 may provide useful information regarding post-meiotic precise genetic characteristics. Single-cell genomics for Cryptosporidium is quite a new and growing branch and ensuring a high-quality reference genome of each species will decipher new ways to understand this species.
(iv) Variability of molecular characteristics (specificity)
In addition to the scarcity of available markers across species, genetic variability has been observed in the sexual life of Cryptosporidium due to recombination.224 This variability, including SNPs, insertions, or deletions within the markers, could potentially cause the multi-locus typing approach to fail in certain cases. Therefore, it is crucial to sequence all 8 chromosomes of Cryptosporidium spp. directly collected from water samples worldwide and develop location-specific markers for multi-locus typing. However, establishing a clonal population from a single sporozoite may help to understand the virulence and transmissibility of each subtype.225
8. Conclusion and future direction
In this review, we have discussed the economic impact of Cryptosporidium outbreaks from a public health perspective and provided a comprehensive overview of both conventional and state-of-the-art detection strategies for this parasite. Currently, the USEPA 1623.1 method is considered the gold standard protocol for Cryptosporidium spp. detection and is an integral part of regulated quality control to minimize risks and potential Cryptosporidium outbreaks. However, there is an urgent need for alternative methods that can offer a faster, cheaper, and simpler platform for the isolation, detection, and genotyping of Cryptosporidium, addressing the limitations of the USEPA 1623.1 method. To improve the performance of isolation and detection, one approach is to incorporate filtration and elution in a single device with automated operation and control, which would simplify sample processing, minimize steps, and reduce overall time. However, many of these proof-of-concept studies need further development and validation to achieve a high level of consistency, sensitivity, and specificity to be considered by water authorities and regulators. Another potential advancement is the use of magnetic micro- and nanoparticles directly in the water sample to capture Cryptosporidium oocysts before filtration, presenting a next-generation challenge for a rapid and integrated detection system. Achieving proper sample circulation, effective mixing of magnetic particles, and efficient recovery of oocysts pose bio-engineering challenges. To achieve selective capture and detection of the whole pathogen, monoclonal antibodies are essential. As mentioned earlier, unless molecular signatures are tested, monoclonal antibodies cannot differentiate between species. DNA/RNA aptamers could offer hope in such cases. Aptamers have gained attention as alternative biorecognition elements,56,226–228 but systematic screening of aptamers specific to different species/genotypes of Cryptosporidium is currently lacking.
Recent efforts have indicated that the utilization of nanoparticle-coupled nucleic-acid-based detection techniques holds potential for enhancing the sensitivity of oocyst isolation and detection. An example of such a technique is the colorimetric assay developed by Luka et al.161 which utilizes the aggregation of AuNPs that are linked to specific oligonucleotides to capture Cryptosporidium RNA. This approach presents a simple, label-free, and PCR-free method of detection. It is however crucial to carefully control nonspecific adsorption to ensure accurate and reliable results. In addition to nanoparticle-coupled nucleic-acid-based techniques, implementing artificial intelligence (AI) and machine learning-based automatic image processing can have a transformative impact on parasite detection. By minimizing artifacts and improving the accuracy of miniaturized systems, these technologies can revolutionize the field. They have the potential to enhance the efficiency and precision of detecting parasites, providing valuable insights for diagnosis and research.
Genotyping and subtyping of Cryptosporidium present significant challenges that need to be addressed. This is particularly important in developing new sensors for Cryptosporidium since species are host-specific, and in most cases, only two species are infectious to humans. The difficulty lies in the fact that Cryptosporidium spp. share an almost identical genome, making sequencing the only practical solution for analysis. However, the cost and time required for sequencing pose limitations. Despite this, multi-locus genotyping, along with gp60-based genotyping, has shown partial success in speciating isolates and tracing oocysts back to their host/source. Currently, the understanding of cryptosporidiosis epidemiology is fragmented due to the lack of genotype comparisons across wide geographical areas and inconsistencies in recording diagnostic and epidemiological data. Informative genotype comparisons and meta-analyses encompassing multiple surveys could potentially establish a standard genotyping scheme and enhance our understanding of cryptosporidiosis epidemiology.
Conflicts of interest
There are no conflicts to declare.
Acknowledgements
The authors acknowledge the higher degree research scholarships, GUIPRS and GUPRS Scholarships, to R. G. M. from Griffith University.
References
- A. Zahedi, A. Paparini, F. Jian, I. Robertson and U. Ryan, Public health significance of zoonotic Cryptosporidium species in wildlife: Critical insights into better drinking water management, Int. J. Parasitol. Parasites Wildl., 2016, 5, 88–109 CrossRef PubMed
.
- U. M. Ryan, Y. Feng, R. Fayer and L. Xiao, Taxonomy and molecular epidemiology of Cryptosporidium and Giardia – a 50 year perspective (1971–2021), Int. J. Parasitol., 2021, 51, 1099–1119 CrossRef CAS PubMed
.
- D. Mondal, R. Haque, R. B. Sack, B. D. Kirkpatrick and W. A. Petri, Attribution of Malnutrition to Cause-Specific Diarrheal Illness: Evidence from a Prospective Study of Preschool Children in Mirpur, Dhaka, Bangladesh, Am. J. Trop. Med. Hyg., 2009, 80, 824–826 CrossRef PubMed
.
- S. Dong, Y. Yang, Y. Wang, D. Yang, Y. Yang, Y. Shi, C. Li, L. Li, Y. Chen, Q. Jiang and Y. Zhou, Prevalence of Cryptosporidium Infection in the Global Population: A Systematic Review and Meta-analysis, Acta Parasitol., 2020, 65, 882–889 CrossRef PubMed
.
- I. A. Khalil, C. Troeger, P. C. Rao, B. F. Blacker, A. M. Brown and D. V. Colombara,
et al., Morbidity, mortality, and long-term consequences associated with diarrhoea from Cryptosporidium infection in children younger than 5 years: a meta-analyses study, Lancet Global Health, 2018, 6, e758–e768 CrossRef PubMed
.
- W. Checkley, R. H. Gilman, L. D. Epstein, M. Suarez, J. Fernando Diaz, L. Cabrera, R. E. Black and C. R. Sterling, Asymptomatic and Symptomatic Cryptosporidiosis: Their Acute Effect on Weight Gain in Peruvian Children, Am. J. Epidemiol., 1997, 145, 156–163 CrossRef CAS PubMed
.
- D. I. Guerrant, S. R. Moore, A. A. M. Lima, P. D. Patrick, J. B. Schorling and R. L. Guerrant, Association of early childhood diarrhea and cryptosporidiosis with impaired physical fitness and cognitive function four-seven years later in a poor urban community in northeast Brazil, Am. J. Trop. Med. Hyg., 1999, 61, 707–713 CrossRef CAS PubMed
.
- J. A. Platts-Mills, S. Babji, L. Bodhidatta, J. Gratz, R. Haque and A. Havt,
et al., Pathogen-specific burdens of community diarrhoea in developing countries: a multisite birth cohort study (MAL-ED), Lancet Global Health, 2015, 3, e564–e575 CrossRef PubMed
.
- P. G. Ashigbie, S. Shepherd, K. L. Steiner, B. Amadi, N. Aziz, U. H. Manjunatha, J. M. Spector, T. T. Diagana and P. Kelly, Use-case scenarios for an anti-cryptosporidium therapeutic, PLoS Neglected Trop. Dis., 2021, 15, e0009057 CrossRef PubMed
.
-
Centers for Disease Control and Prevention, Nitazoxanide, 2015, http://www.cdc.gov/parasites/crypto/nitazoxanide.html, (accessed 08 Jan 2022) Search PubMed.
- B. Amadi, M. Mwiya, J. Musuku, A. Watuka, S. Sianongo, A. Ayoub and P. Kelly, Effect of nitazoxanide on morbidity and mortality in Zambian children with cryptosporidiosis: A randomised controlled trial, Lancet, 2002, 360, 1375–1380 CrossRef PubMed
.
- B. Amadi, M. Mwiya, S. Sianongo, L. Payne, A. Watuka, M. Katubulushi and P. Kelly, High dose prolonged treatment with nitazoxanide is not effective for cryptosporidiosis in HIV positive Zambian children: A randomised controlled trial, BMC Infect. Dis., 2009, 9, 1–7 CrossRef PubMed
.
- M. S. Love and R. K. M. Choy, Emerging treatment options for cryptosporidiosis, Curr. Opin. Infect. Dis., 2021, 34, 455–462 CrossRef PubMed
.
- J. Tandel, E. D. English, A. Sateriale, J. A. Gullicksrud, D. P. Beiting, M. C. Sullivan, B. Pinkston and B. Striepen, Life cycle progression and sexual development of the apicomplexan parasite Cryptosporidium parvum, Nat. Microbiol., 2019, 4, 2226–2236 CrossRef PubMed
.
- E. E. Tyzzer, An extracellular Coccidium, Cryptosporidium Muris (Gen. Et Sp. Nov.), of the gastric
Glands of the Common Mouse, J. Med. Res., 1910, 23, 487–510.3 CAS
.
- A. S. Collick, E. A. Fogarty, P. E. Ziegler, M. T. Walter, D. D. Bowman and T. S. Steenhuis, Survival of Cryptosporidium parvum Oocysts in Calf Housing Facilities in the New York City Watersheds, J. Environ. Qual., 2006, 35, 680–687 CrossRef CAS PubMed
.
