DOI:
10.1039/D2MA00527A
(Review Article)
Mater. Adv., 2022,
3, 7757-7772
Intelligent hydrogels and their biomedical applications
Received
11th May 2022
, Accepted 24th August 2022
First published on 23rd September 2022
Abstract
Intelligent biomaterials can modify their properties in response to physical, chemical, and biological stimuli. These smart characteristics drive the innovation of biomaterials in therapy and diagnostics for detecting diseases and providing treatment at early stages. Mainly, hydrogels have gained significant interest in developing smart materials due to their excellent biocompatibility and ability to interact with body fluids that host condition-specific stimuli. Temperature, pressure, pH, light, ROS, cell metabolites, and other physicochemical factors specific to specific disease conditions were studied as major stimuli for designing intelligent biomaterials. The stimuli-responsive characteristic mainly depends on the sensitivity of the biomaterial to the stimuli factor and the tunable macromolecular structure of the materials. The method of biomaterial fabrication is critical in determining the physical and chemical properties of the biomaterial. Surface functionalisation, material blending, and crosslinking are commonly used to synthesise intelligent hydrogels to change the macromolecular structure. The impact and mechanism of these fabrication methods on the macromolecular structure and stimuli responsiveness of intelligent materials remain unidentified. This review focuses on strategies for transforming conventional hydrogels into intelligent hydrogels, their concerning mechanisms of stimuli-responsiveness and their biomedical applications.
1. Introduction
Intelligence or smartness refers to the capacity to understand and adapt based on external factors or senses. The ability to change/modify based on external factors is often considered intelligence in general. Further, the concept is applicable in materials science to induce intelligence by incorporating sensitive moieties into the material. Therefore, the concept of intelligence is adopted in computer science engineering as “artificial intelligence”, whereas materials science adopts “intelligent biomaterials”.1 Intelligent materials can respond and modify the materials based on external factors like light, temperature, pressure, pH, glucose, reactive oxygen species (ROS) and several other factors. Owing to the ability to understand and adapt to external factors, intelligent biomaterials have diverse applications in biosensors, medical implants, medical devices, data storage, intelligent textiles, drug delivery and the development of artificial skin. Here, we discuss intelligent hydrogels, strategies for inducing intelligence in hydrogels, and the biomedical applications of smart/intelligent hydrogels.1,2
Biomedical applications increasingly rely on stimuli-responsive materials activated by physical/chemical and biological signals, and such materials are referred to as “intelligent/smart biomaterials”.3 Intelligent biomaterials can dynamically adjust physiological factors and respond to exogenous stimuli, and they remain to impact many facets of medical advances. Smart materials can accelerate the development of innovative medicines and enhance the treatments for chronic diseases.4 One of the major problems in clinical biomaterials is the poor understanding of the biomaterial to the microenvironment of the disease, which necessitates the design of a biomaterial with induced responsiveness to the environmental signals.5 Therefore, the development of intelligent hydrogels that understand the underlying mechanism based on the stimuli responses signified by the diseased cells/conditions is widespread. Stimuli-responsive behaviour is a significant advantage of intelligent biomaterials supporting clinical research in future.
The field of regenerative medicine combines materials science, engineering, chemistry, and biology to bring solutions to repair and restore the functions of damaged tissues.6 Hydrogels have been identified as one of the promising biomaterials for drug delivery and tissue regeneration because they address biocompatibility and functionality and possess adjustable features.7 On the other hand, hydrogels are 3D hydrophilic polymeric substances crosslinked in an aqueous medium. The ability of hydrogels to hold a high water content contributes to their excellent physicochemical properties, including permeability, large specific surface areas, thermal insulation, and controllable mechanical and optical properties.8,9 In addition, the structural and physiological capacity of hydrogels also provides cell support and mimics the extracellular matrix (ECM) architecture.10 Based on the choice of polymer, hydrogels are classified as natural, synthetic, decellularised or hybrid hydrogels; cationic, anionic, or neutral hydrogels; or chemically or physically crosslinked hydrogels.11 In addition, low, high, and superabsorbent hydrogels have different absorption rates.
Further, hydrogels can be classified as micro-, macro-, or ultra-porous depending on the material's origin, gel formation mechanism, side group functionalities,12 physicochemical properties, and biodegradability.13,14 Hydrogels have been used as prominent tissue engineering scaffolds over the decades. Hydrogel's high-water holding capacity resembles the host tissue microenvironment, thus enabling the potential for biomedical applications. Further, hydrogels have significant applications as a support system in cell immobilisation and the delivery of growth factors through pore distribution.15 The properties of hydrogels and types of polymers used are illustrated in Fig. 1.
