3D architectured zinc–chromium layered double hydroxide with nickel cobalt sulfide composite for the electrochemical detection of ronidazole†
Received
8th November 2023
, Accepted 18th March 2024
First published on 9th April 2024
Abstract
Currently, the elevated usage of antibiotics in the health sector has led to genetic modification, antibiotic resistance, and other adverse effects on ecosystems. Ronidazole (RZ) is a multifunctional veterinary drug that can cause severe health and environmental problems. Hence, it is essential to monitor this drug in environmental and biological samples with high accuracy. Analogously, we fabricated a zinc–chromium-layered double hydroxide with a nickel cobalt sulfide (ZC/NCS) catalyst for the electrochemical sensing of RZ. Its structural and morphological properties were deeply examined with several spectroscopic techniques. The electrochemical properties were investigated using impedance and voltammetric methods. The electroanalytical performance of ZC/NCS was studied with different voltammetric approaches. Based on the results, our prepared ZC/NCS demonstrated a low limit of detection (1.1 nM and 2.6 nM), satisfactory linear ranges of 0.01–297 μM (at low concentration), and 297–2187 μM (at high concentration), optimal sensitivity (0.374 μA μM−1 cm−2), and high performance. Furthermore, the designed sensor was applied to monitor RZ in environmental (river water), animal (chicken meat extract), and human (urine) samples, with remarkable recovery values (98.6–101.8%). Therefore, our prepared ZC/NCS composite is a promising electrode material for the real-time analysis of RZ.
Environmental significance
It has recently been determined that pharmaceutical compounds, mainly antibiotics, are a major causative agent of global pollution. These compounds and their metabolites affect the health of animals, humans, and the ecosystem in multiple ways. Hence, rapid, accurate, selective, and sensitive electrochemical sensing techniques can be employed to measure these pollutants. Advanced nanomaterials such as 2D and 3D materials offer huge surface areas and numerous active sites that lend themselves to greater efficiency. These nanomaterials facilitate more accurate and versatile detection of environmental pollution by their unique performance ability. The most precise detection of pollutants at low concentrations can be achieved with electrochemical sensors. Thereby, they can contribute to reducing the impact of pollution on the ecosystem and human health.
|
1. Introduction
Pharmaceutical products are complex organic compounds with a crucial role in human health and animal husbandries.1 There are approximately 3000 varieties of chemical substances that can be utilized as pharmaceutical compounds, with an annual manufacturing output of several hundred tons.2 Drugs are classified as antibiotics, analgesics, anti-inflammatories, antipyretics, antiseptics, and antivirals,3 and antibiotics (ABs) occupy an essential portion due to their selective inhibition behavior and satisfactory biological function.1 These compounds are widely employed as medicine for poultries, fish breeding industries, and human beings.4 In 2018, the global consumption of ABs was reported to be 40.2 billion defined daily doses per day. At present, this limit has been increased by 46% when compared with the previous year.5 The AB residues enter into the environment by (i) discharge of untreated effluent from pharmaceutical industries, (ii) human and animal usage, and (iii) the improper disposal of medicines that have reached their expiration date.6 Additionally, the digested drugs are mainly excreted from the body via urine and feces, either in unmodified or metabolite forms.2,7 These metabolites retain the potency of their parent compounds and adversely affect the ecosystem. This leads to several hazardous impacts such as mutations and the development of antibiotic resistance genes.2,7,8
Ronidazole (RZ) is a nitroimidazole-derived compound that is often employed as an anti-bacterial, anti-protozoal, and anti-coccidial agent.9 Because of its versatility, RZ is highly useful in treating numerous diseases such as bovine trichomoniasis in cattle and mice, swine dysentery in pigs, coccidiosis, histomoniasis, Campylobacter jejuni infection in poultries, and giardiasis in dog kennels.9–12 For instance, the recommended oral dosage of RZ to eliminate trichomonas in cats is 15 mg kg−1 every 12 h for 14 days.10 Hence, RZ is considered to be the most efficacious veterinary medicine. This drug can also be administered as a food additive for promoting animal growth, which results in increased economic value of livestock.13,14 RZ can also be prescribed to cure amoeba hepatic abscess, giardiasis, and Clostridium difficile in humans under the optimal minimum dosage.12,14,15 Apart from its advantages, nitroimidazole, and its derivatives are considered extremely carcinogenic, neurotoxic, genotoxic, and mutagenic.9,10,16 These metabolites can be transferred from feedstocks to humans and can lead to severe health-related problems. Consequently, the European Union, followed by the United States Food and Drug Administration, has banned these drugs from being prescribed as medicine and food additives for animals and birds.11,13,17 Because RZ is less biodegradable and easily homogenizes with water, it tends to disturb the natural balance of water systems and aquatic habitats.9
It is very important to monitor the toxicity of pollutants in the environment. For this purpose, several methodologies such as chemiluminescence, high-performance liquid chromatography, fluorometry, surface-enhanced Raman spectroscopy, microemulsion electrokinetic chromatography, and enzyme-linked immunosorbent assay were applied.18 Despite all their possible advantages, these techniques are time-consuming, complex, costly, and require skilled technicians for execution. To overcome the drawbacks of these methods, electrochemical sensors have been applied to detect target compounds with increased sensitivity and precision.19 When an electrode surface is chemically altered with a suitable electrocatalyst, the sensing performance of the respective electrode can be modified. Because of their excellent physicochemical properties, there is currently a greater amount of research being conducted on two-dimensional (2D) and three-dimensional (3D) materials as compared to 0D and 1D materials. Moreover, transition metal-based materials exhibit variable oxidation states and can generate a greater number of electrons. Therefore, they are widely employed in electrochemical sensing and energy storage applications.20,21 Ideally, these materials should show the outstanding characteristic features of a large surface area, shorter ion-diffusion pathway, satisfactory mechanical and thermal stability, high conductivity, numerous active sites, and large surface-to-volume ratio.22,23
Among the numerous 2D materials, layered double hydroxide (LDH) (with a common formula of [M2+1−xM3+x(OH)2]x+(An−)x/n·mH2O, where M2+ and M3+ denote the metal cations, and An− denote the intercalated anions (charge balancing) with n-charge) has been widely employed.24–26 LDHs possess brucite-type structures that result in a unique shape, and they are non-toxic with a tunable cation structure, anion exchange capacity at the interlayers, huge surface area, increased stability, and satisfactory flexibility.27–30 In recent times, transition metal-based LDHs have functioned as excellent electrocatalysts in sensing applications.31,32 Herein, we synthesized zinc–chromium-LDH (ZC), which is an n-type semiconductor material that acquires many redox sites with satisfactory catalytic activity and employed as an electrocatalyst.23,33 ZC is an outstanding electrode material that has been widely used in carbon dioxide reduction and degradation, and hydrogen and oxygen evolution reactions.34 Kokulnathan et al. developed a ZC sensor for monitoring furazolidone in urine and water samples.35
There has been considerable use of transition metal sulfide (TMS) because its electronegativity is lower as compared with its oxide counterparts.36,37 As an electrocatalyst, TMS offers high energy density and specific capacitance, shortened electron transport path, low electronegativity, excellent electrical conductivity, numerous active sites, stability, eco-friendliness, and also low-cost efficiency.35,38–40 TMSs exist in various morphologies such as nanoparticles, nanowires, nanotubes, and nanosheets.41 Binary metal sulfides are more efficient than single sulfides because they contribute to the synergistic performance in electrocatalysis.42 Here, we synthesized nickel cobalt sulfide (NCS) with a 3D hollow sphere structure using a two-step hydrothermal technique. Divalent and trivalent metal cations in the NCS can occupy the tetrahedral sites and the octahedral positions, respectively. This would remarkably result in greater flexibility and metallic behavior.22,43 Chen et al. reported a CB/NCS@CeO2 composite-based electrochemical sensor for the determination of nitrite in various water samples.44
In the current study, we prepared two electrocatalysts, namely, ZC and NCS, via a hydrothermal technique. The prepared functional materials were composited by a sonication method (ZC/NCS), and their morphological and physical details were analyzed with various spectroscopic techniques. The electrochemical performances of ZC, NCS, and ZC/NCS were scrutinized with voltammetric measurements. In addition, the prepared electrocatalyst was applied for the analysis of RZ in river water, chicken meat extract, and human urine samples. The important features of this work are (i) a unique 3D transition metal-based composite, (ii) no other previous study has reported this combination of materials, and (iii) the detection of RZ is enhanced by the synergistic function of the 3D hierarchical structure of ZC/NCS. (iv) ZC/NCS exhibited a very low limit of detection (LOD), remarkable selectivity, and excellent repeatability, reproducibility, and stability results, and (v) satisfactory recoveries from real samples were obtained with ZC/NCS.