- S. M. Dorner, P. M. Huck and R. M. Slawson, Estimating Potential Environmental Loadings of Cryptosporidium spp. and Campylobacter spp. from Livestock in the Grand River Watershed, Ontario, Canada, Environ. Sci. Technol., 2004, 38, 3370–3380 CrossRef CAS PubMed
.
- C. P. Chauret, C. Z. Radziminski, M. Lepuil, R. Creason and R. C. Andrews, Chlorine Dioxide Inactivation of Cryptosporidium parvum Oocysts and Bacterial Spore Indicators, Appl. Environ. Microbiol., 2001, 67, 2993–3001 CrossRef CAS PubMed
.
- S. Jain, T. G. Costa Melo, S. S. Dolabella and J. Liu, Current and emerging tools for detecting protozoan cysts and oocysts in water, TrAC - Trends, Anal. Chem., 2019, 121, 115695 CAS
.
-
U.S. Environmental Protection Agency, Cryptosporidium: Drinking Water Health Advisory, 2001, https://www.epa.gov/sites/default/files/2015-10/documents/cryptosporidium-report.pdf, (accessed 08 Jan 2024) Search PubMed.
- W. R. M. Kenzie, W. L. Schell, K. A. Blair, D. G. Addiss, D. E. Peterson, N. J. Hoxie, J. J. Kazmierczak and J. P. Davis, Massive Outbreak of Waterborne Cryptosporidium Infection in Milwaukee, Wisconsin: Recurrence of Illness and Risk of Secondary Transmission, Clin. Infect. Dis., 1995, 21, 57–62 CrossRef PubMed
.
- A. Efstratiou, J. E. Ongerth and P. Karanis, Waterborne transmission of protozoan parasites: Review of worldwide outbreaks - An update 2011–2016, Water Res., 2017, 114, 14–22 CrossRef PubMed
.
- C. L. Chappell, P. C. Okhuysen, R. Langer-Curry, G. Widmer, D. E. Akiyoshi and S. Tzipori, Cryptosporidium hominis: experimental challenge of healthy adults, Am. J. Trop. Med. Hyg., 2006, 75, 851–857 CrossRef CAS PubMed
.
- H. Erbert, P. Ont, Y. L. C. Happell, H. R. S. Terling, A. C. O. Khuysen, J. Oan, B. R. Ose, W. Alter and J. Akubowski, The Infectivity of Cryptosporidium parvum in Healthy Volunteers, N. Engl. J. Med., 1995, 332, 855–859 CrossRef PubMed
.
-
World Health Organization, Risk Assessment of Cryptosporidium in Drinking Water, 2009, https://www.who.int/publications/i/item/WHO-HSE-WSH-09.04, (accessed 08 Jan 2024) Search PubMed.
-
World Health Organization, Guidelines for drinking-water quality, 4th edn, incorporating the 1st addendum, 2017, https://www.who.int/publications/i/item/9789241549950, (accessed 08 Jan 2024) Search PubMed.
- P. S. Corso, M. H. Kramer, K. A. Blair, D. G. Addiss, J. P. Davis and A. C. Haddix, Costs of Illness in the 1993 Waterborne Cryptosporidium Outbreak, Milwaukee, Wisconsin, Emerging Infect. Dis., 2003, 9, 426–431 CrossRef PubMed
.
- A. Chyzheuskaya, M. Cormican, R. Srivinas, D. O'Donovan, M. Prendergast, C. O'Donoghue and D. Morris, Economic assessment of waterborne outbreak of cryptosporidiosis, Emerging Infect. Dis., 2017, 23, 1650–1656 CrossRef PubMed
.
- R. M. B. Franco, R. Rocha-Eberhardt and R. C. Neto, Occurrence of Cryptosporidium oocysts and Giardia cysts in raw water from the Atibaia river, Campinas, Brazil, Rev. Inst. Med. Trop. Sao Paulo, 2001, 43, 109–111 CrossRef CAS PubMed
.
- K. M. Shepherd and A. P. Wyn-Jones, Evaluation of different filtration techniques for the concentration of Cryptosporidium oocysts from water, Water Sci. Technol., 1995, 31, 425–429 CrossRef
.
- S. Renoth, P. Karanis, D. Schoenen and H. M. Seitz, Recovery Efficiency of Cryptosporidium from Water with a Crossflow System and Continuous Flow Centrifugation: a Comparison Study, Zentralbl. Bakteriol., 1996, 283, 522–528 CrossRef CAS PubMed
.
- T. Wohlsen, J. Bates, B. Gray, P. Aldridge, S. Stewart, M. Williams and M. Katouli, The occurrence of Cryptosporidium and Giardia in the Lake Baroon catchment, Queensland, Australia, J. Water Supply: Res. Technol.--AQUA, 2006, 55, 357–366 CrossRef
.
- K. Helmi, S. Skraber, J.-B. Burnet, L. Leblanc, L. Hoffmann and H.-M. Cauchie, Two-year monitoring of Cryptosporidium parvum and Giardia lamblia occurrence in a recreational and drinking water reservoir using standard microscopic and molecular biology techniques, Environ. Monit. Assess., 2010, 179, 163–175 CrossRef PubMed
.
- Z. Matheson, T. M. Hargy, R. M. McCuin, J. L. Clancy and C. R. Fricker, An evaluation of the Gelman Envirochek® capsule for the simultaneous concentration of Cryptosporidium and Giardia from water, J. Appl. Microbiol., 1998, 85, 755–761 CrossRef CAS PubMed
.
- C. L. DiGiorgio, D. A. Gonzalez and C. C. Huitt, Cryptosporidium and Giardia Recoveries in Natural Waters by Using Environmental Protection Agency Method 1623, Appl. Environ. Microbiol., 2002, 68, 5952–5955 CrossRef CAS PubMed
.
- G. H. Kimble, J. E. Amburgey and V. R. Hill, Comparison of Hollow-Fiber Ultrafilters with Pleated Capsule Filters for Surface and Tap Water Samples Using U.S. EPA Method 1623, J. Environ. Eng., 2012, 138, 899–901 CrossRef CAS PubMed
.
- V. R. Hill, A. L. Polaczyk, A. M. Kahler, T. L. Cromeans, D. Hahn and J. E. Amburgey, Comparison of Hollow-Fiber Ultrafiltration to the USEPA VIRADEL Technique and USEPA Method 1623, J. Environ. Qual., 2009, 38, 822–825 CrossRef CAS PubMed
.
- E. R. Rhodes, L. F. Villegas, N. J. Shaw, C. Miller and E. N. Villegas, A Modified EPA Method 1623 that Uses Tangential Flow Hollow-fiber Ultrafiltration and Heat Dissociation Steps to Detect Waterborne Cryptosporidium and Giardia spp, J. Visualized Exp., 2012, e4177, DOI:10.3791/4177-v
.
-
A. M. Kahler and V. R. Hill, Detection of Cryptosporidium Recovered from Large-Volume Water Samples Using Dead-End Ultrafiltration, in Methods in Molecular Biology, Humana Press Inc., 2020, vol. 2052, pp. 23–41 Search PubMed
.
- Y. Feng, X. Zhao, J. Chen, W. Jin, X. Zhou, N. Li, L. Wang and L. Xiao, Occurrence, Source, and Human Infection Potential of Cryptosporidium and Giardia spp. in Source and Tap Water in Shanghai, China, Appl. Environ. Microbiol., 2011, 77, 3609–3616 CrossRef CAS PubMed
.
- P. Karanis and A. Kimura, Evaluation of three flocculation methods for the purification of Cryptosporidium parvum oocysts from water samples, Lett. Appl. Microbiol., 2002, 34, 444–449 CrossRef CAS PubMed
.
- F. Lora-Suarez, R. Rivera, J. Triviño-Valencia and J. E. Gomez-Marin, Detection of protozoa in water samples by formalin/ether concentration method, Water Res., 2016, 100, 377–381 CrossRef CAS PubMed
.
- U. Zuckerman and S. Tzipori, Portable continuous flow centrifugation and method 1623 for monitoring of waterborne protozoa from large volumes of various water matrices, J. Appl. Microbiol., 2006, 100, 1220–1227 CrossRef CAS PubMed
.
- J. A. Higgins, J. M. Trout, R. Fayer, D. Shelton and M. C. Jenkins, Recovery and detection of Cryptosporidium parvum oocysts from water samples using continuous flow centrifugation, Water Res., 2003, 37, 3551–3560 CrossRef CAS PubMed
.
- M. E. Warkiani, C. P. Lou and H. Q. Gong, Fabrication and characterization of a microporous polymeric micro-filter for isolation of Cryptosporidium parvum oocysts, J. Micromech. Microeng., 2011, 21, 035002 CrossRef
.
- M. E. Warkiani, C. P. Lou, H. B. Liu and H. Q. Gong, A high-flux isopore micro-fabricated membrane for effective concentration and recovering of waterborne pathogens, Biomed. Microdevices, 2012, 14, 669–677 CrossRef PubMed
.
- N. M. M. Pires and T. Dong, Recovery of Cryptosporidium and Giardia organisms from surface water by counter-flow refining microfiltration, Environ. Technol., 2013, 34, 2541–2551 CrossRef CAS PubMed
.