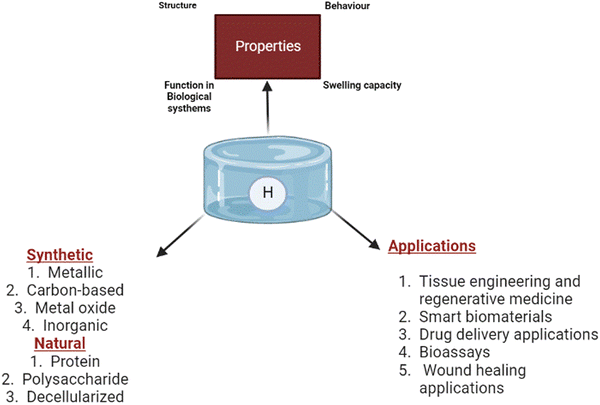 |
| Fig. 1 General illustration of the properties of hydrogels, types of polymers used and their biomedical applications. | |
Intelligent hydrogels, also known as stimuli-responsive hydrogels, are three-dimensional networks made up of polymerised hydrophilic polymer networks that may substantially vary their volumes and other properties in response to external stimuli like temperature, pH, and specific chemical signals.16 The adaptability of smart hydrogels depends on their ability to respond quickly and effectively to environmental cues while also having a high degree of flexibility.17 Multi-stimulus responsiveness helps them to have versatile tissue engineering applications. As a result, intelligent hydrogels are highly preferred as they understand the physicochemical/biochemical aspects of a disease mechanism by sensing stimuli responses and alter the biomaterial accordingly. Recent technologies have made it possible to design hydrogels to respond to specific biological cues by introducing gene-editing techniques like the clustered regularly interspaced short palindromic repeats (CRISPR–Cas9) approach using RNA-defined inputs.18
Here we describe the (1) intelligent biomaterials: design strategies and functionalities, (2) mechanism of biotransformation of a hydrogel to an intelligent hydrogel, (3) synthesis of multi-stimuli-responsive hydrogels, and (4) current trends and biomedical application of intelligent hydrogels.
1.1. Intelligent biomaterials: design criteria and functionality strategies
Implementing intelligent biomaterials sensitive to external stimuli in biological systems has been a new emphasis in tissue regeneration research. The insertion of specific molecules (sensitive/reactive) into biomaterials allows control over their architectural, pharmacological, and physicochemical features, according to one technique for developing intelligent biomaterials for biotherapeutics.19 This programming of hydrogels boosts the functionalities, including cell attachment, proliferation, and migration.20 As a result, the synthetic methodologies used to fabricate intelligent biomaterials must be carefully studied, with nature and biomimetic strategies or click-based orthogonal methods being two viable options. Because of their simplicity, convenience, and productivity, click-based orthogonal approaches are frequently used to execute various physiological and morphological properties within hydrogels.21,22 These changes can make materials more sensitive to environmental stimulation, allowing them to perform or activate specific functions or physical and chemical changes in response to interactions with receptors, enzymes, cells, or other stimuli.23 The irreversible or reversible copolymerisation procedures can intercalate the polymeric matrix.24
Further, hydrogels can undergo structural changes in response to external stimuli attributable to the reversible bridge via physical crosslinking, thermally induced polymer chain entanglement, and self-assembly.25 It is frequently necessary to use both reversible and irreversible crosslinking. The irreversible bonding strengthens the structural integrity of biomaterials, whereas the reversible (bidirectional) bonding mainly constitutes self-healing characteristics.26,27 Apart from mechanical and chemical versatility, the biomaterial used in biomedical applications should also be biocompatible, which is possible through the biomimetic approach.28
Shape memory is one of the distinct features of intelligent biomaterials. Injectable shape-memory hydrogels are built to deliver drugs to viable tissues through a minimally invasive treatment strategy.1 The flexible materials were microfabricated, and the biological material maintained its basic shape after being injected with cells.29 Following exposure to the desired stimulation, biomaterials with shape memory and self-healing behaviour can return to their previous functioning and structure.30 For instance, a change in temperature or physical strain causes the change.8 In general, self-healing biomaterials could prevent sudden damage to an implant or coating by quickly reverting to their native structure, creating a favourable microenvironment for tissue ingrowth and cellular mobility, and plausibly strengthening the tissue-regenerative ability of the material/scaffold.31,32 Since hydrogels are frequently utilised as biological scaffolds, the frameworks must degrade in vivo once cellular integration with nearby tissues is accomplished.15,33,34 In most biodegradable hydrogel systems, stimulus-specific cleavable crosslinkers are used, which can be disassembled in response to stimulation by hydrolysis protein denaturation.35Fig. 2 illustrates the hydrogel networking pattern with the distribution of the polymeric network with cells and the sensitive moieties to display the responsive properties. To state clearly, intelligence is induced in the hydrogel by incorporating sensitive moieties using appropriate design parameters. The design parameters include physical, chemical and biological techniques in introducing specificity to certain environmental factors. Crosslinking and conjugating specific molecules in the interconnected polymeric network enhance the specificity of the hydrogel when exposed to such stimuli. Therefore, designing and synthesising biomaterials is significant in fabricating intelligent biomaterials.2Fig. 2 illustrates the behaviour and composition of the hydrogel when subjected to external signals/stimuli.
