Rational construction of micro-plate-like iron oxide/aluminium oxide-fabricated screen-printed carbon electrode for the electrochemical detection of the antiandrogen drug†
Received
9th November 2023
, Accepted 17th February 2024
First published on 14th March 2024
Abstract
As the rate of prostate cancer is increasing, the strategies and challenges for its treatment are attracting significant attention. In this case, nilutamide (NTM) is an antiandrogen drug employed for the treatment of prostate cancer; however, its over-usage may induce severe health issues. Thus, herein, we designed an electrochemical sensor for the detection of NTM in real samples to monitor its presence. The working surface of the sensor was based on binary metal oxide iron oxide/aluminium oxide (FeAlO) fabricated on a screen-printed carbon electrode (SPCE). The structure, surface morphology, and elemental properties of the synthesized FeAlO were characterized using XRD, Raman spectroscopy, XPS, and FESEM. The SPCE surface modified with FeAlO (FeAlO/SPCE) was analyzed using various electrochemical techniques for the optimized and sensitive detection of NTM. Significantly, the probe sensor exhibited excellent performance with a detection limit of 7.7 nM and a linear range of 0.029–634.7 μM, together with a sensitivity of 5.502 μA μM−1 cm−2. Additional studies proved that FeAlO/SPCE was recyclable using several independent electrodes. The interference study with the factors influencing NTM showed a response at a low concentration, indicating the excellent selectivity of the sensor. The stability and real sample experiment showed good results. Hence, FeAlO/SPCE will be an efficient electrode material for NTM sensing with more significant features facilitating advanced features.
Environmental significance
The modern era is facing several issues including environmental pollution as one of the major contributors. In this case, several factors are influential, such as pollutants from several industries and leftover products that are not biodegradable. Furthermore, the overuse of drugs and their discharge nowadays is one of the causes of environmental pollution. Herein, an electrochemical sensing platform was employed as a vital strategy in detecting one of these compounds, i.e., nilutamide (NTM). NTM is an antiandrogen drug used in the treatment of prostate cancer. Also, its over-consumption leads to a variety of life-threatening diseases. Hence, detecting NTM is crucial, and its electrochemical analysis was studied. The electrode material for detecting NTM was FeAlO fabricated on a disposable screen-printed carbon electrode (SPCE). Thus, the fabricated disposable electrode will be an essential tool in detecting NTM in environmental samples such as industrial river water, biological fluids such as human serum and urine samples, and pharmaceutical tablets. The as-developed sensor will be more effective in sensing many other pollutants in the environment as a key factor in reducing environmental issues.
|
1. Introduction
The widespread consumption of pharmaceutical drugs is important for avoiding various illnesses and maintaining a healthy society. However, despite the benefits and development of medication, it is associated with various drawbacks. Drugs are broadly classified according to numerous criteria and are employed to treat specific illnesses. In this case, nilutamide (NTM) is widely prescribed to patients with prostate cancer at the stage of metastasis.1 NTM is reported to be involved in the blockage of the male sex hormones.2 Particularly in the case of prostate cancer, NTM acts by suppressing testosterone, which is responsible for cell growth in the prostate.3 NTM, which is an androgen inhibitor, can be chemically referred to as (5,5-dimethyl-3-[4-nitro-3-(trifluoromethyl)phenyl]imidazolidine-2,4-dion).4 Although it is beneficial for treatment, the use of NTM for a prolonged period may lead to problems such as interstitial pneumonitis, pneumonitis, gynecomastia, hepatotoxicity, diarrhea, and elevation in serum enzymes,5 which have been reported in some cases.6 These harmful effects of NTM are attributed to its biotransformation (nitroaromatic moiety) to carcinogenic aromatic amines.7 Thus, the detection of NTM on the lab scale will be useful. In this case, although different techniques are employed to monitor the presence of NTM at higher dosages or for its analysis, the development of simple and cost-effective methods is crucial.
Several techniques can be exploited for the detection of NTM such as fluorescence, gas/liquid chromatography, spectrophotometry, chemiluminescence, atomic spectroscopy, electrochemical analysis, and inductive coupled plasma mass spectrometry.8–11 However, their high cost, difficulties in handling chemical reagents, time-consuming process, and many sophisticated steps hinder their real-time application.12 Thus, the development of simpler detection techniques that meet the requirements of modern advancement is in demand.13 In this case, the electrochemical method, which is fast and rapid for the determination of analytes, with no complicated processes, and sensitive and reproducible at different electrode surfaces will be the best strategy for sensing NTM in comparison with the above-mentioned analysis methods.14–16 The electrochemical sensing can be further enhanced using a screen-printed carbon electrode (SPCE) as the working electrode with the modification of the catalyst of choice on its carbon surface. The usage of SPCE in comparison with the widely available electrodes such as platinum, gold, and carbon electrodes is due to its high thermal capacity, resistance to chemical attack, decreased electrical resistivity, and lower rates of oxidation with environmental gases or water vapors than other electrodes, as reported in the literature.17
Metal oxides are a good choice as electrode materials for the electrochemical sensing of NTM given that they improve the analyte pre-concentration on the electrode surface, accompanied by a higher surface area. These advantages enable enhanced sensitivity and boosted electrochemical results.18 Herein, aluminium oxide (AlO) was studied, which has widespread applications due to its numerous features, attracting attention from researchers. The specific characteristics of aluminium (Al) are ideal electrical conductivity, low cost, and enhanced charge movement during the reaction, which will provide better results when modulated with other composites. Also, the idea of recycling and utilizing the Al waste can be implemented to avoid its disposal.18 Aluminium oxide (Al2O3, AlO) is used in thin film applications due to its good thin film characteristics and high thermal stability.13 Alternatively, magnetite iron oxide (FeO) with high catalytic, magnetic and electrical properties is also applicable to improve the performance of sensors.19–21 Additionally, when employed in sensing, it boosts the reaction due to the contact between the catalyst and substrate.22 The electronic structure of Fe can be tuned with a unique six-electron distribution in its 3d orbital, which further results in multiple valence states (Fe) in Fe2O3.23 Previously, an FeAlO composite was studied in electrochemical sensors, exhibiting a very low detection limit, enhanced specific surface area, and good sensitivity towards the target analyte.24,25 The benefits of FeAlO include its non-toxic nature, earth abundance, remarkable adsorption capacity and easy availability.26 Mixed metal oxides are more effective and sensitive than their single counterparts given that mixed metal oxides affect the electronic association of the system.27 Among the various preparation methods such as chemical (template assisted, solvent and other chemical synthesis methods), physical method (sputtering), microwave-assisted methods, biological, electrochemical (electrodeposition) and many other combined reactions, the hydrothermal technique (chemical method) was the most suitable in this study.28 This is due to its advantages such as product yield with bulk production rate, materials produced with a small range from micro- to quantum-level structures, simple nature, low-energy consumption, and easy to handle with no additional man power required.29
The present work on manpower the electrochemical detection of NTM was performed using FeAlO. Firstly, FeAlO was characterized to study its physico-chemical features together with its morphological analysis. The electrochemical analysis was carried out with initial optimization of the electrochemical parameters, like the concentration of FeAlO material loaded on the screen-printed carbon electrode (SPCE), the performance of the fabricated electrodes under different conditions, and real-time investigations. The hybrid combination of binary metal oxides of FeAlO was demonstrated to be cost-effective, sensitive and selective method for the detection of NTM by diverse measurements established.