2. Experimental procedure
Complete details on chemicals, reagents, and instrumentation are provided in the ESI† document.
2.1. Synthesis of ZC
Flower-like ZC was synthesized by employing the procedure outlined in previous literature.35 In brief, 3
:
1 stoichiometric ratios of Zn(NO3)2·6H2O and Cr(NO3)3·9H2O were dissolved in 60 mL of deionized (DI) water with a magnetic stirrer and named solution A. Similarly, solution B was prepared by adding 0.5 g of NH4F and 1 g of CO(NH2)2 to 10 mL of DI water. Following the complete dissolution, solution B was gradually introduced into solution A. The entire emulsion was continuously stirred for 1 h to achieve a highly homogeneous solution. Subsequently, the resulting mixture was transferred to a Teflon-coated stainless steel autoclave (100 mL), and a hydrothermal reaction was carried out at 120 °C for 6 h. During the reaction, the dissociated metal ions reacted with fluoride ions and formed metal-fluoro complexes. Upon hydrolysis, the complexed fluoride ions were replaced by the hydroxy group from water and produced metal hydroxide nanostructures. At higher temperatures (>100 °C), the urea decomposed to carbonate anions (CO32−) and intercalated into the LDH layers. This contributes to the overall stability and morphology of the final structure.45 After cooling to room temperature, the resultant precipitates were collected, washed three times with a DI water/C2H5OH mixture, and then dried overnight at 60 °C in a hot-air oven.
2.2. Synthesis of NCS
Hollow spheres of NCS were prepared by following a previously reported synthesis procedure with slight modifications.46 This typical preparation process was divided into two steps. Firstly, 1
:
2 ratios of Ni(NO3)2·6H2O and Co(NO3)2·6H2O were added to a mixture of 8 mL of glycerol and 40 mL of isopropanol, which was completely mixed with a magnetic stirrer to form a homogeneous solution (at room temperature). The resultant pink-colored emulsion was poured into the autoclave and maintained at 180 °C for 6 h. The metal ions from their salts reacted with glycerol, resulting in the stable NiCo-glyceride precursor.47 The final brown precipitate was centrifuged, washed with C2H5OH, and then dried.
Secondly, 30 mg of NiCo-glyceride precursor and 50 mg of C2H5NS were added to 40 mL of ethanol, and the solution was vigorously agitated for 1 h. Then, the entire emulsion was transferred to an autoclave and reacted at 160 °C for 6 h. In this reaction, the sulfide ions (S2−) from the decomposed C2H5NS interacted with the glyceride surface and formed NiCo-glyceride@NCS. When the reaction proceed, the complete anion exchange has been occurred and resulted in the formation of NCS. The obtained yields were washed with a solution of DI water/C2H5O and then dried. Finally, the powder was maintained in the heating zone of a tubular furnace and annealed at 350 °C for 2 h under a nitrogen atmosphere. This process significantly improved the crystallinity of the prepared NCS.47 The final black precipitate was collected, and its structural and catalytic characteristics were evaluated.
Detailed graphical representations of the ZC, NCS, and ZC/NCS preparations are illustrated in Scheme 1. In brief, the complete dissolution of Zn(NO3)2·6H2O and Cr(NO3)3·9H2O with NH4F and CH4N2O followed by a hydrothermal reaction resulted in a layered ZC structure. For NCS, the respective Ni and Co salts were dissolved in the mixture of solvents and underwent a solvothermal reaction, forming the NiCo-glyceride precursor. A certain quantity of metal glyceride precursor was further reacted with C2H5NS at an elevated temperature, and NCS spheres were produced. Annellation of the resultant NCS precipitate improved the crystallinity of the material and resulted in hollow NCS nanospheres. Finally, the as-synthesized ZC and NCS were well dispersed under sonication and formed the required ZC/NCS nanocomposite.
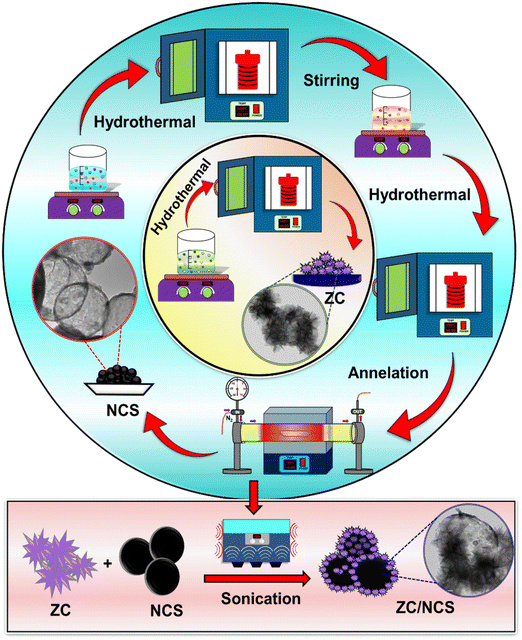 |
| Scheme 1 A detailed schematic representation of the synthesis of ZC, NCS, and ZC/NCS. | |
2.3. Electrode preparation and fabrication
Before modifying the electrodes, a bare glassy carbon electrode (GCE) was polished with an alumina–water slurry mixture, thoroughly rinsed with DI water, and then dried. The ZC/NCS composite was prepared by adding 3 mg of each material into 1 mL of DI water and sonicating the solution for 30 min to obtain a homogeneous electrocatalyst suspension. Then, 6 μL of the prepared electrocatalyst ink was cast onto the pre-treated GCE and dried in a hot-air oven. To compare the electrochemical performances of the other altered electrodes, ZC and NCS electrocatalyst slurries were individually prepared using the same procedure, and were then used to modify the GCE.
3. Results and discussion
3.1. Physical characterization
X-ray diffraction (XRD) analysis was performed to obtain crystallographic information for the as-synthesized materials and the composite (Fig. 1A). The peaks raised at the 2θ values of 11.7°, 23.6°, 34.2°, 38.6°, 46.3°, 61.0°, and 62.4° were indexed to the (003), (006), (101), (012), (015), (110), and (113) planes of hydrotalcite ZC (JCPDS Card No. 40-0215), respectively.35 Similarly, the NCS lattice planes of (111), (220), (311), (400), (511), and (440) were signaled at 16.3°, 26.7°, 31.6°, 37.7°, 50.5°, and 55.6°, respectively (JCPDS Card No. 20-0782).21 Thus, the observed NCS spectrum belongs to the Fd
m space group of the cubic crystal system. The absence of additional signals in both spectra (for ZC and NCS) implies the high purity of the prepared materials. Consequently, in the ZC/NCS spectrum, all the indexed peaks appeared without any changes in their intensities. This result strongly indicates that effective development of the ZC/NCS composite.
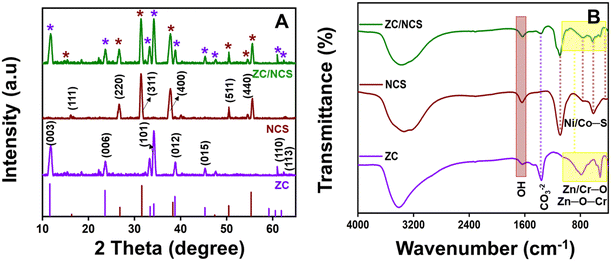 |
| Fig. 1 (A) XRD and (B) FT-IR spectra for ZC, NCS, and ZC/NCS. | |
The structural and functional information for ZC and NCS, and the ZC/NCS composite was obtained with FT-IR spectroscopy (Fig. 1B). The peaks noted at approximately 3450 cm−1 and 1650 cm−1 in all spectra were attributed to the vibrations of the –OH group from the surface-absorbed water molecules. The asymmetric stretching vibration of the interlayered CO32− ion was detected at 1357 cm−1 in ZC.23 Furthermore, the absorption signals raised between 510 to 800 cm−1 corresponded to the stretching and bending vibrations of M–O–M, O–M, and O–M–O bonds (M–Zn or Cr).35 In the case of NCS, the peaks measured at 1087 cm−1 and 763, 609, and 424 cm−1 were assigned to the asymmetric stretching and symmetric stretching vibrational modes of M′–S bonds (M′–Ni or Co), respectively.48 Finally, all the observed peaks were evenly distributed in the ZC/NCS composite, which confirmed the successful formation of the electrocatalyst, and this further validate the obtained XRD results.