- P. Karanis, O. Thekisoe, K. Kiouptsi, J. Ongerth, I. Igarashi and N. Inoue, Development and preliminary evaluation of a loop-mediated isothermal amplification procedure for sensitive detection of Cryptosporidium oocysts in fecal and water samples, Appl. Environ. Microbiol., 2007, 73, 5660–5662 CrossRef CAS PubMed
.
- G. Garcés-Sanchez, P. A. Wilderer, H. Horn, J. C. Munch and M. Lebuhn, Assessment of the viability of Cryptosporidium parvum oocysts with the induction ratio of hsp70 mRNA production in manure, J. Microbiol. Methods, 2013, 94, 280–289 CrossRef PubMed
.
- A. J. Baeumner, M. C. Humiston, R. A. Montagna and R. A. Durst, Detection of viable oocysts of Cryptosporidium parvum following nucleic acid sequence based amplification, Anal. Chem., 2001, 73, 1176–1180 CrossRef CAS PubMed
.
- M. B. Esch, L. E. Locascio, M. J. Tarlov and R. A. Durst, Detection of viable Cryptosporidium parvum using DNA-modified liposomes in a microfluidic chip, Anal. Chem., 2001, 73, 2952–2958 CrossRef CAS PubMed
.
- J. T. Connelly, S. R. Nugen, W. Borejsza-Wysocki, R. A. Durst, R. A. Montagna and A. J. Baeumner, Human pathogenic Cryptosporidium species bioanalytical detection method with single oocyst detection capability, Anal. Bioanal. Chem., 2008, 391, 487–495 CrossRef CAS PubMed
.
- F. Yu, K. Zhang, Y. Wang, D. Li, Z. Cui, J. Huang, S. Zhang, X. Li and L. Zhang, CRISPR/Cas12a-based on-site diagnostics of Cryptosporidium parvum IId-subtype-family from human and cattle fecal samples, Parasites Vectors, 2021, 14, 1–10 CrossRef PubMed
.
- G. Luka, E. Samiei, S. Dehghani, T. Johnson, H. Najjaran and M. Hoorfar, Label-free capacitive biosensor for detection of Cryptosporidium, Sensors, 2019, 19, 258 CrossRef PubMed
.
- G. S. Luka, H. Najjaran and M. Hoorfar, On-chip-based electrochemical biosensor for the sensitive and label-free detection of Cryptosporidium, Sci. Rep., 2022, 12, 6957 CrossRef CAS PubMed
.
- A. Iqbal, M. Labib, D. Muharemagic, S. Sattar, B. R. Dixon and M. V. Berezovski, Detection of Cryptosporidium parvum oocysts on fresh produce using DNA aptamers, PLoS One, 2015, 10, e0137455 CrossRef PubMed
.
- T. Taguchi, A. Arakaki, H. Takeyama, S. Haraguchi, M. Yoshino, M. Kaneko, Y. Ishimori and T. Matsunaga, Detection of Cryptosporidium parvum oocysts using a microfluidic device equipped with the SUS micromesh and FITC-labeled antibody, Biotechnol. Bioeng., 2007, 96, 272–280 CrossRef CAS PubMed
.
- C. Thiruppathiraja, V. Saroja, S. Kamatchiammal, P. Adaikkappan and M. Alagar, Development of electrochemical based sandwich enzyme linked immunosensor for Cryptosporidium parvum detection in drinking water, J. Environ. Monit., 2011, 13, 2782–2787 RSC
.
- C. A. Yanta, K. Bessonov, G. Robinson, K. Troell and R. A. Guy, CryptoGenotyper: A new bioinformatics tool for rapid Cryptosporidium identification, Food Waterborne Parasitol., 2021, 23, e00115 CrossRef PubMed
.
- M. Heiges, H. Wang, E. Robinson, C. Aurrecoechea, X. Gao, N. Kaluskar, P. Rhodes, S. Wang, C. Z. He, Y. Su, J. Miller, E. Kraemer and J. C. Kissinger, CryptoDB: a Cryptosporidium bioinformatics resource update, Nucleic Acids Res., 2006, 34, D419–D422 CrossRef CAS PubMed
.
- M. Bouzid, P. R. Hunter, R. M. Chalmers and K. M. Tyler, Cryptosporidium pathogenicity and virulence, Clin. Microbiol. Rev., 2013, 26, 115–134 CrossRef CAS PubMed
.
- A. Guérin and B. Striepen, The Biology of the Intestinal Intracellular Parasite Cryptosporidium, Cell Host Microbe, 2020, 28, 509–515 CrossRef PubMed
.
- D. J. Pinto and S. Vinayak, Cryptosporidium: Host-Parasite Interactions and Pathogenesis, Curr. Clin. Microbiol. Rep., 2021, 8, 62–67 CrossRef PubMed
.
- Y. Feng, U. M. Ryan and L. Xiao, Genetic Diversity and Population Structure of Cryptosporidium, Trends Parasitol., 2018, 34, 997–1011 CrossRef CAS PubMed
.
- G. Certad, E. Viscogliosi, M. Chabé and S. M. Cacciò, Pathogenic Mechanisms of Cryptosporidium
and Giardia, Trends Parasitol., 2017, 33, 561–576 CrossRef CAS PubMed
.
- U. Ryan and N. Hijjawi, New developments in Cryptosporidium research, Int. J. Parasitol., 2015, 45, 367–373 CrossRef CAS PubMed
.
- U. Ryan, A. Paparini, P. Monis and N. Hijjawi, It's official – Cryptosporidium is a gregarine: What are the implications for the water industry?, Water Res., 2016, 105, 305–313 CrossRef PubMed
.
- A. Khan, J. S. Shaik and M. E. Grigg, Genomics and molecular epidemiology of Cryptosporidium species, Acta Trop., 2018, 184, 1–14 CrossRef CAS PubMed
.
- J. K. O'Leary, R. D. Sleator and B. Lucey, Cryptosporidium spp. diagnosis and research in the 21st century, Food Waterborne Parasitol., 2021, 24, e00131 CrossRef PubMed
.
- H. Bridle, M. Kersaudy-Kerhoas, B. Miller, D. Gavriilidou, F. Katzer, E. A. Innes and M. P. Y. Desmulliez, Detection of Cryptosporidium in miniaturised fluidic devices, Water Res., 2012, 46, 1641–1661 CrossRef CAS PubMed
.
- G. Luka, E. Samiei, N. Tasnim, A. Dalili, H. Najjaran and M. Hoorfar, Comprehensive review of conventional and state-of-the-art detection methods of Cryptosporidium, J. Hazard. Mater., 2022, 421, 126714 CrossRef CAS PubMed
.
- A. Efstratiou, J. Ongerth and P. Karanis, Evolution of monitoring for Giardia and Cryptosporidium in water, Water Res., 2017, 123, 96–112 CrossRef CAS PubMed
.
- M. W. Lechevallier, W. D. Norton, J. E. Siegel and M. Abbaszadegan, Evaluation of the Immunofluorescence Procedure for Detection of Giardia Cysts and Cryptosporidium Oocysts in Water, Appl. Environ. Microbiol., 1995, 61, 690–697 CrossRef CAS PubMed
.
-
USEPA, Method 1623.1: Cryptosporidium and Giardia in Water by Filtration/IMS/FA, 2012, https://www.epa.gov/sites/default/files/2021-05/documents/method_1623_1.pdf, (accessed 08 Jan 2024) Search PubMed.
- T. Kumar, M. Azlan, A. Majid, S. Onichandran, N. Jaturas, H. Andiappan, C. C. Salibay, H. A. L. Tabo, N. Tabo, J. Z. Dungca, J. Tangpong, S. Phiriyasamith, B. Yuttayong, R. Polseela, B. N. Do, N. Sawangjaroen, T.-C. Tan, Y. A. L. Lim and V. Nissapatorn, Presence of Cryptosporidium parvum and Giardia lamblia in water samples from Southeast Asia: towards an integrated water detection system, Infect. Dis. Poverty, 2016, 5, 1–12 CrossRef PubMed
.
-
U.S. Environmental Protection Agency, Method 1622: Cryptosporidium in Water by Filtration/IMS/FA, 2005, https://www.epa.gov/sites/default/files/2015-07/documents/epa-1622.pdf, (accessed 08 Jan 2024) Search PubMed.
- T. Wohlsen, J. Bates, B. Gray and M. Katouli, Evaluation of Five Membrane Filtration Methods for Recovery of Cryptosporidium and Giardia Isolates from Water Samples, Appl. Environ. Microbiol., 2004, 70, 2318–2322 CrossRef CAS PubMed
.
- G. Stanfield, E. Carrington, F. Albinet, B. Compagnon, N. Dumoutier, B. Hambsch, A. Lorthioy, G. Medema, H. Pezoldt, M. R. de Roubin, A. de Lohman and T. Whitmore, An optimised and standardised test to determine the presence of the protozoa Cryptosporidium and Giardia in water, Water Sci. Technol., 2000, 41, 103–110 CrossRef CAS
.
- H. Karim, S. Sylvain, L. Laurence, H. Lucien and C. Henry-Michel, Comparison of three methods to concentrate Giardia cysts and Cryptosporidium oocysts from surface and drinking waters, Water Sci. Technol., 2010, 62, 196–201 CrossRef CAS PubMed
.