2. Materials and instruments
The materials and instruments used for the study are shown in S1. Materials and instruments.†
3. Synthesis of iron oxide/aluminium oxide
The preparation of iron oxide/aluminium oxide was implemented with the addition of iron nitrate (0.1 M) and aluminium chloride (0.1 M) in 100.0 mL of distilled water. The above-mentioned homogeneous solution was stirred together with urea (0.05 M) and ammonium hydroxide (0.05 M). The reaction was constantly stirred at room temperature for 2 h, and then maintained at 120 °C for 12 h in a Teflon-coated autoclave. The resultant solution after cooling to room temperature was centrifuged several times with the solvents of ethanol and double-distilled (DD) water. The solid material after centrifugation was dried in an oven at 50 °C. The final product was collected after 12 h of drying, followed by calcination at 500 °C for 3 h. Subsequently, the prepared FeAlO was characterized and its electrochemical application was investigated. The above-mentioned procedure is schematically shown in Scheme 1.30–32
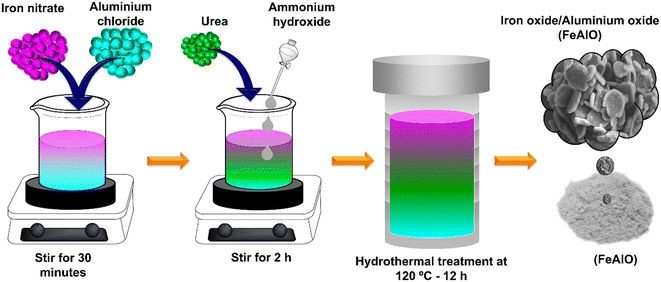 |
| Scheme 1 Schematical representation of the synthesis procedure for FeAlO. | |
3.1. Fabrication of FeAlO/SPCE
The electrochemical sensing of NTM was studied with the SPCE modified with FeAlO, and in this case, the bare SPCE required further modification before the detection of NTM. The bare SPCE was rinsed with ethanol and DD water to discard the impurities settled on the working surface. Alternatively, the FeAlO catalyst solution was prepared by dissolving the optimized quantity in 1 mL of distilled water. This solution was sonicated for 20 min and then employed for the fabrication of the bare SPCE. The above-prepared FeAlO solution was drop-coated over the cleaned SPCE, and then dried for 10 min at 50 °C. The same procedure was followed for the electrochemical optimization of all parameters in sensing NTM.
3.2. Real sample preparation
Human blood serum, human urine, industrial river water, and pharmaceutical tablets were used for the real sample analysis. Initially, the human blood serum and human urine samples were collected and studied according to the guidelines of Chang Gung Medical Foundation (IRB number: 202201485B0) approved by the Institutional Review Board of Chang-Gung Medical Foundation. Informed consent was attained for the executed study. Later, the samples were diluted with PB solution and then employed for the electrochemical study. Industrial river water was collected from Tamsui River, Taiwan further filtered, and then centrifuged at 9000 rpm for 10 min. The supernatant solution was collected and diluted with PB solution (pH 7) and directly used for NTM real sample detection. Pharmaceutical tablets purchased from the pharmacy were ground to a fine powder and then diluted with PB solution, followed by electrochemical analysis. The serum and urine samples after pretreatment were kept at −5 °C and used within 48 h for the real sample study.
4. Results and discussion
4.1. XRD analysis
Powder X-ray diffraction (XRD) was performed to examine the crystalline structure of the iron oxide/aluminium oxide compounds. The XRD pattern of iron oxide (Fe3O4), as illustrated in Fig. 1A, shows the diffraction peaks at 2θ values of 30°, 35.4°, 35.5°, 43°, 53°, and 62°, corresponding to the (112), (121), (103), (004), (204), and (224) hkl planes, respectively. The obtained results agree with JCPDS card no. 01-075-1609, with the desired peak positions and plane values as determined above. The stick pattern (green-colored representation) of the accepted JCPDS card number was inserted together with the obtained results for Fe3O4. The Fe3O4 crystal system is an orthorhombic system with a space group number of 74. Alternatively, the XRD pattern of aluminium oxide (Al2O3) was observed to have diffraction peaks at 2θ values of around 25°, 35°, 37°, 43°, 52°, 57°, 61°, 66°, and 68°, corresponding to the (012), (104), (110), (113), (024), (116), (018), (214), and (300) hkl planes, respectively, which can be ascribed to Al2O3. The successful synthesis of Al2O3 was confirmed by JCPDS card no. 01-083-2080 with a rhombohedral crystal system. The stick pattern for Al2O3 can be observed in blue color together with Fe3O4. According to the successful formation of the Fe3O4 and Al2O3 (FeAlO) material, it can be confirmed that the sample contained no impurity peaks other than FeAlO. The mean crystalline size of the as-prepared samples was evaluated by employing the Scherrer equation, as follows:
where K denotes the lattice constant with a value of 0.94, β denotes the full-width half maximum of the high-intensity plane, λ is the X-ray wavelength (1.54), and θ the diffraction angle. The mean crystalline size (D) of the synthesized samples was determined to be 23.4 nm (Fe3O4) and 24.5 nm (Al2O3).
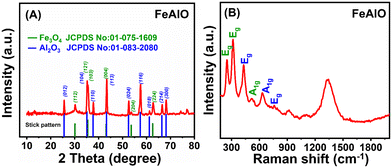 |
| Fig. 1 (A) X-ray diffraction spectra of FeAlO with stick patterns of Fe3O4 and Al2O3. (B) Raman spectrum of FeAlO. | |
4.2. Raman analysis
FeAlO was further examined using the Raman spectroscopy technique, as shown in Fig. 1B. Vibrations were observed at 250.1, 316.5, 430.2, 509, 634.7 and 1332.7 cm−1 for the FeAlO sample. The vibrations at 316.5 and 430.2 cm−1 are associated with the Al–O bond.33 Alternatively, the Raman bands at 250.1 and 509 cm−1 are attributed to the A1g mode and assigned to the Fe–O vibrational stretching.28,34 The stretching vibration band at 634.7 cm−1 is associated with the Eg mode, corresponding to the Fe–O symmetric stretching vibration. The enhanced peak intensity at 1332.7 cm−1 is ascribed to the Al–O bond.33 Thus, the Raman spectra confirmed the presence of FeAlO.