The atomic states of the elements in the ZC/NCS composite were examined with HR-XPS measurement. The overall survey spectrum illustrated in Fig. S1† displays the existence of all the corresponding elements, Zn 2p, Cr 2p, and O 1s of ZC and Ni 2p, Co 2p, and S 2p of NCS in the ZC/NCS composite. In Fig. 2A, the two prominent peaks centered at the binding energies (B.E.) of 1043.6 eV and 1020.5 eV were attributed to the Zn 2p1/2 and Zn 2p3/2 of Zn2+, respectively, in the Zn 2p spectrum. The Cr 2p spectrum resulted in two main peaks at 585.9 eV and 576.4 eV that were belong to the Cr 2p1/2 and Cr 2p3/2 states of Cr3+, respectively (Fig. 2B). The two characteristic signals in the O 1s spectrum (Fig. 2C) were indexed to O2− (528.2 eV) and M–O/M–OH (529.2 eV). For Ni 2p (Fig. 2D), a pair of spin–orbit doublets was determined at B.E.s of 872.8 eV and 855.1 eV.23 They were further deconvoluted into two doublets of Ni2+ (872.8 eV and 855.1 eV) and Ni3+ (878.2 eV and 860.8 eV). This result suggests the co-existence of Ni2+ and Ni3+ states in the Ni spectrum. The notable shifts in the observed signals towards the higher B.E. were the result of the redistribution of the electronic network between the materials. Similarly, dual spin–orbit doublets emerged at (797.5 eV and 779.8 eV) and (795.4 eV and 776.9 eV) were ascribed to the Co2+ and Co3+ states of the Co 2p spectrum, respectively (Fig. 2E). In Fig. 2F of the S 2p spectrum, the obtained signals were associated with S2− (162.6 eV), M′–S (165.4 eV), and S–O (172.1 eV).49 The slight shifts in the B.E.s of the resultant spectra were attributed to composite formation. The attained XPS results further evidence the conquered XRD and FT-IR results.
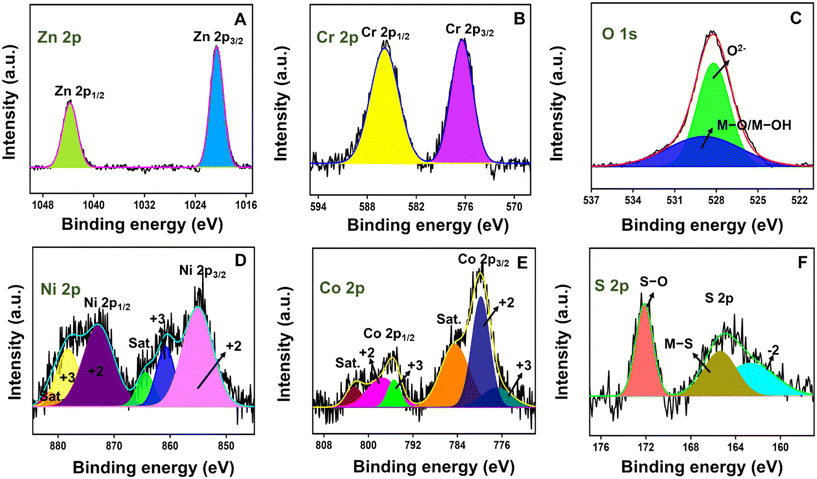 |
| Fig. 2 HR-XPS pattern of the ZC/NCS composite. (A) Zn 2p, (B) Cr 2p, (C) O 1s, (D) Ni 2p, (E) Co 2p, and (F) S 2p. | |
TEM was used to study the external morphologies of ZC, NCS, and ZC/NCS. Uniformly shaped and evenly distributed particles with smooth edges were observed for ZC in Fig. 3(A–C). It exhibits a hierarchical arrangement of ultra-thin sheets with many folds and crumbles on its surface. For NCS, hollow sphere-shaped structures with an average particle size of 300–500 nm were obtained (Fig. 3(D–F)). A highly rough and porous structure was observed on the external surface of the NCS. ZC and NCS demonstrate a 3D structure with a vast surface area and enhanced mass transport and charge transfer. The ZC/NCS composite illustrated in Fig. 3(G–I) displays the insights of the NCS nanosphere surfaces were covered with ZC nanoflowers. Their unique structural arrangement prevented restacking and aggregation of the particles and supported the exposure of maximum active sites. The lattice fringes of the ZC/NCS composite (Fig. 3J) are in greater agreement with the indexed XRD plane values of (018) for ZC and (400) for NCS. Fig. 3K shows the selected area electron diffraction (SAED) pattern of the proposed ZC/NCS composite possessing the (110) plane of ZC and the (220) plane of NCS, with well-distributed bright spots. The energy dispersive X-ray spectroscopy (EDX) (Fig. 3L) and elemental mapping (Fig. 3(M–T)) suggest the successful formation and distribution of the respective elements in the ZC/NCS composite.
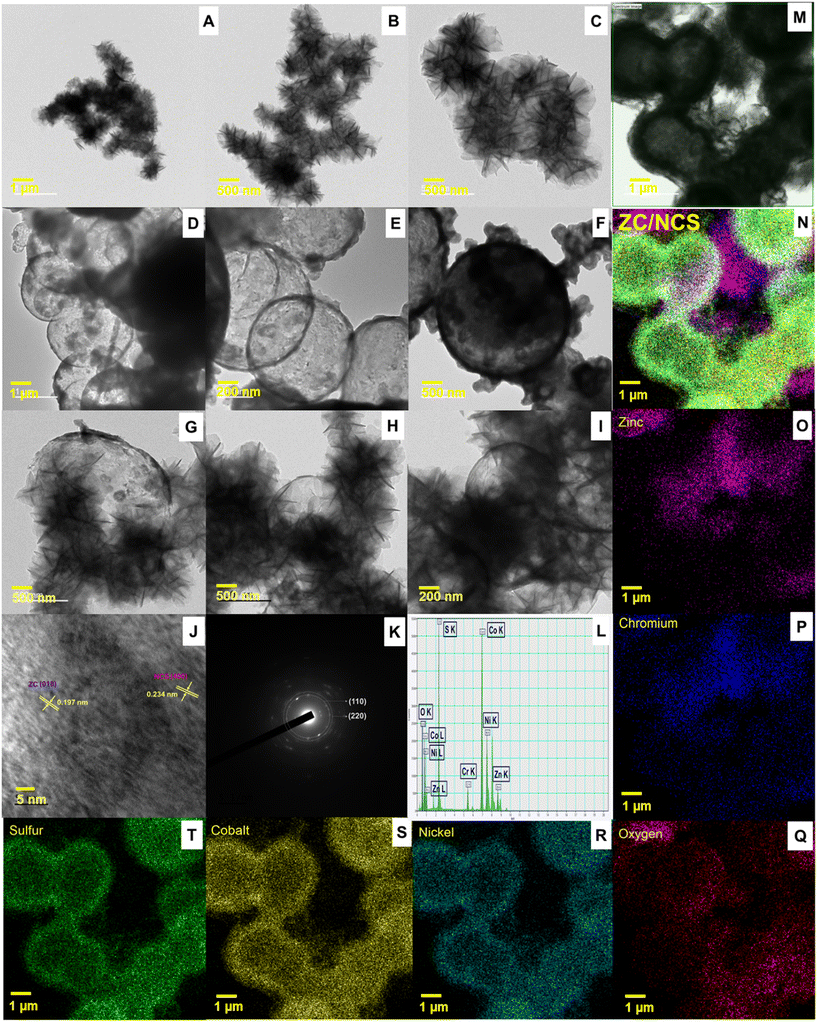 |
| Fig. 3 TEM images of (A–C) ZC, (D–F) NCS, and (G–I) ZC/NCS at different magnifications. (J) Lattice fringes, (K) SAED pattern, and (M) TEM image of ZC/NCS. Elemental mapping of (N) ZC/NCS, (O) zinc, (P) chromium, (Q) oxygen, (R) nickel, (S) cobalt, and (T) sulfur in the ZC/NCS composite. | |
3.2. Electrochemical properties of various electrodes
The interfacial properties of the unmodified and modified GCEs were established with electrochemical impedance spectroscopy (EIS) and cyclic voltammetry (CV) techniques. Both experiments were conducted in 0.1 M KCl with 5 mM [Fe(CN)6]3−/4− as the working electrolyte. In Fig. 4A, the electrochemical properties of bare GCE, ZC/GCE, NCS/GCE, and ZC/NCS/GCE were analyzed, and the measured EIS curves were fitted using Randle's equivalent circuit model (inset). The diameter of the fitted semi-circle curves corresponds to the charge transfer resistance (Rct) value. This value signifies the conductivity of the prepared materials and composite. The estimated Rct values of bare GCE, ZC/GCE, NCS/GCE, and ZC/NCS/GCE were 581 Ω, 262 Ω, 241 Ω, and 118 Ω, respectively. Our ZC/NCS-modified GCE demonstrated 5 times higher conductivity than bare GCE. This result suggests that the ZC/NCS/GCE exhibits a higher catalytic surface area, lower resistance, and faster reaction kinetics as compared to other electrodes.