- C. Chaidez, M. Soto, P. Gortares and K. Mena, Occurrence of Cryptosporidium and Giardia in irrigation water and its impact on the fresh produce industry, Int. J. Environ. Res. Public Health, 2006, 15, 339–345 CrossRef PubMed
.
- B. M. Hsu, C. Huang, Y. F. Hsu, G. Y. Jiang and C. L. Hsu, Evaluation of two concentration methods for detecting Giardia
and Cryptosporidium in water, Water Res., 2001, 35, 419–424 CrossRef CAS PubMed
.
- M. I. Z. Sato, A. T. Galvani, J. A. Padula, A. C. Nardocci, M. D. S. Lauretto, M. T. P. Razzolini and E. M. Hachich, Assessing the infection risk of Giardia and Cryptosporidium in public drinking water delivered by surface water systems in Sao Paulo State, Brazil, Sci. Total Environ., 2013, 442, 389–396 CrossRef CAS PubMed
.
- W. Q. Betancourt, D. C. Duarte, R. C. Vásquez and P. L. Gurian, Cryptosporidium and Giardia in tropical recreational marine waters contaminated with domestic sewage: Estimation of bathing-associated disease risks, Mar. Pollut. Bull., 2014, 85, 268–273 CrossRef CAS PubMed
.
- C. C. Falk, P. Karanis, D. Schoenen and H. M. Seitz, Bench scale experiments for the evaluation of a membrane filtration method for the recovery efficiency of Giardia and Cryptosporidium from water, Water Res., 1998, 32, 565–568 CrossRef CAS
.
- S. Xiao, W. An, Z. Chen, D. Zhang, J. Yu and M. Yang, Occurrences and genotypes of Cryptosporidium oocysts in river network of southern-eastern China, Parasitol. Res., 2012, 110, 1701–1709 CrossRef PubMed
.
- R. M. McCuin, Z. Bukhari and J. L. Clancy, Recovery and viability of Cryptosporidium parvum oocysts and Giardia intestinalis cysts using the membrane dissolution procedure, Can. J. Microbiol., 2011, 46, 700–707 CrossRef
.
- J. S. Hansen and J. E. Ongerth, Effects of time and watershed characteristics on the concentration of Cryptosporidium oocysts in river water, Appl. Environ. Microbiol., 1991, 57, 2790 CrossRef CAS PubMed
.
- J. E. Ongerth, The concentration of Cryptosporidium and Giardia in water - The role and importance of recovery efficiency, Water Res., 2013, 47, 2479–2488 CrossRef CAS PubMed
.
- Y. Lee, L. L. Gomez, I. T. McAuliffe and V. C. W. Tsang, Evaluation of Cryptosporidium parvum oocyst recovery efficiencies from various filtration cartridges by electrochemiluminescence assays, Lett. Appl. Microbiol., 2004, 39, 156–162 CrossRef CAS PubMed
.
- K. J. Kim, H. H. Jung and K. Lee, Evaluation of cyst loss in standard procedural steps for detecting of Giardia lamblia and Cryptosporidium parvum in water, Biotechnol. Bioprocess Eng., 2006, 11, 368–371 CrossRef CAS
.
- M. Inoue, S. K. Rai, T. Oda, K. Kimura, M. Nakanishi, H. Hotta and S. Uga, A new filter-eluting solution that facilitates improved recovery of Cryptosporidium oocysts from water, J. Microbiol. Methods, 2003, 55, 679–686 CrossRef PubMed
.
- B. Mull and V. R. Hill, Recovery of diverse microbes in high turbidity surface water samples using dead-end ultrafiltration, J. Microbiol. Methods, 2012, 91, 429–433 CrossRef CAS PubMed
.
- C. M. Smith and V. R. Hill, Dead-end hollow-fiber ultrafiltration for recovery of diverse microbes from water, Appl. Environ. Microbiol., 2009, 75, 5284–5289 CrossRef PubMed
.
- M. N. S. Al-Sabi, J. A. Gad, U. Riber, J. A. L. Kurtzhals and H. L. Enemark, New filtration system for efficient recovery of waterborne Cryptosporidium oocysts and Giardia cysts, J. Appl. Microbiol., 2015, 119, 894–903 CrossRef CAS PubMed
.
- U. Zuckerman, R. Armon, S. Tzipori and D. Gold, Evaluation of a portable differential continuous flow centrifuge for concentration of Cryptosporidium oocysts and Giardia cysts from water, J. Appl. Microbiol., 1999, 86, 955–961 CrossRef CAS PubMed
.
- T. Oda, M. Sakagami, H. Ito, H. Yano, S. K. Rai, M. Kawabata and S. Uga, Size selective continuous flow filtration method for detection of Cryptosporidium and Giardia, Water Res., 2000, 34, 4477–4481 CrossRef CAS
.
-
USEPA, Method 1623: Cryptosporidium and Giardia in Water by Filtration/IMS/FA, 2005, https://www.epa.gov/sites/default/files/2015-07/documents/epa-1623.pdf, (accessed 2024 Jan 8) Search PubMed.
- G. P. Yakub and K. L. Stadterman-Knauer, Evaluation of Immunomagnetic Separation for Recovery of Cryptosporidium parvum and Giardia duodenalis from High-Iron Matrices, Appl. Environ. Microbiol., 2000, 66, 3628–3631 CrossRef CAS PubMed
.
- P. C. Okhuysen, C. L. Chappell, J. H. Crabb, C. R. Sterling and H. L. DuPont, Virulence of Three Distinct Cryptosporidium parvum Isolates for Healthy Adults, J. Infect. Dis., 1999, 180, 1275–1281 CrossRef CAS PubMed
.
- S. J. Hadfield, G. Robinson, K. Elwin and R. M. Chalmers, Detection and differentiation of Cryptosporidium spp. in human clinical samples by use of real-time PCR, J. Clin. Microbiol., 2011, 49, 918–924 CrossRef CAS PubMed
.
- J. B. Burnet, L. Ogorzaly, A. Tissier, C. Penny and H. M. Cauchie, Novel quantitative TaqMan real-time PCR assays for detection of Cryptosporidium at the genus level and genotyping of major human and cattle-infecting species, J. Appl. Microbiol., 2013, 114, 1211–1222 CrossRef CAS PubMed
.
- M. S. Abrahamsen, T. J. Templeton, S. Enomoto, J. E. Abrahante, G. Zhu, C. A. Lancto, M. Deng, C. Liu, G. Widmer, S. Tzipori, G. A. Buck, P. Xu, A. T. Bankier, P. H. Dear, B. A. Konfortov, H. F. Spriggs, L. Iyer, V. Anantharaman, L. Aravind and V. Kapur, Complete genome sequence of the apicomplexan, Cryptosporidium parvum, Science, 2004, 304, 441–445 CrossRef CAS PubMed
.
- U. Ryan, A. Paparini and C. Oskam, New Technologies for Detection of Enteric Parasites, Trends Parasitol., 2017, 33, 532–546 CrossRef CAS PubMed
.
- E. F. DeLong, G. S. Wickham and N. R. Pace, Phylogenetic stains: ribosomal RNA-based probes for the identification of single cells, Science, 1989, 243, 1360–1363 CrossRef CAS PubMed
.
- R. I. Amann, L. Krumholz and D. A. Stahl, Fluorescent-oligonucleotide probing of whole cells for determinative, phylogenetic, and environmental studies in microbiology, J. Bacteriol., 1990, 172, 762–770 CrossRef CAS PubMed
.
- G. Vesey, N. Ashbolt, E. J. Fricker, D. Deere, K. L. Williams, D. A. Veal and M. Dorsch, The use of a ribosomal RNA targeted oligonucleotide probe for fluorescent labelling of viable Cryptosporidium parvum oocysts, J. Appl. Microbiol., 1998, 85, 429–440 CrossRef CAS PubMed
.
- D. Deere, G. Vesey, M. Milner, K. Williams, N. Ashbolt and D. Veal, Rapid method for fluorescent in situ ribosomal RNA labelling of Cryptosporidium parvum, J. Appl. Microbiol., 1998, 85, 807–818 CrossRef CAS PubMed
.
- D. Deere, G. Vesey, N. Ashbolt, K. A. Davies, K. L. Williams and D. Veal, Evaluation of fluorochromes for flow cytometric detection of Cryptosporidium parvum oocysts labelled by fluorescent in situ hybridization, Lett. Appl. Microbiol., 1998, 27, 352–356 CrossRef CAS PubMed
.
- A. Alagappan, P. L. Bergquist and B. C. Ferrari, Development of a two-color fluorescence in situ hybridization technique for species-level identification of human-infectious Cryptosporidium spp, Appl. Environ. Microbiol., 2009, 75, 5996–5998 CrossRef CAS PubMed
.
- A. Alagappan, N. A. Tujula, M. Power, C. M. Ferguson, P. L. Bergquist and B. C. Ferrari, Development of fluorescent in situ hybridisation for Cryptosporidium detection reveals zoonotic and anthroponotic transmission of sporadic cryptosporidiosis in Sydney, J. Microbiol. Methods, 2008, 75, 535–539 CrossRef CAS PubMed
.