4.3. XPS analysis
The XPS technique was utilized to investigate the presence of FeAlO, as shown in Fig. 2A–D. Fig. 2A represents the overall XPS survey spectrum of FeAlO, which represents the elemental presence of Fe 2p, Al 2p, and O 1s. The spectrum of Fe 2p, as shown in Fig. 2B, was deconvoluted with peaks at various binding energies (BEs) at 710.2, 712.6, 723.9, and 727.6 eV. These binding energies are ascribed to three spin–orbital splitting of Fe 2p3/2 and Fe 2p1/2 respectively.35 The remaining peaks at 719 eV and 733 eV represent the two satellite peaks.36 The Al 2p spectrum was fitted with two peaks at 74.3 and 75.4 eV, as shown in Fig. 2C.37 The two above-mentioned peaks are attributed to the spin–orbital splitting of Al 2p3/2 and Al 2p1/2, respectively, confirming the presence of Al 2p.38Fig. 2D shows the O 1s spectrum of FeAlO deconvoluted into three peaks at BEs of 530.1, 531.7, and 533.01 eV, which are ascribed to the M–O, C–O and –OH, respectively. Thus, the XPS analysis proves the effective formation of FeAlO based on its elemental composition.
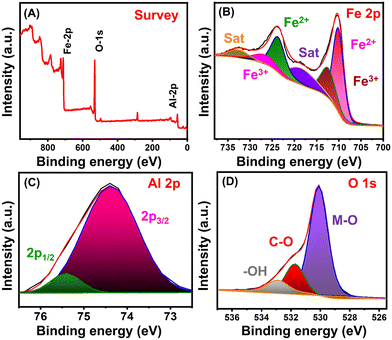 |
| Fig. 2 (A) XPS survey plot of FeAlO and XPS spectra of (B) Fe 2p, (C) Al 2p, and (D) O 1s. | |
4.4. Morphological analysis
The FESEM analysis of the FeAlO sample was performed together with EDAX and elemental mapping to determine the morphology and elements present. Fig. 3A–C shows the FESEM images of FeAlO at different magnifications. Thick plate-like structures were observed in the FESEM analysis of the FeAlO sample. They appeared to be curved and bent at the center region with the edges being thicker and smoother. Some non-uniform plates were also observed, which were attached to the uniform plate-like structures. These structures represent the unreacted particles during the synthesis. Furthermore, the elemental mapping, as shown in Fig. 3D–F, represents and confirms the presence of elements including (D) Fe, (E) Al and (F) O, and the EDAX spectrum and atomic percentage of FeAlO are depicted in Fig. 3G. Thus, the presence of pure FeAlO with no other contaminants during the sample preparation or synthesis of the sample was identified, representing the purity of the prepared sample.
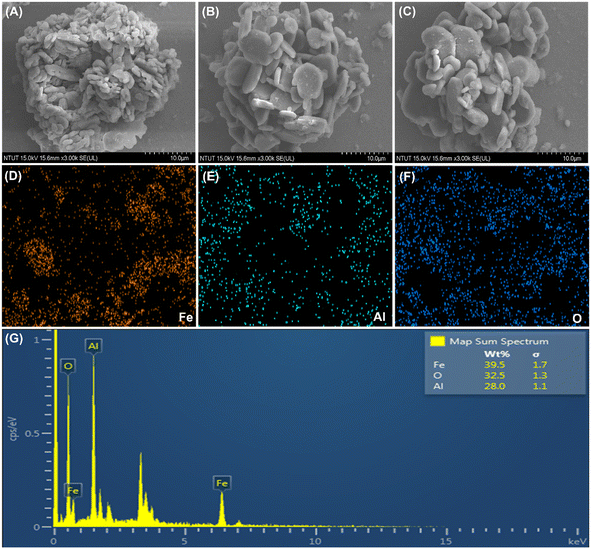 |
| Fig. 3 (A–C) FESEM images of FeAlO (D–F), elemental mapping of FeAlO, and (G) EDAX spectrum of FeAlO. | |
5. Electrochemical analysis
The cyclic voltammetry analysis of the modified and unmodified SPCE was performed in 5.0 mM [Fe(CN)6]3−/4− with 0.1 M of KCl as the electrolyte solution in the potential window of −0.6 V to 0.9 V at 0.05 V s−1. Fig. 4A shows that the CV plots of all the tested electrodes have well-defined redox curves. Initially, the surface of the bare SPCE presented a redox curve with a widened and expanded peak at Ipa of 49.15 μA, Ipc of −48.64 μA, Epa of 0.346 V, and Epc of 0.069 V. According to the comparison of the bare SPCE with FeAlO/SPCE (Ipa of 69.67 μA, Ipc of −73.85 μA, Epa of 0.302 V, and Epc of 0.114 V), the peak current of FeAlO/SPCE was greater than the bare SPCE with a smaller peak distance. The histogram depicting the above-mentioned investigation is presented in Fig. 4B. Fig. 4C displays the analysis at various scan rates for the surface area measurements. The electrolyte and other parameters remained constant, while only the scan rate was in the range of 0.02 V s−1 to 0.2 V s−1 using the modified electrodes. The electrochemical active surface area was estimated using the Randles–Sevcik formula, as follows:
Ip = (2.69 × 105)n3/2AD1/2Cν1/2 |
where, Ip is the peak current, n is the number of electrons transferred, A is the electroactive surface area (cm2), D is the diffusion coefficient (cm2 s−1), C is the concentration of the electrolyte solution (mol cm−3), and ν is the potential scan rate (V s−1). Fig. 4D represents the linear plot of scan rate vs. current for FeAlO/SPCE, where the linear equation is Ipa = 0.4279x + 41.765 for the correlation coefficient of R2 = 0.9922 and Ipc = −0.345x − 45.989 and R2 = 0.9927. Applying the previously mentioned formula, the estimated surface area for the bare SPCE and FeAlO/SPCE was 0.033, and 0.94 cm2 respectively. FeAlO/SPCE possessed a higher surface area, as indicated by the prior experiment. Consequently, the improved surface area will be significant for the enhanced performance with the combination of FeAlO.
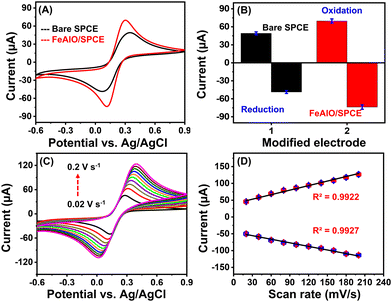 |
| Fig. 4 (A) Cyclic voltammetry graphs of bare SPCE and FeAlO/SPCE in 5.0 mM [Fe(CN)6]3−/4− and 0.1 M KCl electrolyte solution at a scan rate of 0.05 V s−1, and its (B) histogram plot, (C) CV profiles of scan rate analysis (0.02 V s−1 to 0.2 V s−1) for FeAlO/SPCE in 5.0 mM [Fe(CN)6]3−/4− and 0.1 M KCl electrolyte and (D) corresponding linear plot of scan rate vs. current. | |