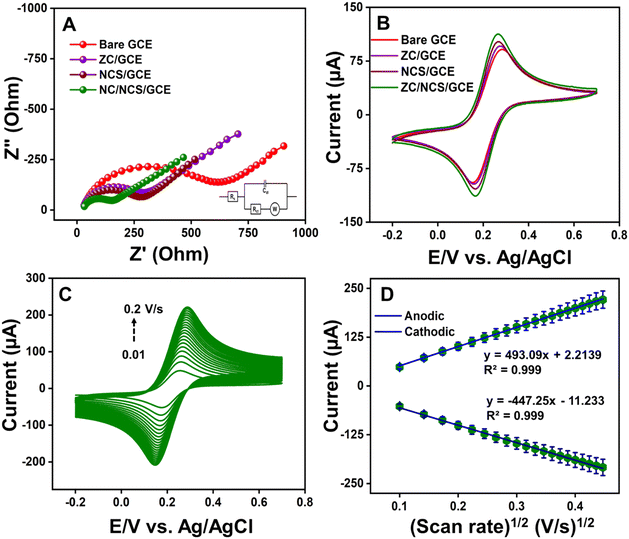 |
| Fig. 4 (A) EIS curves and (B) CV curves for bare GCE, ZC/GCE, NCS/GCE, and ZC/NCS/GCE in 1 M KCl with 5 mM [Fe(CN)6]3−/4− (inset: Randle's equivalent circuit model). (C) CV curves at various scan rates measured for the ZC/NCS/GCE and (D) its respective calibration plot between the current and the square root of the scan rate. | |
The CV curves for various electrodes were recorded and are displayed in Fig. 4B. The peak-to-peak separation (ΔEp = Epa − Epc) and the magnitude of the resultant current ratio (ipa/ipc) progressively varied while modifying the electrodes. The lower potential separation with an enhanced peak current ratio signifies the improved catalytic behavior of the modified GCEs. The obtained current intensity ratio for the ZC/NCS/GCE is approximately equal to 1. Therefore, a single electron is transferred between the reactive species in this redox reaction system.50 The electrochemical active surface area (EASA) of all the electrodes was determined by increasing the sweep rate of the reaction. Fig. S3(A–C)† and 4C display the CV curves of different electrodes at various scan rates. The respective linear calibration plots between the resultant currents and the square root of the scan rate are illustrated in Fig. S3(D–F)† and 4D. This linear relation suggests that the kinetics of the reaction follow the diffusion-controlled mechanism. Hence, the Randles–Sevcik theory was employed to determine the EASA:
| ip = (2.69 × 105)n3/2D1/2Aν1/2C | (1) |
where
ip denotes the redox peak current (μA),
A signifies the electrochemical active surface area (cm
2),
D denotes the diffusion coefficient (7.6 × 10
−6),
n symbolizes the number of electrons (
n = 1), and
ν and
C represent the scan rate (V s
−1) and bulk concentration of the molecules (0.005 M), respectively. Therefore, the obtained EASAs for bare GCE, ZC/GCE, NCS/GCE, and ZC/NCS/GCE were 0.091 cm
2, 0.099 cm
2, 0.114 cm
2, and 0.133 cm
2, respectively. Based on the results, our ZC/NCS electrocatalyst must have a vast surface area and high conductivity.
The heterogeneous electron transfer rate constant (ket) can be derived by utilizing the following equation:51
where
R denotes the gas constant (8.314 J K
−1 mol
−1),
T denotes room temperature (298 K),
Rct indicates charge transfer resistance (Ω),
A denotes EASA (cm
2), and
n,
F, and
C define the number of transferring electrons (
n = 1), Faraday constant (96
![[thin space (1/6-em)]](https://www.rsc.org/images/entities/char_2009.gif)
485 C mol
−1), and concentration of ferricyanide (0.005 C cm
−3), respectively. By employing all the determined values in
eqn (2), the
ket values were found to be 0.101 cm s
−1, 0.205 cm s
−1, 0.194 cm s
−1, and 0.339 cm s
−1 for bare GCE, ZC/GCE, NCS/GCE, and ZC/NCS/GCE, respectively. Therefore, this higher result for our final electrocatalyst-modified GCE as compared to the bare GCE implies faster reaction kinetics. This finding suggests that our ZC/NC is a promising catalyst for the electrochemical reaction.
52
3.3. Electrochemical analysis of RZ
3.3.1. Effect of loading.
The amount of electrocatalysts used to modify the electrode can potentially affect the sensing performance. Consequently, this quantity was optimized by varying the microliters of the electrocatalyst drop-cast on the GCE. This range was varied from 2 to 8 μL, and the electrochemical experiment was performed in pH 7 phosphate buffer (PB) solution in the presence of 200 μM of RZ. Fig. 5A illustrates the CV curve resulting from the experiment. The resultant peak was associated with the reduction of the nitro group into hydroxylamine in the imidazole ring structure of RZ. A bar graph diagram was drawn between the resultant current and the amount of electrocatalyst in Fig. 5B. Initially, the cathodic peak current gradually increased while the loading quantity increased from 2 to 6 μL. After 6 μL, the observed current suddenly decreased, which was the result of the blockage of available reaction sites that interacted with RZ in solution. Therefore, we determined that 6 μL was the most effective amount of ZC/NCS electrocatalyst utilized for the electrochemical reactions.
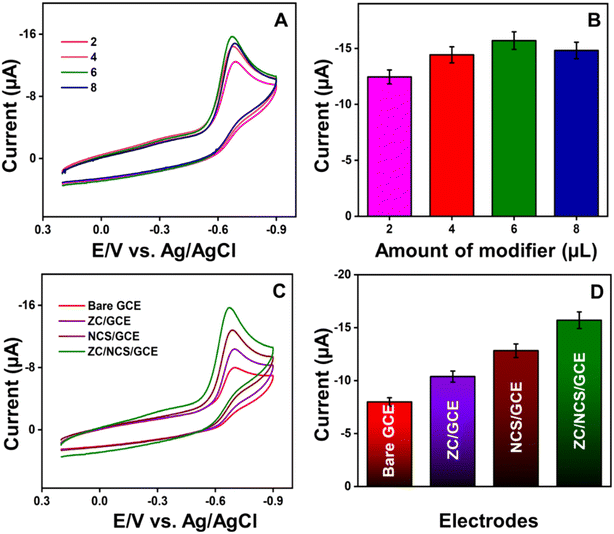 |
| Fig. 5 CV curves of (A) different amounts of loading of ZC/NCS composite on the GCE and (B) a respective bar graph diagram. (C) Different film curves, and (D) a corresponding bar diagram in the presence of 200 μM of RZ. | |
3.3.2. Effect of different electrodes.
The electrocatalytic efficiency of the prepared electrocatalyst was compared with unmodified and other modified electrodes in the presence of 200 μM of RZ in pH 7 PB solution. Fig. 5C portrays the CV signals of the various electrodes, and its relative barogram is displayed in Fig. 5D. Initially, the bare GCE demonstrated a poor electrochemical response (−7.99 μA at −0.702 V), and this was due to the lack of active sites interacting with RZ molecules. For ZC/GCE and NCS/GCE, their cathodic responses were increased to −10.38 μA and −12.82 μA, respectively. The intercalated CO32− ions (ZC) and the surface-occupied sulfide ions (NCS) participated in the anion exchange reaction and quickly interacted with RZ.47,50 The metal ion centers in the electrocatalyst actively participate in the electron transfer process and exhibit a higher electrochemical response. Finally, in ZC/NCS, the synergistic phenomenon of both materials enhanced the reaction kinetics and electrochemical reaction with a potential shift. Additionally, the lower Rct of the final composite facilitated the higher mass and charge transfer at the electrode–electrolyte interface.