- E. Chung, J. E. Aldom, A. H. Chagla, M. Kostrzynska, H. Lee, G. Palmateer, J. T. Trevors, S. Unger and S. de Grandis, Detection of Cryptosporidium parvum oocysts in municipal water samples by the polymerase chain reaction, J. Microbiol. Methods, 1998, 33, 171–180 CrossRef CAS
.
- S. Hallier-Soulier and E. Guillot, An immunomagnetic separation polymerase chain reaction assay for rapid and ultra-sensitive detection of Cryptosporidium parvum in drinking water, FEMS Microbiol. Lett., 1999, 176, 285–289 CrossRef CAS PubMed
.
- S. D. Sluter, S. Tzipori and G. Widmer, Parameters affecting polymerase chain reaction detection of waterborne Cryptosporidium parvum oocysts, Appl. Microbiol. Biotechnol., 1997, 48, 325–330 CrossRef CAS PubMed
.
- P. A. Rochelle, D. M. Ferguson, T. J. Handojo, R. de Leon, M. H. Stewart and R. L. Wolfe, An assay combining cell culture with reverse transcriptase PCR to detect and determine the infectivity of waterborne Cryptosporidium parvum, Appl. Environ. Microbiol., 1997, 63, 2029–2037 CrossRef CAS PubMed
.
- G. D. di Giovanni, F. H. Hashemi, N. J. Shaw, F. A. Abrams, M. W. Lechevallier and M. Abbaszadegan, Detection of Infectious Cryptosporidium parvum Oocysts in Surface and Filter Backwash Water Samples by Immunomagnetic Separation and Integrated Cell Culture-PCR, Appl. Environ. Microbiol., 1999, 65, 3427–3432 CrossRef CAS PubMed
.
- A. M. Johnson, G. D. di Giovanni and P. A. Rochelle, Comparison of assays for sensitive and reproducible detection of cell culture-infectious Cryptosporidium parvum and Cryptosporidium hominis in drinking water, Appl. Environ. Microbiol., 2012, 78, 156–162 CrossRef CAS PubMed
.
- M. W. LeChevallier, G. D. Di Giovanni, J. L. Clancy, Z. Bukhari, S. Bukhari, J. S. Rosen, J. Sobrinho and M. M. Frey, Comparison of method 1623 and cell culture-PCR for detection of Cryptosporidium spp. in source waters, Appl. Environ. Microbiol., 2003, 69, 971–979 CrossRef CAS PubMed
.
- J. Jiang, K. A. Alderisio, A. Singh and L. Xiao, Development of procedures for direct extraction of Cryptosporidium DNA from water concentrates and for relief of PCR inhibitors, Appl. Environ. Microbiol., 2005, 71, 1135–1141 CrossRef CAS PubMed
.
- C. Schrader, A. Schielke, L. Ellerbroek and R. Johne, PCR inhibitors – occurrence, properties and removal, J. Appl. Microbiol., 2012, 113, 1014–1026 CrossRef CAS PubMed
.
- Y. Hawash, M. M. Ghonaim and A. S. Al-Hazmi, Internal Amplification Control for a Cryptosporidium Diagnostic PCR: Construction and Clinical Evaluation, Korean J. Parasitol., 2015, 53, 147–154 CrossRef CAS PubMed
.
- P. T. Monis and C. P. Saint, Development of a nested-PCR assay for the detection of cryptosporidium parvum in finished water, Water Res., 2001, 35, 1641–1648 CrossRef CAS PubMed
.
- F. M. Ulloa-Stanojlović, B. Aguiar, L. M. Jara, M. I. Z. Sato, J. A. Guerrero, E. Hachich, G. R. Matté, M. Dropa, M. H. Matté and R. S. de Araújo, Occurrence of Giardia intestinalis and Cryptosporidium sp. in wastewater samples from São Paulo State, Brazil, and Lima, Peru, Environ. Sci. Pollut. Res., 2016, 23, 22197–22205 CrossRef PubMed
.
- N. Prystajecky, P. M. Huck, H. Schreier and J. L. Isaac-Renton, Assessment of Giardia and Cryptosporidium spp. as a microbial source tracking tool for surface water: Application in a mixed-use watershed, Appl. Environ. Microbiol., 2014, 80, 2328–2336 CrossRef PubMed
.
- R. A. Guy, P. Payment, U. J. Krull and P. A. Horgen, Real-time PCR for quantification of Giardia and Cryptosporidium in environmental water samples and sewage, Appl. Environ. Microbiol., 2003, 69, 5178–5185 CrossRef CAS PubMed
.
- R. Lappan, R. Henry, S. L. Chown, S. P. Luby, E. E. Higginson, L. Bata, T. Jirapanjawat, C. Schang, J. J. Openshaw, J. O'Toole, A. Lin, A. Tela, A. Turagabeci, T. H. F. Wong, M. A. French, R. R. Brown, K. Leder, C. Greening and D. McCarthy, Monitoring of diverse enteric pathogens across environmental and host reservoirs with TaqMan array cards and standard qPCR: a methodological comparison study, Lancet Planet. Health, 2021, 5, e297–e308 CrossRef PubMed
.
- L. Guo, K. Wan, J. Zhu, C. Ye, K. Chabi and X. Yu, Detection and distribution of vbnc/viable pathogenic bacteria in full-scale drinking water treatment plants, J. Hazard. Mater., 2021, 406, 124335 CrossRef CAS PubMed
.
- A. R. Jex, H. V. Smith, P. T. Monis, B. E. Campbell and R. B. Gasser, Cryptosporidium - Biotechnological advances in the detection, diagnosis and analysis of genetic variation, Biotechnol. Adv., 2008, 26, 304–317 CrossRef CAS PubMed
.
- N. Li, N. F. Neumann, N. Ruecker, K. A. Alderisio, G. D. Sturbaum, E. N. Villegas, R. Chalmers, P. Monis, Y. Feng and L. Xiao, Development and evaluation of three real-time PCR assays for genotyping and source tracking Cryptosporidium spp. in water, Appl. Environ. Microbiol., 2015, 81, 5845–5854 CrossRef CAS PubMed
.
- R. Yang, A. Paparini, P. Monis and U. Ryan, Comparison of next-generation droplet digital PCR (ddPCR) with quantitative PCR (qPCR) for enumeration of Cryptosporidium oocysts in faecal samples, Int. J. Parasitol., 2014, 44, 1105–1113 CrossRef CAS PubMed
.
- A. Zahedi, U. Ryan, V. Rawlings, T. Greay, S. Hancock, M. Bruce and C. Jacobson, Cryptosporidium and Giardia in dam water on sheep farms – An important source of transmission?, Vet. Parasitol., 2020, 288, 109281 CrossRef CAS PubMed
.
- Y. Zhao, F. Chen, Q. Li, L. Wang and C. Fan, Isothermal Amplification of Nucleic Acids, Chem. Rev., 2015, 115, 12491–12545 CrossRef CAS PubMed
.
- N. Tomita, Y. Mori, H. Kanda and T. Notomi, Loop-mediated isothermal amplification (LAMP) of gene sequences and simple visual detection of products, Nat. Protoc., 2008, 3, 877–882 CrossRef CAS PubMed
.
- M. A. Bakheit, D. Torra, L. A. Palomino, O. M. M. Thekisoe, P. A. Mbati, J. Ongerth and P. Karanis, Sensitive and specific detection of Cryptosporidium species in PCR-negative samples by loop-mediated isothermal DNA amplification and confirmation of generated LAMP products by sequencing, Vet. Parasitol., 2008, 158, 11–22 CrossRef CAS PubMed
.
- M. R. Mahmoudi, B. Kazemi, A. Mohammadiha, A. Mirzaei and P. Karanis, Detection of Cryptosporidium and Giardia (oo) cysts by IFA, PCR and LAMP in surface water from Rasht, Iran, Trans. R. Soc. Trop. Med. Hyg., 2013, 107, 511–517 CrossRef CAS PubMed
.
- C. Gallas-Lindemann, I. Sotiriadou, J. Plutzer, M. J. Noack, M. R. Mahmoudi and P. Karanis, Giardia and Cryptosporidium spp. dissemination during wastewater treatment and comparative detection via immunofluorescence assay (IFA), nested polymerase chain reaction (nested PCR) and loop mediated isothermal amplification (LAMP), Acta Trop., 2016, 158, 43–51 CrossRef CAS PubMed
.
- Z. Koloren, I. Sotiriadou and P. Karanis, Investigations and comparative detection of Cryptosporidium species by microscopy, nested PCR and LAMP in water supplies of ordu, middle Black Sea, Turkey, Ann. Trop. Med. Parasitol., 2011, 105, 607–615 CrossRef CAS PubMed
.
- Z. Koloren, D. Kaya and C. Avsar, Detection of Cryptosporidium Species in the Sea and Tap Water Samples of Black Sea, Turkey, J. Parasitol., 2013, 99, 554–557 CrossRef CAS PubMed
.
- B. K. Hønsvall and L. J. Robertson, From research lab to standard environmental analysis tool: Will NASBA make the leap?, Water Res., 2017, 109, 389–397 CrossRef PubMed
.