5.1. Electrochemical detection of NTM
5.1.1. Optimization of the loading of FeAlO on the bare SPCE.
The electrochemical detection of NTM was performed at FeAlO/SPCE with a variation in the different parameters utilized to ensure the best result to further evaluate the fabricated electrode. According to the fabrication of the electrode, the loading of FeAlO on the bare SPCE represents a major step, which will significantly affect its performance for the detection of NTM, and hence the quantity of FeAlO in the microliter range was optimized initially. The amount of FeAlO in microliters (2.0, 4.0, 6.0, and 8.0 μL) was studied (Fig. 5A) in 0.1 M of PB solution (pH 7) with 75.0 μM of NTM at 0.05 V s−1. The peak response with a reduction peak at −0.61 V and a peak at 0.17 V was obtained for all the microliter quantities of FeAlO studied. Initially, at 2.0 μL, the response was acquired at −9.2 μA, which gradually improved when the quantity of FeAlO was higher (4.0 μL). However, it was observed that the response peak showed a lower cathodic peak response at 6.0 and 8.0 μL. The substantial and optimum loading (FeAlO) on SPCE was analyzed to be 4.0 μL. The improved active sites and the faster electron transfer between the analyte and the electrode surface led to the superior performance of the modified electrode. Also, when the loading increased, the layer over the SPCE became thicker, leading to a hindered response towards NTM. Thus, according to the analysis, the optimized quantity of FeAlO modified on the SPCE was 4 μL, which was the constant concentration of FeAlO employed for all subsequent analyses.
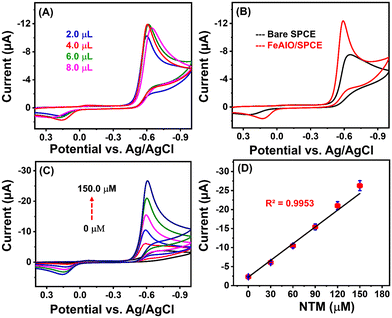 |
| Fig. 5 (A) CV curves with a variation in the quantity of FeAlO/SPCE in the presence of 75.0 μM NTM at a scan rate of 0.05 V s−1, (B) study at different modified electrodes (bare SPCE and FeAlO/SPCE) with 75.0 μM of NTM in 0.1 M PB solution at the scan rate of 0.05 V s−1, (C) different concentrations (0 μM to 150.0 μM) of NTM with 0.1 M PBS at a scan rate of 0.05 V s−1 at FeAlO/SPCE and (D) corresponding linear plot of concentration vs. current. | |
The electrochemical detection was examined using different modified SPC electrodes to analyze the changes and response to NTM. Fig. 5B shows the CV plots of the fabricated electrodes including bare SPCE and FeAlO/SPCE with 0.1 M of PB solution (pH 7.0) and an uninterrupted scan rate of 0.05 V s−1 with 75.0 μM of NTM in the constant potential window of 0.4 to −1.0 V. The bare SPCE showed a reduction peak response at around −7.51 μA at a peak potential of around −0.65 V, while that of FeAlO/SPCE was −12.35 μA. The bare SPCE response at a lower reduction current indicates that no adequate reactants contributed to its reaction with NTM reduction. In comparison with the bare electrode, the FeAlO/SPCE-modified electrode exhibited a superior cathodic peak current and the peak potential shifted to nearly −0.59 V. It is prominent that FeAlO/SPCE showed a better performance than the bare electrode for detecting NTM. According to the previous surface area analysis, the improved surface area highly contributed to the higher cathodic peak response. The absence of NTM was scrutinized with CV analysis for the bare SPCE and FeAlO/SPCE at a scan rate of 0.05 V s−1, as shown in Fig. S3A.† The CV curves showed no response, indicating the absence of NTM. Thus, NTM with FeAlO/SPCE studied in the previous analysis better represents the influence of the modified electrode.
To further understand the response of FeAlO/SPCE in the presence of NTM, its concentration was continuously increased and monitored. Fig. 5C represents the CV response curves for different concentrations of NTM from 0 to 150.0 μM. With an increase in the concentration of NTM, the reduction peak current response linearly increased with sharper reduction peaks, and no declined response or shift towards a negative or positive potential was observed due to saturation at the FeAlO/SPCE. Thus, the results demonstrate that the FeAlO electrode material has an outstanding sensing performance. Fig. 5D shows the linear plot for concentration vs. current and the linear results, corresponds to the linear equation: Ipc = 0.1615x + 1.4824 and R2 = 0.9953.
5.2. Scan rate and pH
The scan rate studies were additionally carried out during the electrochemical detection of NTM to determine the reaction potential shift and prove the appropriate parameters at different scan rates. The scan rate at the FeAlO/SPCE changed from 0.02 V s−1 to 0.2 V s−1 with 75.0 μM volume of NTM. The utilized electrolyte was 0.1 M PBS (pH 7.0) in the potential range of 0.4 V to −1.0 V. With an increase in the overall sweep rate, the current response increased as well, exhibiting no extra negative potential shifts during the experiment. Fig. 6A shows the CV images of the scan rate analysis and Fig. 6B illustrates the linear plot of scan rate vs. current with the linear regression equation of Ipc = 0.0667x + 7.1867 and R2 = 0.9913. The quick response is due to the strongly reacting ions that are not involved in the response at the electrode/electrolyte interaction. Moreover, the changes due to scan rate reflect the response current and no other additional shifts associated with potential, or the response was not noticeable. The electron transfer coefficient was calculated using the Laviron theory, as follows:
where Epc is cathodic peak potential, E° is the formal potential, R is the gas constant, T is the temperature, α is the charge transfer coefficient, ks is the standard heterogeneous rate constant, n is the number of electrons transferred in the irreversible reaction, F is the faraday constant, and ν is the scan rate. The electron transfer coefficient was determined to be 2.31 s−1. Scheme 2 depicts the reduction response process of NTM at FeAlO/SPCE.