3.3.3. Effect of concentration.
The influence of the concentration of RZ at the ZC/NCS/GCE was measured (Fig. 6A) in pH 7 PB solution upon the standard addition of RZ from 50 to 250 μM. For each successive addition of RZ, the reductive peak current gradually increased with a slight positive shift in its potential. This occurred because as the analyte concentration increased, there was a corresponding increase in the number of active molecules interacting with the electrocatalyst. This led to a greater number of electron transfer reactions, resulting in an enhanced current response. A linear calibration plot was outlined with the resultant reduction current and concentration, as illustrated in Fig. 6B. The regression equation can be displayed as Ipc = −0.0532 [RZ μM−1] − 4.9176 with the correlation coefficient constant (R2) of 0.979. This confirms the strong relationship between current and concentration of RZ.
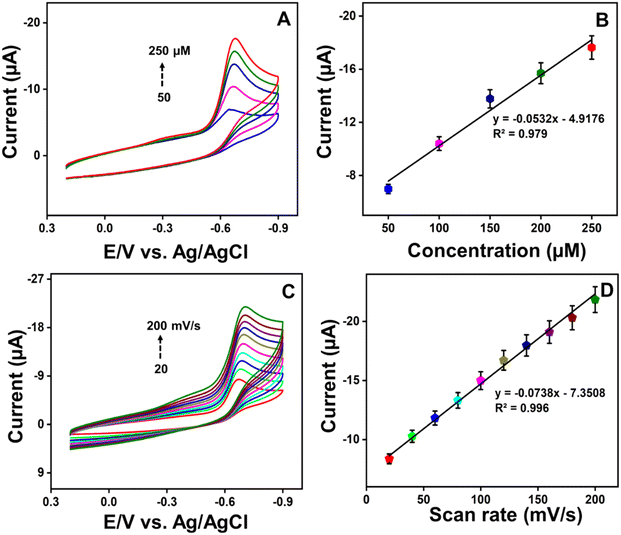 |
| Fig. 6 CV curves of (A) at different concentrations of RZ, (B) its calibration graph, (C) at different scan rates of range 20 to 200 mV s−1, and (D) its corresponding linear plot in the presence of 200 μM of RZ. | |
3.3.4. Effect of scan rate.
The impact of the scan rate on the proposed ZC/NCS/GCE for the determination of RZ was studied (Fig. 6C), and its respective calibration graph is showcased in Fig. 6D. The CV responses were recorded at different scan rates from 20 to 200 mV s−1 in the presence of 200 μM of RZ (pH 7). The cathodic signals proportionally increased while the scan rate was increased from lower to higher values along with the positive potential shift. This occurred because as the rate of the reaction increased, the rate of mass transport of reactive species near the electrode surface also increased. Therefore, a high current response can be achieved by consecutively increasing the scan rate. In addition, the change in cathodic peak potential may be attributed to the effect of the change in diffusion layer size. Using that, the regression equation was obtained as Ipc (μA) = −0.0738 (mV s−1) − 7.3508 (R2 = 0.996). From this result, the reduction of RZ on the ZC/NCS/GCE follows an adsorption-controlled mechanism.
3.3.5. Effect of pH.
The working medium also encounters the most important part of the electrochemical sensing application. Thus, the designed ZC/NCS/GCE sensor was tested by detecting RZ at various pH values, in the order of 3 to 9, and its consequent current values were measured. In Fig. 7A, it has been noted that the cathodic peak potentials of RZ were shifted towards more cathodic ones upon increasing the pH ranges. Fig. S3† shows a linear plot between the potential shift vs. pH. This aspect resulted from the protonation and deprotonation ability of RZ. Because the nitro group in the RZ molecule is weakly basic, it tends to be easily protonated. At higher concentrations of OH−, electrostatic repulsion occurs between the anion centers, which reduces the transfer of charges to the electrode.50 In Fig. 7B, the highest reduction current was noted for pH 7, and hence, it was chosen as the functioning electrolyte for all the electrochemical experiments. The general mechanism for the reduction of RZ is portrayed in Fig. 7C.
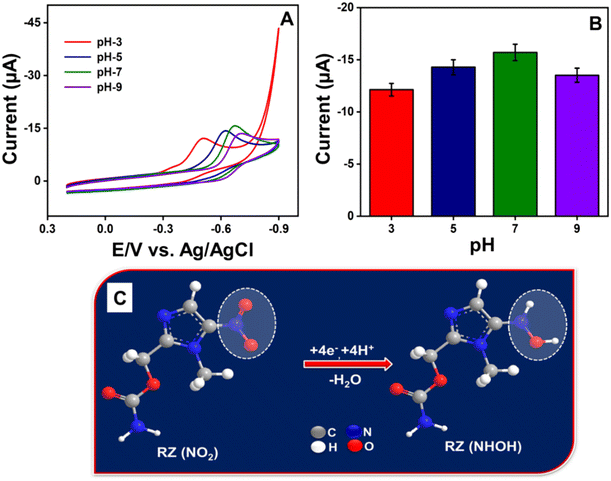 |
| Fig. 7 CV curves of (A) various pH values, (B) a related histogram measured in the presence of 200 μM of RZ, and (C) a possible electrochemical reduction mechanism for RZ. | |
3.4. Electroanalytic performance of RZ
After obtaining the optimized CV results, the analytical performance of the ZC/NCS/GCE sensor towards the detection of RZ was examined with a highly sensitive differential pulse voltammetry (DPV) technique. Under optimal parameters, the LOD and sensitivity of the ZC/NCS-based electrochemical sensor were estimated. In Fig. 8A, the DPV response of the ZC/NCS/GCE depicts a steady increase in the current response upon each addition of various concentrations of RZ over the entire range of 0.01–2187 μM. A calibration plot of current vs. concentration is illustrated in Fig. 8B with two linear ranges. The linearity covered the lower concentration range of 0.01–297 μM with R2 = 0.994 and the higher concentration range of 297–2187 μM (R2 = 0.994). The presence of two linear ranges can be attributed to the fact that the increasing concentration restricts the adsorption kinetics of RZ molecules near the electrode. Additionally, the interaction of analytes with higher concentrations was impeded by the accumulation of molecules, which consequently slows charge transport.53,54 The LOD and sensitivity were estimated by applying the following equations:where Sb denotes the standard deviation, m defines the slope of the linear plot, and A denotes the surface area of the electrode.
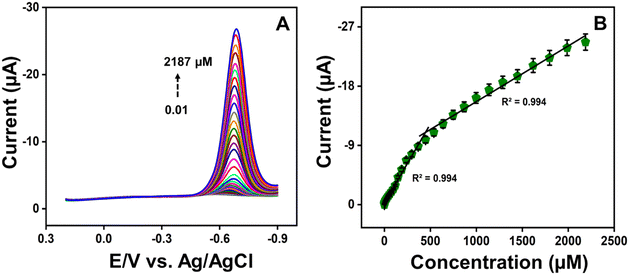 |
| Fig. 8 DPV curve of (A) different addition concentrations of RZ, and (B) its corresponding calibration plot. | |
Thus, the LOD values were derived as 1.1 nM (lower concentration range of 0.01–297 μM) and 2.6 nM (higher concentration range of 297–2189 μM), and sensitivity was calculated as 0.374 μA μM−1 cm−2. Table 1 displays the comparison table of our sensor results with other literature findings. By comparing the displayed data, it can be concluded that the sensitivity of our fabricated ZC/NCS/GCE sensor is satisfactory, with a superior trace level detection limit for RZ.
Table 1 Comparative report of ZC/NCS-based sensors for RZ detection using various techniques
Electrocatalyst |
Method |
Linear range (μM) |
LOD (nM) |
Sensitivity (μA μM−1 cm−2) |
Ref. |
MoN@GA: molybdenum nitride with graphene aerogel.
Eu3+/Tb3+@Lu-MOF: erbium and terbium in a lanthanide metal–organic-framework.
Cd-MOF: cadmium metal–organic-framework.
CdII–EuIII-based MOF: cadmium and europium-based metal–organic-framework.
CoCo2O4 NRs/h-BN: cobalt oxide with hexagonal boron nitride.