- J. C. Guatelli, K. M. Whitfield, D. Y. Kwoh, K. J. Barringer, D. D. Richman and T. R. Gingeras, Isothermal, in vitro amplification of nucleic acids by a multienzyme reaction modeled after retroviral replication, Proc. Natl. Acad. Sci. U. S. A., 1990, 87, 1874–1878 CrossRef CAS PubMed
.
- S. A. Simpkins, A. B. Chan, J. Hays, B. Pöpping and N. Cook, An RNA transcription-based amplification technique (NASBA) for the detection of viable Salmonella enterica, Lett. Appl. Microbiol., 2000, 30, 75–79 CrossRef CAS PubMed
.
- B. K. Hønsvall and L. J. Robertson, Real-time nucleic acid sequence-based amplification (NASBA) assay targeting MIC1 for detection of Cryptosporidium parvum and Cryptosporidium hominis oocysts, Exp. Parasitol., 2017, 172, 61–67 CrossRef PubMed
.
- G. Widmer, E. A. Orbacz and S. Tzipori, β-Tubulin mRNA as a marker of Cryptosporidium parvum oocyst viability, Appl. Environ. Microbiol., 1999, 65, 1584–1588 CrossRef CAS PubMed
.
- E. Bailly, S. Valot, A. Vincent, Y. Duffourd, N. Grangier, M. Chevarin, D. Costa, R. Razakandrainibe, L. Favennec, L. Basmaciyan and F. Dalle, Evaluation of Next-Generation Sequencing Applied to Cryptosporidium parvum and Cryptosporidium hominis Epidemiological Study, Pathogens, 2022, 11, 938 CrossRef CAS PubMed
.
- A. Zahedi, A. W. Gofton, T. Greay, P. Monis, C. Oskam, A. Ball, A. Bath, A. Watkinson, I. Robertson and U. Ryan, Profiling the diversity of Cryptosporidium species and genotypes in wastewater treatment plants in Australia using next generation sequencing, Sci. Total Environ., 2018, 644, 635–648 CrossRef CAS PubMed
.
- K. Braima, A. Zahedi, S. Egan, J. Austen, L. Xiao, Y. Feng, B. Witham, N. Pingault, S. Perera, C. Oskam, S. Reid and U. Ryan, Molecular analysis of cryptosporidiosis cases in Western Australia in 2019 and 2020 supports the occurrence of two swimming pool associated outbreaks and reveals the emergence of a rare C. hominis IbA12G3 subtype, Infect., Genet. Evol., 2021, 92, 104859 CrossRef CAS PubMed
.
- M. G. Mphephu, M. D. Ekwanzala and M. N. B. Momba, Cryptosporidium species and subtypes in river water and riverbed sediment using next-generation sequencing, Int. J. Parasitol., 2021, 51, 339–351 CrossRef CAS PubMed
.
- A. Zahedi, T. L. Greay, A. Paparini, K. L. Linge, C. A. Joll and U. M. Ryan, Identification of eukaryotic microorganisms with 18S rRNA next-generation sequencing in wastewater treatment plants, with a more targeted NGS approach required for Cryptosporidium detection, Water Res., 2019, 158, 301–312 CrossRef CAS PubMed
.
- A. Zahedi, A. W. Gofton, F. Jian, A. Paparini, C. Oskam, A. Ball, I. Robertson and U. Ryan, Next Generation Sequencing uncovers within-host differences in the genetic diversity of Cryptosporidium gp60 subtypes, Int. J. Parasitol., 2017, 47, 601–607 CrossRef CAS PubMed
.
- S. Hansen and A. A. el Wahed, Point-of-care or point-of-need diagnostic tests: time to change outbreak investigation and pathogen detection, Trop. Med. Infect. Dis., 2020, 5, 151 CrossRef PubMed
.
- K. J. Land, D. I. Boeras, X. S. Chen, A. R. Ramsay and R. W. Peeling, REASSURED diagnostics to inform disease control strategies, strengthen health systems and improve patient outcomes, Nat. Microbiol., 2018, 4(1), 46–54 CrossRef PubMed
.
- J. Gomez-Marquez and K. Hamad-Schifferli, Local development of nanotechnology-based diagnostics, Nat. Nanotechnol., 2021, 16, 484–486 CrossRef CAS PubMed
.
- K. M. Koo, N. Soda and M. J. A. Shiddiky, Magnetic nanomaterial–based electrochemical biosensors for the detection of diverse circulating cancer biomarkers, Curr. Opin. Electrochem., 2021, 25, 100645 CrossRef CAS
.
- R. G. Mahmudunnabi, M. Umer, K. D. Seo, D. S. Park, J. H. Chung, M. J. A. Shiddiky and Y. B. Shim, Exosomal microRNAs array sensor with a bioconjugate composed of p53 protein and hydrazine for the specific lung cancer detection, Biosens. Bioelectron., 2022, 207, 114149 CrossRef CAS PubMed
.
- K. M. Koo, L. G. Carrascosa, M. J. A. Shiddiky and M. Trau, Extensions of miRNAs for Amplification-Free Electrochemical Detection on Screen-Printed Gold Electrodes, Anal. Chem., 2016, 88, 2000–2005 CrossRef CAS PubMed
.
- M. V. Riquelme, H. Zhao, V. Srinivasaraghavan, A. Pruden, P. Vikesland and M. Agah, Optimizing blocking of nonspecific bacterial attachment to impedimetric biosensors, Sens. Biosensing Res., 2016, 8, 47–54 CrossRef
.
- T. Houssin, J. Follet, A. Follet, E. Dei-Cas and V. Senez, Label-free analysis of water-polluting parasite by electrochemical impedance spectroscopy, Biosens. Bioelectron., 2010, 25, 1122–1129 CrossRef CAS PubMed
.
- O. Laczka, L. Skillman, W. G. Ditcham, B. Hamdorf, D. K. Y. Wong, P. Bergquist and A. Sunna, Application of an ELISA-type screen printed electrode-based potentiometric assay to the detection of Cryptosporidium parvum oocysts, J. Microbiol. Methods, 2013, 95, 182–185 CrossRef CAS PubMed
.
- A. Dibao-Dina, J. Follet, M. Ibrahim, A. Vlandas and V. Senez, Electrical impedance sensor for quantitative monitoring of infection processes on HCT-8 cells by the waterborne
parasite Cryptosporidium, Biosens. Bioelectron., 2015, 66, 69–76 CrossRef CAS PubMed
.
- K. Srinivasan, C. Thiruppathiraja, K. Subramanian and K. Dinakaran, Sensitive detection of C. parvum using near infrared emitting Ag2S@silica core-shell nanospheres, RSC Adv., 2014, 4, 62399–62403 RSC
.
- C. Thiruppathiraja, S. Kamatchiammal, P. Adaikkappan and M. Alagar, An advanced dual labeled gold nanoparticles probe to detect Cryptosporidium parvum using rapid immuno-dot blot assay, Biosens. Bioelectron., 2011, 26, 4624–4627 CrossRef CAS PubMed
.
- G. S. Luka, E. Nowak, Q. R. Toyata, N. Tasnim, H. Najjaran and M. Hoorfar, Portable on-chip colorimetric biosensing platform integrated with a smartphone for label/PCR-free detection of Cryptosporidium RNA, Sci. Rep., 2021, 11, 23192 CrossRef CAS PubMed
.
- S. E. Weigum, A. Castellanos-Gonzalez, A. C. White and R. Richards-Kortum, Amplification-free detection of Cryptosporidium parvum nucleic acids with the use of DNA/RNA-directed gold nanoparticle assemblies, J. Parasitol., 2013, 99, 923–926 CrossRef CAS PubMed
.
- M. M. Kaminski, O. O. Abudayyeh, J. S. Gootenberg, F. Zhang and J. J. Collins, CRISPR-based diagnostics, Nat. Biomed. Eng., 2021, 5, 643–656 CrossRef CAS PubMed
.
- O. O. Abudayyeh and J. S. Gootenberg, CRISPR diagnostics, Science, 2021, 372, 914–915 CrossRef CAS PubMed
.
- O. O. Abudayyeh, J. S. Gootenberg, P. Essletzbichler, S. Han, J. Joung, J. J. Belanto, V. Verdine, D. B. T. Cox, M. J. Kellner, A. Regev, E. S. Lander, D. F. Voytas, A. Y. Ting and F. Zhang, RNA targeting with CRISPR–Cas13, Nature, 2017, 550, 280–284 CrossRef PubMed
.
- J. S. Chen, E. Ma, L. B. Harrington, M. da Costa, X. Tian, J. M. Palefsky and J. A. Doudna, CRISPR-Cas12a target binding unleashes indiscriminate single-stranded DNase activity, Science, 2018, 360, 436–439 CrossRef CAS PubMed
.
- M. Jinek, K. Chylinski, I. Fonfara, M. Hauer, J. A. Doudna and E. Charpentier, A programmable dual-RNA-guided DNA endonuclease in adaptive bacterial immunity, Science, 2012, 337, 816–821 CrossRef CAS PubMed
.
- L. Cong, F. A. Ran, D. Cox, S. Lin, R. Barretto, N. Habib, P. D. Hsu, X. Wu, W. Jiang, L. A. Marraffini and F. Zhang, Multiplex genome engineering using CRISPR/Cas systems, Science, 2013, 339, 819–823 CrossRef CAS PubMed
.