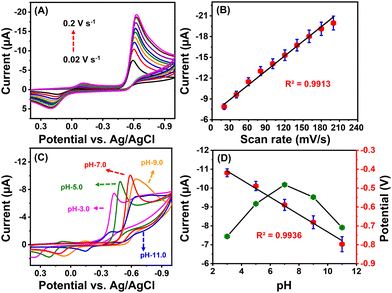 |
| Fig. 6 (A) CV curves of FeAlO/SPCE with a concentration of NTM of 75.0 μM and 0.1 M PB solution at various scan rates from 0.02 V s−1 to 0.2 V s−1, (B) linear plot of scan rate vs. current, (C) CV profiles of FeAlO/SPCE at different pH (3.0 to 11.0) with 75.0 μM NTM investigated in 0.1 M PB solution at the scan rate of 0.05 V s−1 and (D) corresponding linear plots of pH vs. current and potential. | |
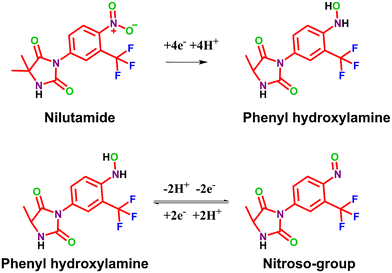 |
| Scheme 2 Electrochemical reduction mechanism of nilutamide. | |
To optimize the electrolyte (PB solution), pH ranging from acidic to alkaline medium was investigated using FeAlO/SPCE in the presence of NTM. The sweep rate was maintained at 0.05 V s−1 with NTM (75.0 μM) for varying pH values of 3.0, 5.0, 7.0, 9.0, and 11.0. The above-mentioned analysis with the CV graphs for various pH is represented in Fig. 6C. It was observed that when the pH 3.0 was studied, the reduction peak response was observed at −0.419 V and −7.44 μA as the cathodic current response. Alternatively, at pH 5.0, the potential response shifted and a greater peak current response was observed. To obtain the optimum pH response, the range of pH values was eventually changed. The response increased at pH 7, exhibiting an improved reduction peak current response (−10.18 μA), with a potential shift at −0.589 V. However, at pH 9.0, the response decreased and continued to decrease at pH 11.0. Thus, the best response was obtained at pH 7, which was utilized in the subsequent investigations for the detection of NTM. Fig. 6D shows the linear plot for pH vs. current and potential. The linear regression equation for pH vs. potential was detected to be Ipc = −0.0474x − 0.2632 and R2 = 0.9936. In response to the linear equation, −47 is almost the same as the Nernst value, which has a value of about −59 mV. Thus, according to the electrode/electrolyte interaction, the transfer of two electrons and two protons in the reaction process was identified. The FeAlO/SPCE reduction of NTM was identified with the reduction and oxidation peak, indicating the reduction of NTM to phenylhydroxylamine and phenylhydroxylamine to a nitroso group (reduction). Thus, FeAlO/SPCE was determined to be an excellent material for detecting NTM with the continuous monitoring of numerous parameters.
5.3. Differential pulse voltammetry
The DPV examinations were performed at FeAlO/SPCE for the most precise detection of NTM, and also to analyze and monitor the minor changes at different injections of NTM. The NTM concentration was continuously increased with a linear range of 0.029 μM to higher 634.7 μM under the conditions of 0.1 M PBS, pH 7.0, and potential range of 0.4 V to −1.2 V. Fig. 7A depicts the DPV profiles at FeAlO/SPCE, certainly showing a linearly higher reduction peak at about (−0.53–−0.57 V) in response to the continuous addition of NTM. The increase in NTM provided an uninterrupted increase in the current response with no changes in its potential. However, the presence of excess NTM molecules at the FeAlO/SPCE interface led to slight changes in the DPV profiles. As illustrated in Fig. 7B, the linear equation employed from the continuous measurement arising with the NTM injection is represented with two separate linear ranges representing the lower and higher concentrations of NTM. The lower concentration calibration values are Ipc = 0.3852x + 0.4013 and R2 = 0.9915, whereas Ipc = 0.0307x + 0.4791 and R2 = 0.994 at a higher concentration of NTM. Fig. 7B depicts the linear plot for the DPV analysis at FeAlO/SPCE. The detection and sensitivity limits were calculated by evaluating the as-determined DPV graphs using the equation LOD = 3SD/σ, where SD is the standard deviation obtained for the performed analysis and σ is the slope value of the calibration plot. The LOD was 7.7 nM for NTM sensing with the sensitivity determined to be 5.502 μA μM−1 cm2. Table 1 represents a comparison of the performance of the constructed sensor with previous NTM sensing results. The outcomes obtained demonstrate that the combination of FeAlO boosted the electrocatalytic efficiency at the modified SPCE surface for the efficient sensing of NTM.
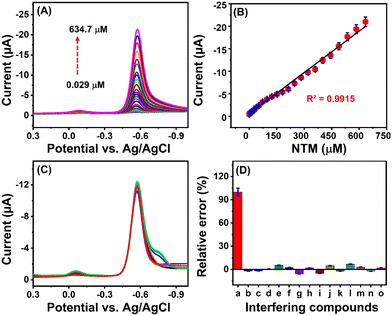 |
| Fig. 7 (A) DPV plot of FeAlO/SPCE with the addition of NTM (0.029 μM to 634.7 μM) in 0.1 M PB solution, (B) calibration plot of NTM concentration vs. current, (C) selectivity analysis studied at FeAlO/SPCE with NTM and various interferents, and (D) relative error bar of the selectivity study. | |
Table 1 Table showing several materials reported for the detection of NTM
Electrode material |
Technique |
Linear range |
Limit of detection |
Ref. |
— |
MEKC |
— |
26 μg L−1 |
39
|
DDQ/PCA |
UV-vis |
10.0–50.0 μg mL−1 |
0.0175 μg mL−1 |
40
|
ZnONPs/CPE |
SWASV |
— |
2.26 μg mL−1 |
7
|
CeV/CNF/GCE |
DPV |
0.01–540.0 μM |
2.0 nM |
41
|
BZO/SCN/GCE |
DPV |
0.09–189.61 μM |
0.006 |
42
|
SWNPs/SPCE |
DPV |
0.05–318.0 μM |
2.6 nM |
43
|
S/P/g-C3N4/GCE |
DPV |
0.019–1891.98 μM |
0.0032 μM |
3
|
FeAlO/SPCE
|
DPV
|
0.029–634.7 μM
|
0.0077 μM
|
This work
|
5.4. Interference analysis
According to the various optimization studies, all the key parameters for FeAlO/SPCE were confirmed, providing excellent outcomes for the detection of NTM. Nevertheless, the selectivity of the newly developed electrode is an essential sensing property to be studied. The selectivity study was conducted in the presence of different types of compounds categorized as interfering, anti-interfering, similar families, and closely related categories. Using the DPV technique, all the above-described compounds were examined using the same electrochemical setup with the injection of NTM (a) and other interfering compounds (b–o). The DPV graphs acquired for the selectivity analysis are depicted in Fig. 7C. Interfering compounds such as 4-nitrophenol (b), aminophenol (c), nitrobenzene (d), acetaminophen (e), flutamide (f), chlorine ions (g), sodium ions (h), glucose (i), mercury (j), mesalamine (k), ciprofloxacin (l), carbendazim (m), nifedipine (n) and hydroquinone (o) were regularly provided at a level that was 10-folds greater than NTM. Also, the influence of the metal ions was studied and shown in Fig. S3B† in 0.1 M of PBS with Cd2+, Cr2+, Pb2+, and Zn2+ metal ions. The obtained results undoubtedly indicate that FeAlO/SPCE showed strong selective action for NTM at lower levels than the other tested compounds. According to the above examination, the major impact of potential is an essential factor and the FeAlO/SPCE is highly selective to NTM even at 10-fold lower concentration than other interfering agents added. Fig. 7D shows the relative error plot for the selectivity studies. Similarly, the FeAlO/SPCE is superior exhibiting the majority of the properties suitable for the electrochemical investigation of NTM with excellent results.