NCMO-0.5: 0.5 mmol nickel-doped cobalt molybdate.
|
MoN@GAa |
Amperometry |
0.001–918.44 |
1.3 |
78.65 |
9
|
Eu3+/Tb3+@Lu-MOFb |
Fluorescence |
— |
26.7 |
— |
14
|
Cd-MOFc |
Fluorescence |
— |
160 000 |
— |
55
|
CdII–EuIII-based MOFd |
Luminescence |
— |
1859 |
— |
56
|
CoCo2O4 NRs/h-BNe |
DPV |
0.01–10.41 |
3 |
5.845 |
54
|
12.81–1345.41 |
NCMO-0.5f |
DPV |
0.03–8.82 |
15 |
1.57 |
57
|
12.83–1221.83 |
ZC/NCS
|
DPV
|
0.01–297
|
1.1
|
0.374
|
This work
|
297–2187
|
2.6
|
3.4.1. Anti-interference study.
The practical utility of the proposed ZC/NCS/GCE sensor was tested with selectivity and anti-interference studies (Fig. S4A†). Many potential co-interfering compounds such as metal ions, biomolecules, and nitro derivatives were added (at a 10-fold higher concentration) into the electrochemical system containing 30 μM of RZ. Analytes namely, (a) calcium, (b) magnesium, (c) nitrate, (d) sodium, (e) zinc, (f) catechin, (g) hydrogen peroxide, (h) glucose, (i) hydrazine, (j) hydroquinone, (k) urea, (l) vitamin C, (m) acetic acid, (n) ascorbic acid, (o) fumaric acid, (p) gallic acid, (q) oxalic acid, (r) succinic acid, (s) uric acid, (t) 4-nitroaniline, (u) furazolidone, (v) 4-nitrobenzoic acid, (w) flutamide, and (x) 4-nitrophenol, and their corresponding DPV signals were measured. Bar graph diagrams were plotted with the obtained currents vs. interfering compounds, and the relative error graphs vs. interfering compounds are depicted in Fig. S4B and C,† respectively. These results indicated that there was a very negligible response to the added interferons and satisfactory selectivity for RZ. This proves that our ZC/NCS-based sensor could be effectively applied to analyze RZ.
3.4.2. Repeatability, reproducibility, and storage stability.
The performance reliability of the ZC/NCS/GCE sensor was demonstrated with repeatability and reproducibility tests. For repeatability, a single GCE was modified thrice with 6 μL of ZC/NCS electrocatalyst, and experiments were performed. The resultant DPV signals were noted in the presence of 200 μM of RZ, as shown in Fig. S5A.† All the reduction peaks were raised at the same potential in all three repetitions, with a relative standard deviation (RSD) of 0.51%. The reproducibility test was performed with the three different 6 μL ZC/NCS-modified GCEs in the presence of 200 μM of RZ (Fig. S5B†). By using the obtained current values, the RSD was calculated as 0.56%. The stable capability of the ZC/NCS/GCE sensor was estimated with storage stability. A 6 μL ZC/NCS-modified GCE was stored for two weeks, and experiments were performed by injecting 200 μM of RZ each week. It can be concluded from Fig. S5C† that the storage stability of our designed sensor is excellent.
3.4.3. Real-sample analysis.
The practical utility of the ZC/NCS/GCE in real-world trials was tested with river water, chicken meat extract, and human urine samples (Fig. S6(A–C)†). A urine sample was collected from a healthy adult volunteer, the chicken was purchased from a local market in Taipei, and a water sample was collected from the Xindian River, Taiwan. The human urine sample was well diluted, centrifuged (1 h), and filtered with Whatman filter paper. The chicken meat extract was prepared by grinding a small piece of chicken meat with PB solution, centrifuging it, and filtering the sample. Similarly, the water sample was treated by centrifuging the water for 1 h, followed by filtration.
Afterwards, all the pre-treated samples were diluted with 0.1 M PB (pH 7) solution at a ratio of 3
:
7, and an electrochemical experiment was performed. After the current value for blank solutions was measured, a known concentration of RZ was added to the electrolyte mixture, and its corresponding reduction peak currents were noted. The recovery percentage for the as-fabricated ZC/NCS/GCE sensor was then calculated, and the results are displayed in Table S1.† With obtained results of 98.6–101.8%, it can be concluded that our prepared sensor can be utilized to detect RZ in real-time samples.
4. Conclusion
We fabricated a hierarchically structured ZC/NCS electrocatalyst for the detection of RZ. The physicochemical properties were investigated with the spectroscopic techniques of XRD, FT-IR, HR-XPS, as well as TEM. The electrochemical properties were tested by modifying the GCE with ZC/NCS, and the results were compared with bare GCE, ZC/GCE, and NCS/GCE using EIS, and CV techniques. The electroanalytical efficiency of our sensor was estimated with DPV measurement. Our prepared sensor exhibited excellent analytical performance with low LOD (1.1 nM and 2.6 nM), excellent linear range (0.001–2187 μM), satisfactory sensitivity (0.374 μA μM−1 cm−2), and outstanding anti-interference ability. It also exhibited strong repeatability (0.51%), reproducibility (0.56%), and stability. Our proposed sensor was applied for the real-time monitoring of RZ in river water, chicken meat extract, and human urine samples, and we obtained outstanding recovery values (98.6–101.8%). Hence, our ZC/NCS/GCE is an excellent potential electrochemical sensor for the determination of RZ.
Ethical statement
This research study was conducted in strict adherence to the ethical guidelines and principles outlined in the Declaration of Helsinki, ensuring the protection and well-being of human research participants. The objective of this research is to investigate the clinical application of 3D architectured zinc–chromium layered double hydroxide with nickel cobalt sulfide composite for the electrochemical detection of ronidazole for analysis of human urine samples. This study seeks to contribute to the scientific understanding of drug detection methods, with potential applications in healthcare and forensic analysis. Participation in this research study was entirely voluntary. Participants from Chang Gung Memorial Hospital, Taiwan were fully informed about the study, including its purpose, procedures, potential risks, and benefits before commencing this research. All personal information is handled with the strictest confidentiality to protect the privacy of participants. This research project has undergone ethical review by the Chang Gung Biosafety Committee (contract number: 202101616B0), an independent and qualified committee, to ensure compliance with ethical standards and regulations. This research is designed to significantly contribute to the scientific understanding of electrochemical detection methods for ronidazole. This research is designed to significantly contribute to scientific knowledge and may have potential social benefits. The research strictly adheres to all relevant laws and regulations governing the conduct of research with human participants.
Conflicts of interest
There are no conflicts to declare.
Acknowledgements
The authors gratefully acknowledge the financial support of the National Science and Technology Council through contract no. NSTC 112-2221-E-182-022 and NSTC 112-2113-M-027-001. The financial support from the Chang Gung Memorial Hospital through contract no. CMRPD5M0021 to B. S. Lou is also acknowledged. The authors extend their appreciation to the researchers supporting project number RSP2024R469, King Saud University, Riyadh, Saudi Arabia.
References
- H. Zeng, J. Li, W. Zhao, J. Xu, H. Xu, D. Li and J. Zhang, The Current Status and Prevention of Antibiotic Pollution in Groundwater in China, Int. J. Environ. Res. Public Health, 2022, 19, 11256 CrossRef CAS.
- M. Ortúzar, M. Esterhuizen, D. Olicón-Hernández, J. González-López and E. Aranda Ballesteros, Pharmaceutical pollution in aquatic environments: a concise review of environmental impacts and bioremediation systems, Front. Microbiol., 2022, 13, 1–25 CrossRef PubMed.
- S. Bhatt and S. Chatterjee, Fluoroquinolone antibiotics: Occurrence, mode of action, resistance, environmental detection, and remediation–A comprehensive review, Environ. Pollut., 2022, 120440 CrossRef CAS PubMed.
- A. G. Ayankojo, J. Reut, V. B. C. Nguyen, R. Boroznjak and V. Syritski, Advances in detection of antibiotic pollutants in aqueous media using the molecular imprinting technique—a review, Biosensors, 2022, 12, 441 CrossRef CAS PubMed.
- R. Katiyar, C.-W. Chen, R. R. Singhania, M.-L. Tsai, G. D. Saratale, A. Pandey, C.-D. Dong and A. K. Patel, Efficient remediation of antibiotic pollutants from the environment by innovative biochar: current updates and prospects, Bioengineered, 2022, 13, 14730–14748 CrossRef CAS PubMed.
- Y. Chen, J. Yang, L. Zeng and M. Zhu, Recent progress on the removal of antibiotic pollutants using photocatalytic oxidation process, Crit. Rev. Environ. Sci. Technol., 2022, 52, 1401–1448 CrossRef CAS.