- R. Lei, L. Li, P. Wu, X. Fei, Y. Zhang, J. Wang, D. Zhang, Q. Zhang, N. Yang and X. Wang, RPA/CRISPR/Cas12a-Based On-Site and Rapid Nucleic Acid Detection of Toxoplasma gondii in the Environment, ACS Synth. Biol., 2022, 11, 1772–1781 CrossRef CAS PubMed
.
- Y. Li, F. Deng, T. Hall, G. Vesey and E. M. Goldys, CRISPR/Cas12a-powered immunosensor suitable for ultra-sensitive whole Cryptosporidium oocyst detection from water samples using a plate reader, Water Res., 2021, 203, 117553 CrossRef CAS PubMed
.
- E. Dressaire and A. Sauret, Clogging of microfluidic systems, Soft Matter, 2016, 13, 37–48 RSC
.
- W. Huang, L. Yang, G. Yang and F. Li, Microfluidic multi-angle laser scattering system for rapid and label-free detection of waterborne parasites, Biomed. Opt. Express, 2018, 9, 1520 CrossRef CAS PubMed
.
- W. Huang, L. Yang, L. Lei and F. Li, Label-free detection and identification of waterborne parasites using a microfluidic multi-angle laser scattering system, Opt. Commun., 2017, 400, 25–29 CrossRef CAS
.
- R. Lejard-Malki, J. Follet, A. Vlandas and V. Senez, Selective electrohydrodynamic concentration of waterborne parasites on a chip, Lab Chip, 2018, 18, 3310–3322 RSC
.
- C. Lay, C. Y. Teo, L. Zhu, X. L. Peh, H. M. Ji, B. R. Chew, R. Murthy, H. H. Feng and W. T. Liu, Enhanced microfiltration devices configured with hydrodynamic trapping and a rain drop bypass filtering architecture for microbial cells detection, Lab Chip, 2008, 8, 830–833 RSC
.
- N. Allahrabbi, Y. S. M. Chia, M. S. M. Saifullah, K. M. Lim and L. Y. Lanry Yung, A hybrid dielectrophoretic system for trapping of microorganisms from water, Biomicrofluidics, 2015, 9, 034110 CrossRef PubMed
.
- H. Narayanan Unni, D. Hartono, L. Y. L. Yung, M. M.-L. Ng, H. P. Lee, B. C. Khoo and K. M. Lim, Characterization and separation of Cryptosporidium and Giardia cells using on-chip dielectrophoresis, Biomicrofluidics, 2012, 6, 012805 CrossRef PubMed
.
- V. Farmehini, A. Rohani, Y. H. Su and N. S. Swami, A wide-bandwidth power amplifier for frequency-selective insulator-based dielectrophoresis, Lab Chip, 2014, 14, 4183–4187 RSC
.
- L. Diéguez, M. Winter, S. Molan, P. Monis, B. King and B. Thierry, Disposable microfluidic micromixers for effective capture of Cryptosporidium parvum oocysts from water samples, J. Biol. Eng., 2018, 12, 1–8 CrossRef PubMed
.
- X. Gu, L. Lei, Y. Sun, X. Si, M. Wang, F. Li, G. Yang, L. Yang, G. Pan and W. Huang, Microfluidic diffraction phase microscopy for high-throughput, artifact-free quantitative phase imaging and identification of waterborne parasites, Opt. Laser Technol., 2019, 120, 105681 CrossRef CAS
.
- S. V. Angus, H. J. Kwon and J. Y. Yoon, Field-deployable and near-real-time optical microfluidic biosensors for single-oocyst-level detection of Cryptosporidium parvum from field water samples, J. Environ. Monit., 2012, 14, 3295–3304 RSC
.
- S. J. Reinholt, A. Behrent, C. Greene, A. Kalfe and A. J. Baeumner, Isolation and amplification of mRNA within a simple microfluidic lab on a chip, Anal. Chem., 2014, 86, 849–856 CrossRef CAS PubMed
.
- S. R. Nugen, P. J. Asiello, J. T. Connelly and A. J. Baeumner, PMMA biosensor for nucleic acids with integrated mixer and electrochemical detection, Biosens. Bioelectron., 2009, 24, 2428–2433 CrossRef CAS PubMed
.
- K. A. Edwards and A. J. Baeumner, Optimization of DNA-tagged dye-encapsulating liposomes for lateral-flow assays based on sandwich hybridization, Anal. Bioanal. Chem., 2006, 386, 1335–1343 CrossRef CAS PubMed
.
- G. Robinson and R. M. Chalmers, Assessment of polymorphic genetic markers for multi-locus typing of Cryptosporidium parvum and Cryptosporidium hominis, Exp. Parasitol., 2012, 132, 200–215 CrossRef CAS PubMed
.
- S. O. S. Silva, L. J. Richtzenhain, I. N. Barros, A. M. M. C. Gomes, A. V. Silva, N. D. Kozerski, J. B. de Araújo Ceranto, L. B. Keid and R. M. Soares, A new set of primers directed to 18S rRNA gene for molecular identification of Cryptosporidium spp. and their performance in the detection and differentiation of oocysts shed by synanthropic rodents, Exp. Parasitol., 2013, 135, 551–557 CrossRef CAS PubMed
.
- L. Xiao, A. Singh, J. Limor, T. K. Graczyk, S. Gradus and A. Lal, Molecular Characterization of Cryptosporidium Oocysts in Samples of Raw Surface Water and Wastewater, Appl. Environ. Microbiol., 2001, 67, 1097–1101 CrossRef CAS PubMed
.
- L. Xiao, U. M. Morgan, J. Limor, A. Escalante, M. Arrowood, W. Shulaw, R. C. A. Thompson, R. Fayer and A. A. Lal, Genetic Diversity within Cryptosporidium parvum and Related Cryptosporidium Species, Appl. Environ. Microbiol., 1999, 65, 3386–3391 CrossRef CAS PubMed
.
- D. M. Roellig and L. Xiao, Cryptosporidium
Genotyping for Epidemiology Tracking, Methods Mol. Biol., 2020, 2052, 103–116 CrossRef CAS PubMed
.
- O. Sunnotel, W. J. Snelling, L. Xiao, K. Moule, J. E. Moore, B. C. Millar, J. S. G. Dooley and C. J. Lowery, Rapid and sensitive detection of single Cryptosporidium oocysts from archived glass slides, J. Clin. Microbiol., 2006, 44, 3285–3291 CrossRef CAS PubMed
.
- R. A. B. Nichols, L. Connelly, C. B. Sullivan and H. V. Smith, Identification of Cryptosporidium Species and Genotypes in Scottish Raw and Drinking Waters during a One-Year Monitoring Period, Appl. Environ. Microbiol., 2010, 76, 5977 CrossRef CAS PubMed
.
- Y. Feng, N. Li, L. Duan and L. Xiao, Cryptosporidium Genotype and Subtype Distribution in Raw Wastewater in Shanghai, China: Evidence for Possible Unique Cryptosporidium hominis Transmission, J. Clin. Microbiol., 2009, 47, 153–157 CrossRef CAS PubMed
.
- A. Dey, U. Ghoshal, V. Agarwal and U. C. Ghoshal, Genotyping of Cryptosporidium Species and Their Clinical Manifestations in Patients with Renal Transplantation and Human Immunodeficiency Virus Infection, J. Pathog., 2016, 2016, 1–9 CrossRef PubMed
.
- S. Hong, K. Kim, S. Yoon, W. Y. Park, S. Sim and J. R. Yu, Detection of Cryptosporidium parvum in Environmental Soil and Vegetables, J. Korean Med. Sci., 2015, 29, 1367–1371 CrossRef PubMed
.
- L. Xiao, Molecular epidemiology of cryptosporidiosis: An update, Exp. Parasitol., 2010, 124, 80–89 CrossRef CAS PubMed
.
-
L. Xiao and U. M. Ryan, Molecular epidemiology, Cryptosporidium and Cryptosporidiosis, ed. R. Fayer and L. Xiao, CRC Press, 2008, pp. 119–171 Search PubMed
.
- W. B. Strong, J. Gut and R. G. Nelson, Cloning and Sequence Analysis of a Highly Polymorphic Cryptosporidium parvum Gene Encoding a 60-Kilodalton Glycoprotein and Characterization of Its 15-and 45-Kilodalton Zoite Surface Antigen Products, Infect. Immun., 2000, 68, 4117–4134 CrossRef CAS PubMed
.
- G. Robinson, G. Pérez-Cordón, C. Hamilton, F. Katzer, L. Connelly, C. L. Alexander and R. M. Chalmers, Validation of a multilocus genotyping scheme for subtyping Cryptosporidium parvum for epidemiological purposes, Food Waterborne Parasitol., 2022, 27, e00151 CrossRef PubMed
.
- R. M. Chalmers, G. Robinson, K. Elwin and R. Elson, Analysis of the Cryptosporidium spp. and gp60 subtypes linked to human outbreaks of cryptosporidiosis in England and Wales, 2009 to 2017, Parasites Vectors, 2019, 12, 95 CrossRef PubMed
.
- I. Holzhausen, M. Lendner, F. Göhring, I. Steinhöfel and A. Daugschies, Distribution of Cryptosporidium parvum gp60 subtypes in calf herds of Saxony, Germany, Parasitol. Res., 2019, 118, 1549–1558 CrossRef CAS PubMed
.