5.5. Analysis of different FeAlO/SPCE sensor parameters
Studies were conducted to prove the unique properties of the electrode under different conditions such as its stability (ability to maintain a stable response for a long time), reproducibility (capacity to recreate an electrode in other electrodes) and repeatability (ability to perform repeated monitoring). At first, the stability study was performed for a duration of twenty consecutive days with a 5-days gap until the twentieth day. The study employed the CV technique at the FeAlO/SPCE modified electrode and the addition of NTM (75.0 μM) at 0.05 V s−1 as the experimental parameters. Fig. S1A† shows the stability analysis of FeAlO/SPCE for NTM sensing. The reduction response was mostly similar at every 5 days of measurement with a very small difference in the cathodic current response, which is attributed to the environmental conditions during the studies. A CV analysis was carried out to measure the reproducibility of FeAlO, which was employed using three separate electrodes. Fig. S1B† displays the results of this investigation employing the same conditions used in the stability experiments with the change in the working electrode. Only tiny differences were observable with the reduction of NTM showing similar responses in each of the three independent SPCE. A repeatability study was evaluated for almost five consecutive studies for the detection of NTM at FeAlO/SPCE. Fig. S1C† displays the CV profiles recorded for the repeatability investigation following the injection of (75.0 μM) NTM at a scan rate of 0.05 V s−1. FeAlO/SPCE repeatedly exhibited a constant reduction response for NTM sensing. The linear plots of stability, reproducibility and repeatability are depicted in Fig. S1D–F,† respectively. Additionally, Fig. S2A and B† depict the histogram diagram of repeatability (6 repeated analysis) and reproducibility (5 independent electrodes) analysis studied at FeAlO/SPCE with 75 μM of NTM to investigate the repeatability and reproducibility, respectively.
5.6. Real sample analysis
The most significant outcomes for NTM monitoring were acceptable, and therefore FeAlO/SPCE was validated using real samples. NTM was studied in real samples of human blood serum, human urine, industrial river water, and pharmaceutical tablets. All the collected real samples were preconditioned before DPV testing. The preconditioned sample preparations are shown in 3.2. Real sample preparation. Each diluted sample was taken for DPV analysis conducted at FeAlO/SPCE employing the regular addition procedure using NTM. The DPV curves for this analysis are shown in Fig. 8A–D, and the linear plots are shown in Fig. S4A–D,† together with the appropriate correlation coefficients shown as the insert, respectively. Moreover, the acquired data showed that the detection of NTM over the FeAlO/SPCE surface is beneficial, with significant rates of recovery.
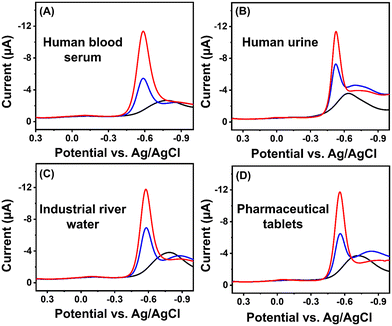 |
| Fig. 8 (A–D) DPV curves obtained at FeAlO/SPCE for real sample analysis of human blood serum, human urine, industrial river water, and pharmaceutical tablets in the presence of spiked NTM, respectively. | |
6. Conclusion
The prepared FeAlO nanohybrid for NTM sensing was effectively identified and its outcomes were excellent. The characterization of FeAlO determined its pure form with no other impurities to affect its electrochemical properties. The facile diffusion of the analyte molecules in the solution and their greater interaction with the inner surface of the FeAlO/SPC electrode resulted in a precise and stable response. The reproducibility and repeatability investigations indicated a stable performance under various conditions. The real samples including biological fluids (human blood serum and urine), environmental and pharmaceutical drug samples showed better outcomes. Thus, the as-fabricated electrode constructed with FeAlO demonstrated outstanding detection ability across multiple sensor investigations such as stability and selectivity. Thus, the developed electrode material has a wide range of potential uses due to its extensively accessible advantageous features.
Author contributions
Sivakumar Musuvadhi Babulal – conceptualization, data curation, formal analysis, investigation, methodology, writing – original draft, writing – review & editing, Nandini Nataraj – conceptualization, data curation, formal analysis, investigation, methodology, writing – original draft, writing – review & editing, Tse-Wei Chen – formal analysis, funding acquisition, project administration, resources, software, Shen Ming Chen – formal analysis, funding acquisition, project administration, resources, supervision, validation, visualization, writing – review & editing, Bih-Show Lou – formal analysis, funding acquisition, project administration, visualization, writing – review & editing, Arshad Mehmood Abbasi, Amal M. Al-Mohaimeed – formal analysis, writing – review & editing, Mohamed Soliman Elshikh, Jaysan Yu – formal analysis, resources, validation, visualization.
Conflicts of interest
The authors declare no known competing financial interests or personal relationships that could have influenced the work reported in this paper.
Acknowledgements
The authors gratefully acknowledge the financial support of the Ministry of Science and Technology, Taiwan through contract no. NSTC 112-2113-M-027-001. The financial support from the Chang Gung Memorial Hospital through contract no. CMRPD5K0021 to B. S. Lou is also acknowledged. The authors extend their appreciation to the Researchers supporting project number (RSP2023R247), King Saud University, Riyadh, Saudi Arabia.
References
- O. Payen, S. Top, A. Vessières, E. Brulé, M. A. Plamont, M. J. McGlinchey, H. Müller-Bunz and G. Jaouen, Synthesis and structure-activity relationships of the first ferrocenyl-aryl-hydantoin derivatives of the nonsteroidal antiandrogen nilutamide, J. Med. Chem., 2008, 51, 1791–1799, DOI:10.1021/jm701264d.
- R. A. Janknegt, C. C. Abbou, R. Bartoletti, L. Bernstein-Hahn, B. Bracken, J. M. Brisset, F. C. Da Silva, G. Chisholm, E. D. Crawford, F. M. J. Debruyne, G. D. Dijkman, J. Frick, L. Goedhals, H. Knonagel, P. M. Venner, D. F. Paulson and P. C. Walsh, Orchiectomy and nilutamide or placebo as treatment of metastatic prostatic cancer in a multinational double-blind randomized trial, J. Urol., 1993, 149, 77–82, DOI:10.1016/s0022-5347(17)36003-2.
- K. Y. Hwa, A. Santhan, A. Ganguly and T. S. Kanna Sharma, Two dimensional architectures of graphitic carbon nitride with the substitution of heteroatoms for bifunctional electrochemical detection of nilutamide, Chemosphere, 2023, 320, 138068, DOI:10.1016/j.chemosphere.2023.138068.
- S. Akhter, N. K. Mohd Zain, M. Shalauddin, V. K. Singh, I. I. Misnon, R. K. Sharma, S. Das, W. J. Basirun, M. R. Johan and R. Jose, Tri-metallic Co-Ni-Cu based metal organic framework nanostructures for the detection of an anticancer drug nilutamide, Sens. Actuators, A, 2021, 325, 112711, DOI:10.1016/j.sna.2021.112711.