- C. Monahan, S. Harris, D. Morris and E. Cummins, A comparative risk ranking of antibiotic pollution from human and veterinary antibiotic usage–An Irish case study, Sci. Total Environ., 2022, 826, 154008 CrossRef CAS PubMed.
- R. B. González-González, A. Sharma, R. Parra-Saldívar, R. A. Ramirez-Mendoza, M. Bilal and H. M. Iqbal, Decontamination of emerging pharmaceutical pollutants using carbon-dots as robust materials, J. Hazard. Mater., 2022, 423, 127145 CrossRef PubMed.
- M. M. Stanley, S.-F. Wang, J. N. Baby, B. Sriram and M. George, Deep eutectic solvent assisted synthesis of molybdenum nitride entrapped graphene aerogel heterostructure with enhanced electrochemical behavior for ronidazole drug detection, J. Mol. Liq., 2023, 375, 121308 CrossRef.
- J. M. Steiner, S. Schwamberger, N. Pantchev, H.-J. Balzer, M. G. Vrhovec, M. Lesina and H. Algül, Use of ronidazole and limited culling to eliminate Tritrichomonas muris from laboratory mice, J. Am. Assoc. Lab. Anim. Sci., 2016, 55, 480–483 Search PubMed.
- S. R. Andersen, N. M. Shukri, J. Boel and P. Saadbye, Metronidazole resistance in Campylobacter jejuni from poultry meat, J. Food Prot., 2006, 69, 932–934 CrossRef CAS PubMed.
- R. Fiechter, P. Deplazes and M. Schnyder, Control of Giardia infections with ronidazole and intensive hygiene management in a dog kennel, Vet. Parasitol., 2012, 187, 93–98 CrossRef CAS PubMed.
- C. Han, J. Chen, X. Wu, Y.-W. Huang and Y. Zhao, Detection of metronidazole and ronidazole from environmental samples by surface-enhanced Raman spectroscopy, Talanta, 2014, 128, 293–298 CrossRef CAS PubMed.
- X. Z. Wang, X. Y. Mao, Z. Q. Zhang, R. Guo, Y. Y. Zhang, N. J. Zhu, K. Wang, P. P. Sun, J. Z. Huo and X. R. Wang, Solvothermal and ultrasonic preparation of two unique cluster-based Lu and Y coordination materials: metal–organic framework-based ratiometric fluorescent biosensor for an ornidazole and ronidazole and sensing platform for a biomarker of amoeba liver abscess, Inorg. Chem., 2020, 59, 2910–2922 CrossRef CAS PubMed.
- A. AbdelKhalek and M. N. Seleem, Repurposing the veterinary antiprotozoal drug ronidazole for the treatment of Clostridioides difficile infection, Int. J. Antimicrob. Agents, 2020, 56, 106188 CrossRef CAS PubMed.
- B. Sriram, J. N. Baby, S.-F. Wang, M. R. Ranjitha, M. Govindasamy and M. George, Eutectic solvent-mediated synthesis of NiFe-LDH/sulfur-doped carbon nitride arrays: investigation of electrocatalytic activity for the dimetridazole sensor in human sustenance, ACS Sustainable Chem. Eng., 2020, 8, 17772–17782 CrossRef CAS.
- T. Kokulnathan, T.-W. Chen, S.-M. Chen, F. Ahmed, P. Hasan, A. Bilgrami and S. Kumar, A robust combination of dysprosium vanadate/halloysite nanotubes: the electrochemical system for dimetridazole detection, Mater. Today Chem., 2022, 24, 100890 CrossRef CAS.
- T. S. Priya, K. Palanichamy, S.-M. Chen, T.-W. Chen, A. J. Anthuvan and J. Yu, Exfoliated 2D Graphitic-Carbon Nitride Nanosheets as Sensor for Electrochemical Detection of Furazolidone, Int. J. Electrochem. Sci., 2022, 17, 220842 CrossRef CAS.
- V. Velusamy, S. Palanisamy, T. Kokulnathan, S.-W. Chen, T. C. Yang, C. E. Banks and S. K. Pramanik, Novel electrochemical synthesis of copper oxide nanoparticles decorated graphene-β-cyclodextrin composite for trace-level detection of antibiotic drug metronidazole, J. Colloid Interface Sci., 2018, 530, 37–45 CrossRef CAS PubMed.
- T. Liu, J. Liu, L. Zhang, B. Cheng and J. Yu, Construction of nickel cobalt sulfide nanosheet arrays on carbon cloth for performance-enhanced supercapacitor, J. Mater. Sci. Technol., 2020, 47, 113–121 CrossRef CAS.
- G. B. Shombe, M. D. Khan, J. Choi, R. K. Gupta, M. Opallo and N. Revaprasadu, Tuning composition of CuCo 2 S 4–NiCo 2 S 4 solid solutions via solvent-less pyrolysis of molecular precursors for efficient supercapacitance and water splitting, RSC Adv., 2022, 12, 10675–10685 RSC.
- D. P. Sahoo, K. K. Das, S. Patnaik and K. Parida, Double charge carrier mechanism through 2D/2D interface-assisted ultrafast water reduction and antibiotic degradation over architectural S, P co-doped gC 3 N 4/ZnCr LDH photocatalyst, Inorg. Chem. Front., 2020, 7, 3695–3717 RSC.
- S. Mansingh, D. P. Sahoo, L. Paramanik, M. Sahoo and K. Parida, Robust charge carrier engineering via plasmonic effect and conjugated Π-framework on Au loaded ZnCr-LDH/RGO photocatalyst towards H 2 and H 2 O 2 production, Inorg. Chem. Front., 2022, 9, 559–576 RSC.
- T. Kokulnathan, T.-J. Wang, F. Ahmed and T. Alshahrani, Hydrothermal synthesis of ZnCr-LDH/tungsten carbide composite: a disposable electrochemical strip for mesalazine analysis, Chem. Eng. J., 2023, 451, 138884 CrossRef CAS.
- M. T. Mirzaee, S. Seidi and R. Alizadeh, Pipette-tip SPE based on Graphene/ZnCr LDH for Pb (II) analysis in hair samples followed by GFAAS, Anal. Biochem., 2021, 612, 113949 CrossRef CAS PubMed.
- B. Sriram, V. A. Sherlin, S.-F. Wang, Y. F. Hsu and M. George, Hierarchical 3D architecture NiCo–layered double hydroxide decorated functionalized halloysite nanotubes composite. An efficient electrocatalyst for ractopamine detection, Appl. Clay Sci., 2023, 242, 107028 CrossRef CAS.
- R. B. Shinde, A. S. Patil, S. V. Sadavar, Y. M. Chitare, V. V. Magdum, N. S. Padalkar, U. M. Patil, S. T. Kochuveedu, V. G. Parale and H.-H. Park, Polyoxotungstate intercalated self-assembled nanohybrids of Zn-Cr-LDH for room temperature Cl2 sensing, Sens. Actuators, B, 2022, 352, 131046 CrossRef CAS.
- E. Akbarzadeh, M. Rasteh and M. R. Gholami, Visible light photocatalytic performance of Ag2O/ZnCr-LDH nanocomposite, Chem. Phys. Lett., 2020, 751, 137558 CrossRef CAS.
- R. Yang, B. Liang, S. Zheng, C. Hu, Y. Xu, Y. Ma, Y. Bai, K. Dai, Y. Tang and C. Zhang, 3D hierarchical structure collaborating with 2D/2D interface interaction in BiVO4/ZnCr-LDH heterojunction with superior visible-light photocatalytic removal efficiency for tetracycline hydrochloride, Arabian J. Chem., 2023, 16, 104397 CrossRef CAS.
- X. B. Joseph, J. N. Baby, S.-F. Wang, B. Sriram and M. George, Interfacial superassembly of Mo2C@ NiMn-LDH frameworks for electrochemical monitoring of carbendazim fungicide, ACS Sustainable Chem. Eng., 2021, 9, 14900–14910 CrossRef CAS.
- T. Kokulnathan, T.-J. Wang, F. Ahmed and N. Arshi, Fabrication of flower-like nickel cobalt-layered double hydroxide for electrochemical detection of carbendazim, Surf. Interfaces, 2023, 36, 102570 CrossRef CAS.