- R. Chalmers and S. Cacció, Towards a consensus on genotyping schemes for surveillance and outbreak investigations of Cryptosporidium, Berlin, June 2016, Eurosurveillance, 2016, 21, 30338 CrossRef PubMed
.
- S. Tanriverdi, A. Grinberg, R. M. Chalmers, P. R. Hunter, Z. Petrovic, D. E. Akiyoshi, E. London, L. Zhang, S. Tzipori, J. K. Tumwine and G. Widmer, Inferences about the global population structures of Cryptosporidium parvum and Cryptosporidium hominis, Appl. Environ. Microbiol., 2008, 74, 7227–7234 CrossRef CAS PubMed
.
- M. Mallon, A. MacLeod, J. Wastling, H. Smith, B. Reilly and A. Tait, Population Structures and the Role of Genetic Exchange in the Zoonotic Pathogen Cryptosporidium parvum, J. Mol. Evol., 2003, 56(4), 407–417 CrossRef CAS PubMed
.
- W. Huang, Y. Guo, C. Lysen, Y. Wang, K. Tang, M. H. Seabolt, F. Yang, E. Cebelinski, O. Gonzalez-Moreno, T. Hou, C. Chen, M. Chen, M. Wan, N. Li, M. C. Hlavsa, D. M. Roellig, Y. Feng and L. Xiao, Multiple introductions and recombination events underlie
the emergence of a hyper-transmissible Cryptosporidium hominis subtype in the USA, Cell Host Microbe, 2023, 31, 112–123 CrossRef CAS PubMed
.
- A. R. Jex and K. M. Tyler, American variants of Cryptosporidium hominis: Over-sexed and over here?, Cell Host Microbe, 2023, 31, 5–7 CrossRef CAS PubMed
.
- A. Zahedi, A. W. Gofton, T. Greay, P. Monis, C. Oskam, A. Ball, A. Bath, A. Watkinson, I. Robertson and U. Ryan, Profiling the diversity of Cryptosporidium species and genotypes in wastewater treatment plants in Australia using next generation sequencing, Sci. Total Environ., 2018, 644, 635–648 CrossRef CAS PubMed
.
- L. Rojas-Lopez, K. Elwin, R. M. Chalmers, H. L. Enemark, J. Beser and K. Troell, Development of a gp60-subtyping method for Cryptosporidium felis, Parasites Vectors, 2020, 13, 1–8 CrossRef PubMed
.
- C. A. Nadon, E. Trees, L. K. Ng, E. M. Nielsen, A. Reimer, N. Maxwell, K. A. Kubota and P. Gerner-Smidt, Development and application of MLVA methods as a tool for inter-laboratory surveillance, Eurosurveillance, 2013, 18 DOI:10.2807/1560-7917.ES2013.18.35.20565
.
- G. Pérez-Cordón, G. Robinson, J. Nader and R. M. Chalmers, Discovery of new variable number tandem repeat loci in multiple Cryptosporidium parvum genomes for the surveillance and investigation of outbreaks of cryptosporidiosis, Exp. Parasitol., 2016, 169, 119–128 CrossRef PubMed
.
- A. Grinberg, P. J. Biggs, V. S. R. Dukkipati and T. T. George, Extensive intra-host genetic diversity uncovered in Cryptosporidium parvum using Next Generation Sequencing, Infect., Genet. Evol., 2013, 15, 18–24 CrossRef CAS PubMed
.
- J. Beser, B. M. Hallström, A. Advani, S. Andersson, G. Östlund, J. Winiecka-Krusnell, M. Lebbad, E. Alm, K. Troell and R. B. G. Arrighi, Improving the genotyping resolution of Cryptosporidium hominis subtype IbA10G2 using one step PCR-based amplicon sequencing, Infect., Genet. Evol., 2017, 55, 297–304 CrossRef CAS PubMed
.
- Y. Y. Feng, S. L. Ong, J. Y. Hu, L. F. Song, X. L. Tan and W. J. Ng, Effect of particles on the recovery of Cryptosporidium oocysts from source water samples of various turbidities, Appl. Environ. Microbiol., 2003, 69, 1898–1903 CrossRef CAS PubMed
.
-
R. Das, Cryptosporidium detection through antibody immobilization on a solid surface, 2007, Available from: DOI:10.14288/1.0062603.
- P. Pavli, S. Venkateswaran, M. Bradley and H. Bridle, Enhancing Cryptosporidium parvum recovery rates for improved water monitoring, Chemosphere, 2016, 143, 57–63 CrossRef CAS PubMed
.
- C. Schang, N. D. Crosbie, M. Nolan, R. Poon, M. Wang, A. Jex, N. John, L. Baker, P. Scales, J. Schmidt, B. R. Thorley, K. Hill, A. Zamyadi, C. W. Tseng, R. Henry, P. Kolotelo, J. Langeveld, R. Schilperoort, B. Shi, S. Einsiedel, M. Thomas, J. Black, S. Wilson and D. T. McCarthy, Passive Sampling of SARS-CoV-2 for Wastewater Surveillance, Environ. Sci. Technol., 2021, 55, 10432–10441 CrossRef CAS PubMed
.
- M. B. Jenkins, L. J. Anguish, D. D. Bowman, M. J. Walker and W. C. Ghiorse, Assessment of a dye permeability assay for determination of inactivation rates of Cryptosporidium parvum oocysts, Appl. Environ. Microbiol., 1997, 63, 3844–3850 CrossRef CAS PubMed
.
- S. Badsha, N. Mokhtar, H. Arof, Y. A. L. Lim, M. Mubin and Z. Ibrahim, Automatic Cryptosporidium and Giardia viability detection in treated water, Eurasip J. Image Video Process, 2013, 2013, 56 CrossRef
.
- R. P. Baptista, G. W. Cooper and J. C. Kissinger, Challenges
for cryptosporidium population studies, Genes, 2021, 12, 894 CrossRef CAS PubMed
.
- R. Wang, L. Zhang, C. Axén, C. Bjorkman, F. Jian, S. Amer, A. Liu, Y. Feng, G. Li, C. Lv, Z. Zhao, M. Qi, H. Dong, H. Wang, Y. Sun, C. Ning and L. Xiao, Cryptosporidium parvum IId family: clonal population and dispersal from Western Asia to other geographical regions, Sci. Rep., 2014, 4, 1–5 CAS
.
- S. Coupe, C. Sarfati, S. Hamane and F. Derouin, Detection of Cryptosporidium and identification to the species level by nested PCR and restriction fragment length polymorphism, J. Clin. Microbiol., 2005, 43, 1017–1023 CrossRef CAS PubMed
.
- I. Heo, D. Dutta, D. A. Schaefer, N. Iakobachvili, B. Artegiani, N. Sachs, K. E. Boonekamp, G. Bowden, A. P. A. Hendrickx, R. J. L. Willems, P. J. Peters, M. W. Riggs, R. O'Connor and H. Clevers, Modelling Cryptosporidium infection in human small intestinal and lung organoids, Nat. Microbiol., 2018, 3, 814–823 CrossRef CAS PubMed
.
- M. Morada, S. Lee, L. Gunther-Cummins, L. M. Weiss, G. Widmer, S. Tzipori and N. Yarlett, Continuous culture of Cryptosporidium parvum using hollow fiber technology, Int. J. Parasitol., 2016, 46, 21–29 CrossRef CAS PubMed
.
- K. Troell, B. Hallström, A. M. Divne, C. Alsmark, R. Arrighi, M. Huss, J. Beser and S. Bertilsson, Cryptosporidium as a testbed for single cell genome characterization of unicellular eukaryotes, BMC Genomics, 2016, 17, 1–12 CrossRef PubMed
.
- X. Feng, S. M. Rich, S. Tzipori and G. Widmer, Experimental evidence for genetic recombination in the opportunistic pathogen Cryptosporidium parvum, Mol. Biochem. Parasitol., 2002, 119, 55–62 CrossRef CAS PubMed
.
- T. T. Mkandawire and A. Sateriale, The Long and Short of Next Generation Sequencing for Cryptosporidium Research, Front. Cell. Infect. Microbiol., 2022, 12, 871860 CrossRef CAS PubMed
.
- A. Iqbal, J. Liu, B. Dixon, B. Zargar and S. A. Sattar, Development and application of DNA-aptamer-coupled magnetic beads and aptasensors for the detection of Cryptosporidium parvum oocysts in drinking and recreational water resources, Can. J. Microbiol., 2019, 65, 851–857 CrossRef CAS PubMed
.
- E. M. Hassan, B. R. Dixon, S. A. Sattar, A. Stalker, B. Örmeci and M. C. DeRosa, Highly sensitive magnetic-microparticle-based aptasensor for Cryptosporidium parvum oocyst detection in river water and wastewater: Effect of truncation on aptamer affinity, Talanta, 2021, 222, 121618 CrossRef CAS PubMed
.
- M. Majdinasab, A. Hayat and J. L. Marty, Aptamer-based assays and aptasensors for detection of pathogenic bacteria in food samples, TrAC, Trends Anal. Chem., 2018, 107, 60–77 CrossRef CAS
.
|
This journal is © The Royal Society of Chemistry 2024 |