- S. Akhter, W. J. Basirun, M. Shalauddin, M. R. Johan, S. Bagheri, O. Akbarzadeh and N. S. Anuar, Hybrid nanocomposite of functionalized multiwall carbon nanotube, nitrogen doped graphene and chitosan with electrodeposited copper for the detection of anticancer drug nilutamide in tablet and biological samples, Mater. Chem. Phys., 2020, 253, 123393, DOI:10.1016/j.matchemphys.2020.123393.
- R. N. Brogden and D. Faulds, Goserelin: A Review of its Pharmacodynamic and Pharmacokinetic Properties and Therapeutic Efficacy in Prostate Cancer, Drugs Aging, 1995, 6, 324–343, DOI:10.2165/00002512-199506040-00007.
- Y. Temerk, H. Ibrahim and N. Farhan, Square wave adsorptive stripping voltammetric determination of anticancer drug nilutamide in biological fluids using cationic surfactant cetyltrimethylammonium bromide, Anal. Methods, 2015, 7, 9137–9144, 10.1039/c5ay01811k.
- N. Nataraj, S. M. Chen and S. K. Krishnan, Strontium vanadate-supported graphitic carbon nitride nanocomposite for simultaneous voltammetric determination of acetaminophen and levofloxacin in complex biological samples, Environ. Sci.: Nano, 2022, 9, 3927–3942, 10.1039/d1en01008e.
- T. Iranmanesh, S. Jahani, M. M. Foroughi, M. S. Zandi and H. Hassani Nadiki, Synthesis of La2O3/MWCNT nanocomposite as the sensing element for electrochemical determination of theophylline, Anal. Methods, 2020, 12, 4319–4326, 10.1039/d0ay01336f.
- A. Hajializadeh, M. Ansari, M. M. Foroughi and M. Kazemipour, Ultrasonic assisted synthesis of a novel ternary nanocomposite based on carbon nanotubes/zeolitic imidazolate framework-67/polyaniline for solid-phase microextraction of organic pollutants, Microchem. J., 2020, 157, 105008, DOI:10.1016/j.microc.2020.105008.
- M. Taherizadeh, S. Jahani, M. Moradalizadeh and M. M. Foroughi, Synthesis of a dual-functional terbium doped copper oxide nanoflowers for high-efficiently electrochemical sensing of ofloxacin, pefloxacin and gatifloxacin, Talanta, 2023, 255, 124216, DOI:10.1016/j.talanta.2022.124216.
- L. Ghasemi, S. Jahani, M. Ghazizadeh and M. M. Foroughi, Simultaneous determination of amitriptyline and venlafaxine using a novel voltammetric sensor of carbon paste electrode modified with octahedral Pd2+-doped Co3O4 composite, Mater. Chem. Phys., 2023, 296, 127176, DOI:10.1016/j.matchemphys.2022.127176.
- R. Wahab and M. Alam, Aluminum oxide (Al2O3) quantum dots: A sensitive and direct electrochemical nanosensors for the detection of hydrogen peroxide, Mater. Sci. Eng., B, 2023, 290, 116308, DOI:10.1016/j.mseb.2023.116308.
- A. Santhan and K. Y. Hwa, Construction of 2D niobium carbide-embedded silver/silver
phosphate as sensitive disposable electrode material for epinephrine detection in biological real samples, Mater. Today Chem., 2023, 27, 101332, DOI:10.1016/j.mtchem.2022.101332.
- M. Salajegheh, M. Ansari, M. M. Foroghi and M. Kazemipour, Computational design as a green approach for facile preparation of molecularly imprinted polyarginine-sodium alginate-multiwalled carbon nanotubes composite film on glassy carbon electrode for theophylline sensing, J. Pharm. Biomed. Anal., 2019, 162, 215–224, DOI:10.1016/j.jpba.2018.09.032.
- R. Torkzadeh-Mahani, M. M. Foroughi, S. Jahani, M. Kazemipour and H. Hassani Nadiki, The effect of ultrasonic irradiation on the morphology of NiO/Co3O4 nanocomposite and its application to the simultaneous electrochemical determination of droxidopa and carbidopa, Ultrason. Sonochem., 2019, 56, 183–192, DOI:10.1016/j.ultsonch.2019.04.002.
- R. Wahab, F. Khan, M. Alam, J. Ahmad and A. A. Al-Khedhairy, Aluminum oxide quantum dots (Al2O3): An immediate sensing aptitude for the detection of urea, Inorg. Chem. Commun., 2023, 147, 110238, DOI:10.1016/j.inoche.2022.110238.
- S. M. Eid, K. A. M. Attia, A. El-Olemy, A. Emad, F. Abbas and N. A. Abdelshafi, An innovative nanoparticle-modified carbon paste sensor for ultrasensitive detection of lignocaine and its extremely carcinogenic metabolite residues in bovine food samples: Application of NEMI, ESA, AGREE, ComplexGAPI, and RGB12 algorithms, Food Chem., 2023, 426, 136579, DOI:10.1016/j.foodchem.2023.136579.
- R. Zokhtareh, M. Rahimnejad, G. Najafpour-Darzi and H. Karimi-Maleh, A novel sensing platform for electrochemical detection of metronidazole antibiotic based on green-synthesized magnetic Fe3O4 nanoparticles, Environ. Res., 2023, 216, 114643, DOI:10.1016/j.envres.2022.114643.
- S. Musuvadhi Babulal, L. Musuvadhi Babulal, S. M. Chen, C. Karuppiah and C. C. Yang, Fabrication of iron tin oxide/tin oxide intertwined graphitic carbon nitride hybrid composite electrocatalyst for electrochemical detection of 5-aminosalicylic acid in human urine and pharmaceutical tablet samples, J. Taiwan Inst. Chem. Eng., 2023, 145, 104837, DOI:10.1016/j.jtice.2023.104837.
- S. M. Babulal, C. Karuppiah, S. M. Chen, L. M. Babulal, S. Palanisamy, C. C. Yang and M. Chiesa, Rational synthesis of reduced graphene oxide hybrid nanocomposite with iron tungstate for selective detection of epinephrine in biological fluids, FlatChem, 2022, 36, 100445, DOI:10.1016/j.flatc.2022.100445.
- Y. Qin, K. He, B. Chen, Z. Ji and J. Wang, Ultrathin 2D/2D nanohybrid for efficient electrochemical detection of nitrite, Appl. Surf. Sci., 2023, 636, 157776, DOI:10.1016/j.apsusc.2023.157776.
- S. Wu, W. Xiong and H. Li, Insights into the Fe oxidation state of sphere-like Fe2O3 nanoparticles for simultaneous Pb2+ and Cu2+ detection, J. Alloys Compd., 2023, 934, 167863, DOI:10.1016/j.jallcom.2022.167863.
- P. Sengupta, K. Pramanik and P. Sarkar, Simultaneous detection of trace Pb(II), Cd(II) and Hg(II) by anodic stripping analyses with glassy carbon electrode modified by solid phase synthesized iron-aluminate nano particles, Sens. Actuators, B, 2021, 329, 129052, DOI:10.1016/j.snb.2020.129052.