- B. Sriram, J. N. Baby, Y.-F. Hsu, S.-F. Wang and M. George, Surfactant-assisted synthesis of praseodymium orthovanadate nanofiber-supported nife-layered double hydroxide bifunctional catalyst: the electrochemical detection and degradation of diphenylamine, Inorg. Chem., 2022, 61, 5824–5835 CrossRef CAS PubMed.
- D. P. Sahoo, S. Patnaik and K. Parida, Construction of a Z-scheme dictated WO3–X/Ag/ZnCr LDH synergistically visible-light-induced photocatalyst towards tetracycline degradation and H2 evolution, ACS Omega, 2019, 4, 14721–14741 CrossRef CAS PubMed.
- Y. Cui, J. Ma, M. Wu, J. Wu, J. Zhang, Y. Xu, Q. Liu and G. Qian, Facet-dependent topo-heterostructure formed by BiOCl and ZnCr-LDH and its enhanced visible-light photocatalytic activity, Sep. Purif. Technol., 2021, 254, 117635 CrossRef CAS.
- T. Kokulnathan, T.-J. Wang, F. Ahmed and T. Alshahrani, Synthesis of 3D Flower-Like Zinc-Chromium Layered Double Hydroxides: A Functional Electrode Material for Furaltadone Detection, Process Saf. Environ. Prot., 2023, 176, 889–897 CrossRef CAS.
- P. Cai, T. Liu, L. Zhang, B. Cheng and J. Yu, ZIF-67 derived nickel cobalt sulfide hollow cages for high-performance supercapacitors, Appl. Surf. Sci., 2020, 504, 144501 CrossRef CAS.
- B. Sriram, J. N. Baby, S.-F. Wang, M. George, X. B. Joseph and J.-T. Tsai, Surface engineering of three-dimensional-like hybrid AB2O4 (AB= Zn, co, and Mn) wrapped on sulfur-doped reduced Graphene oxide: investigation of the role of an Electrocatalyst for Clioquinol detection, ACS Appl. Electron. Mater., 2020, 3, 362–372 CrossRef.
- M. Zhang, H. Du, Z. Wei, X. Zhang and R. Wang, Ultrafast microwave synthesis of nickel-cobalt sulfide/graphene hybrid electrodes for high-performance asymmetrical supercapacitors, ACS Appl. Energy Mater., 2021, 4, 8262–8274 CrossRef CAS.
- X. Li, W. Yan, S. Guo, Y. Liu, J. Niu, L. Yin and Z. Wang, One-step electrochemical controllable preparation of nickel cobalt sulfide nanosheets and its application in supercapacitors, Electrochim. Acta, 2021, 387, 138488 CrossRef CAS.
- T. Van Nguyen, L. T. Son, P. M. Thao, D. T. Phat, N. T. Lan, N. Van Nghia and T. V. Thu, One-step solvothermal synthesis of mixed nickel–cobalt sulfides as high-performance supercapacitor electrode materials, J. Alloys Compd., 2020, 831, 154921 CrossRef.
- J. Gong, Y. Wang, J. Wang, Y. Liu, C. Hu, T. Zhou, Q. Yu, J. Yang and Y. Dai, Hollow nickel-cobalt sulfide nanospheres cathode hybridized with carbon spheres anode for ultrahigh energy density asymmetric supercapacitors, Int. J. Hydrogen Energy, 2022, 47, 10056–10068 CrossRef CAS.
- Y. He, C. Dong, S. He, H. Li, X. Sun, Y. Cheng, G. Zhou and L. Xu, Bimetallic nickel-cobalt sulfides with hierarchical coralliform architecture for ultrafast and stable Na-ion storage, Nano Res., 2021, 14, 4014–4024 CrossRef CAS.
- X. Chen, Q. Liu, T. Bai, W. Wang, F. He and M. Ye, Nickel and cobalt sulfide-based nanostructured materials for electrochemical energy storage devices, Chem. Eng. J., 2021, 409, 127237 CrossRef CAS.
- L. Chen and J. Zheng, Two-step hydrothermal and ultrasound-assisted synthesis of CB/NiCo2S4@ CeO2 composites for high-sensitivity electrochemical detection of nitrite, Microchem. J., 2022, 181, 107717 CrossRef CAS.
- C. Xing, F. Musharavati, H. Li, E. Zalezhad, O. K. Hui, S. Bae and B.-Y. Cho, Synthesis, characterization, and properties of nickel–cobalt layered double hydroxide nanostructures, RSC Adv., 2017, 7, 38945–38950 RSC.
- D. Chen, H. Wang and M. Yang, A novel ball-in-ball hollow NiCo 2 S 4 sphere based sensitive and selective nonenzymatic glucose sensor, Anal. Methods, 2017, 9, 4718–4725 RSC.
- L. Shen, L. Yu, H. B. Wu, X.-Y. Yu, X. Zhang and X. W. Lou, Formation of nickel cobalt sulfide ball-in-ball hollow spheres with enhanced electrochemical pseudocapacitive properties, Nat. Commun., 2015, 6, 6694 CrossRef CAS PubMed.
- T. Zhu, G. Zhang, T. Hu, Z. He, Y. Lu, G. Wang, H. Guo, J. Luo, C. Lin and Y. Chen, Synthesis of NiCo 2 S 4-based nanostructured electrodes supported on nickel foams with superior electrochemical performance, J. Mater. Sci., 2016, 51, 1903–1913 CrossRef CAS.
- C. Shuai, Z. Mo, X. Niu, X. Yang, G. Liu, J. Wang, N. Liu and R. Guo, Hierarchical NiCo2S4 nanosheets grown on graphene to catalyze the oxygen evolution reaction, J. Mater. Sci., 2020, 55, 1627–1636 CrossRef CAS.
- H. L. Tcheumi, A. P. Kameni Wendji, I. K. Tonle and E. Ngameni, A Low-Cost Layered Double Hydroxide (LDH) Based Amperometric Sensor for the Detection of Isoproturon in Water Using Carbon Paste Modified Electrode, J. Anal. Methods Chem., 2020, 2020, 8068137 Search PubMed.
- M. Coros, C. Varodi, F. Pogacean, E. Gal and S. M. Pruneanu, Nitrogen-Doped Graphene: The Influence of Doping Level on the Charge-Transfer Resistance and Apparent Heterogeneous Electron Transfer Rate, Sensors, 2020, 20, 1815 CrossRef CAS PubMed.
- T. Kokulnathan, T.-J. Wang, E. A. Kumar, N. Duraisamy and L. An-Ting, An electrochemical platform based on yttrium oxide/boron nitride nanocomposite for the detection of dopamine, Sens. Actuators, B, 2021, 349, 130787 CrossRef CAS.
- H. R. Zare, N. Nasirizadeh and M. Mazloum Ardakani, Electrochemical properties of a tetrabromo-p-benzoquinone modified carbon paste electrode. Application to the simultaneous determination of ascorbic acid, dopamine, and uric acid, J. Electroanal. Chem., 2005, 577, 25–33 CrossRef CAS.
- B. Karupppaiah, A. Jeyaraman, S.-M. Chen and Y.-C. Huang, Development of highly sensitive electrochemical sensor for antibiotic drug ronidazole based on spinel cobalt oxide nanorods embedded with hexagonal boron nitride, Electrochim. Acta, 2023, 446, 142008 CrossRef CAS.
- Y.-K. Lu, H.-H. Wang, Q.-X. Hu, Y.-Y. Ma, L. Hou and Y.-Y. Wang, A stable Cd(II)-based MOF with efficient CO2 capture and conversion, and fluorescence sensing for ronidazole and dimetridazole, J. Solid State Chem., 2021, 295, 121890 CrossRef CAS.
- M.-Y. Jiang, L. Yu, Y.-C. Zhou, J. Jia, X.-J. Si, W.-W. Dong, Z.-F. Tian, J. Zhao and D.-S. Li, A Novel d-f Heterometallic CdII-EuIII Metal-organic Framework as a Sensitive Luminescent Sensor for the Dual Detection of Ronidazole and 4-Nitrophenol, Z. Anorg. Allg. Chem., 2020, 646, 268–274 CrossRef CAS.
- B. Karuppaiah, A. Jeyaraman, S.-M. Chen, P. R. Chavan, R. Karthik, J.-J. Shim and S. J. Park, Design and synthesis of nickel-doped cobalt molybdate microrods: An effective electrocatalyst for the determination of antibiotic drug ronidazole, Environ. Res., 2023, 222, 115343 CrossRef CAS PubMed.
|
This journal is © The Royal Society of Chemistry 2024 |