- J. A. Joseph, S. B. Nair, K. A. John, S. Babu, S. Shaji, V. K. Shinoj and R. R. Philip, Aluminium doping in iron oxide nanoporous structures to tailor material properties for photocatalytic applications, J. Appl. Electrochem., 2020, 50, 81–92, DOI:10.1007/s10800-019-01371-6.
- A. Mahapatra, B. G. Mishra and G. Hota, Adsorptive removal of Congo red dye from wastewater by mixed iron oxide-alumina nanocomposites, Ceram. Int., 2013, 39, 5443–5451, DOI:10.1016/j.ceramint.2012.12.052.
- R. R. Tikare, R. R. Powar, S. S. Patil and D. G. Kanase, Fe3+-substituted aluminium oxide nano-flakes: Structural, morphological, optical, and gas sensing properties, Ceram. Int., 2023, 49, 5676–5686, DOI:10.1016/j.ceramint.2022.10.324.
- S. Rackauskas, A. G. Nasibulin, H. Jiang, Y. Tian, V. I. Kleshch, J. Sainio, E. D. Obraztsova, S. N. Bokova, A. N. Obraztsov and E. I. Kauppinen, A novel method for metal oxide nanowire synthesis, Nanotechnology, 2009, 20, 165603, DOI:10.1088/0957-4484/20/16/165603.
- R. Wahab and M. Alam, Highly efficient and fast electrochemical sensor based on aluminium oxide (Al2O3) nanoparticle for the detection of organosulfur compounds, Sens. Actuators, A, 2022, 347, 113967, DOI:10.1016/j.sna.2022.113967.
- Y. Han, L. Wang, D. Wang, D. Liang, S. Wang, G. Lu, Z. Di and G. Jia, Lanthanide ions-doped calcium molybdate pie-like microstructures: Synthesis, structure characterization, and luminescent properties, J. Alloys Compd., 2017, 695, 3018–3023, DOI:10.1016/j.jallcom.2016.11.355.
- M. Minakshi, D. R. G. Mitchell, C. Baur, J. Chable, A. J. Barlow, M. Fichtner, A. Banerjee, S. Chakraborty and R. Ahuja, Phase evolution in calcium molybdate nanoparticles as a function of synthesis temperature and its electrochemical effect on energy storage, Nanoscale Adv., 2019, 1, 565–580, 10.1039/c8na00156a.
- N. Nataraj, T. Chen, S. Chen, Y. Yu, M. Akilarasan, W. A. Al-onazi, M. A. Ali and M. S. Elshikh, Synthesis of hexagonal zinc oxide and rod-shaped zinc stannate as an efficient electrocatalyst for electrochemical detection of calcium channel antagonist, J. Mol. Liq., 2023, 385, 122390, DOI:10.1016/j.molliq.2023.122390.
- M. Baronskiy, A. Rastorguev, A. Zhuzhgov, A. Kostyukov, O. Krivoruchko and V. Snytnikov, Photoluminescence and Raman spectroscopy studies of low-temperature c-Al 2 O 3 phases synthesized from different precursors, Opt. Mater., 2016, 53, 87–93, DOI:10.1016/j.optmat.2016.01.029.
- A. Elgamouz, A. N. Kawde, I. A. Shehadi, S. Sayari, S. A. Abdullah Mohammed, A. Abdelrazeq, C. N. Nassab, A. A. AbdelHamid and K. Hasan, Modified Graphite Pencil Electrode Based on Graphene Oxide-Modified Fe3O4 for Ferrocene-Mediated Electrochemical Detection of Hemoglobin, ACS Omega, 2023, 8, 11880–11888, DOI:10.1021/acsomega.2c07023.
- Q. Ai, Z. Yuan, R. Huang, C. Yang, G. Jiang, J. Xiong, Z. Huang and S. Yuan, One-pot co-precipitation synthesis of Fe3O4 nanoparticles embedded in 3D carbonaceous matrix as anode for lithium ion batteries, J. Mater. Sci., 2019, 54, 4212–4224, DOI:10.1007/s10853-018-3141-3.
- C. Fu, G. Zhao, H. Zhang and S. Li, A facile route to controllable synthesis of Fe3O4/graphene composites and their application in lithium-ion batteries, Int. J. Electrochem. Sci., 2014, 9, 46–60, DOI:10.1016/s1452-3981(23)07697-6.
- A. P. Grosvenor, E. M. Bellhouse, A. Korinek, M. Bugnet and J. R. McDermid, XPS and EELS characterization of Mn 2 SiO 4, MnSiO 3 and MnAl 2 O 4, Appl. Surf. Sci., 2016, 379, 242–248, DOI:10.1016/j.apsusc.2016.03.235.
- T. Song, Q. Liu, J. Liu, W. Yang, R. Chen, X. Jing, K. Takahashi and J. Wang, Fabrication of super slippery sheet-layered and porous anodic aluminium oxide surfaces and its anticorrosion property, Appl. Surf. Sci., 2015, 355, 495–501, DOI:10.1016/j.apsusc.2015.07.140.
- J. Znaleziona, V. Maier, J. Petr, J. Chrastina and J. Ševčík, MEKC determination of nilutamide in human serum using sweeping in high salt sample matrix, Chromatographia, 2011, 74, 151–155, DOI:10.1007/s10337-011-2019-1.
- P. S. Sarat and D. Ramachandran, Two New UV-Visible Spectrophotometric Methods for the Determination of Nilutamide in Pure and their Tablets using 2, 3-Dichloro-5, 6-Dicyano-1, 4, Int. J. Pharm. Pharm. Res., 2015, 2, 161–171 CAS.
- T. Kokulnathan, R. Karthik, S. M. Chen, J. V. Kumar and S. Sakthinathan, A cerium vanadate interconnected with a carbon nanofiber heterostructure for electrochemical determination of the prostate cancer drug nilutamide, Microchim. Acta, 2019, 186, 579, DOI:10.1007/s00604-019-3665-5.
- B. Sriram, J. N. Baby, Y. F. Hsu, S. F. Wang, M. George, P. Veerakumar and K. C. Lin, Electrochemical sensor-based barium zirconate on sulphur-doped graphitic carbon nitride for the simultaneous determination of nitrofurantoin (antibacterial agent) and nilutamide (anticancer drug), J. Electroanal. Chem., 2021, 901, 115782, DOI:10.1016/j.jelechem.2021.115782.
- K. Rajendran, T. Kokulnathan, S. M. Chen, J. A. Allen, C. Viswanathan and H. A. Therese, Nitrogen doped carbon nanofibers loaded with hierarchical vanadium tetrasulfide for the voltammetric detection of the non-steroidal anti-prostate cancer drug nilutamide, Microchim. Acta, 2019, 186, 141, DOI:10.1007/s00604-019-3251-x.
|
This journal is © The Royal Society of Chemistry 2024 |