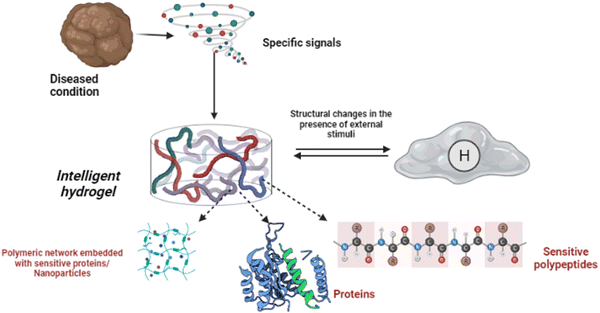 |
| Fig. 2 Support hydrogel matrix to sense specific external stimuli/factors responsible for stimuli responsiveness. Illustration of the hydrogel networking pattern with the distribution of the polymeric network, cells, and sensitive moieties to display the bioresponsive properties. | |
1.2. Inducing intelligence in hydrogels
Humans have the cognitive ability to reason and understand, analyse and find solutions, which also help them invent and develop strategies and communicate and connect with other individuals.36 Human intelligence is defined by the capacity to recognise, address, and draw lessons from issues in various contexts. Similar to how the parallel interpretation led to this conclusion, a material's “intelligence” is defined as its capacity to modify its properties in response to external cues and undergo matching molecular-level structural rearrangement. One definition of the concept of intelligence of a polymer is a substance that understands experiences, is self-aware, and reacts purposefully. The intelligence in hydrogels can be a characteristic property of the polymers or the sensitivity moiety introduced while designing the hydrogels.2 When viewed from a macroscopic perspective, the independent behaviour of atoms or molecules at a subatomic scale enables the reactive behaviours of intelligent materials.37 The hydrogel behaviour changes from the nanoscale level and changes structurally and morphologically by rearranging, aggregating and causing the directional movement of the polymers. Intelligence depends majorly on the property of the polymer used or the design traits by introducing sensitive moieties to specific responses.38Fig. 3 illustrates the behaviour of intelligent hydrogels, and the classification of intelligent polymers is also shown.
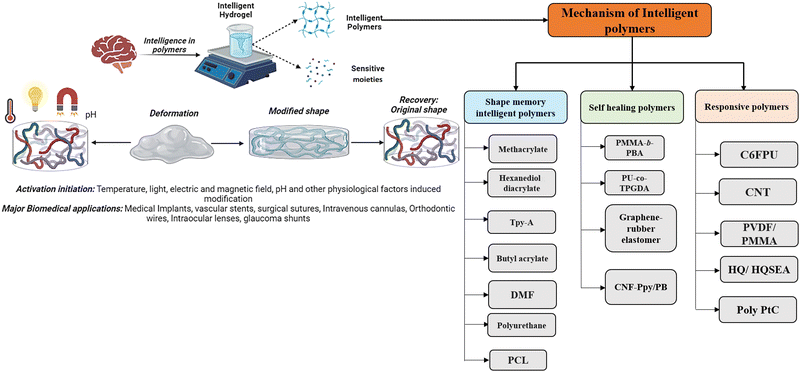 |
| Fig. 3 Illustration of intelligence factors in intelligent hydrogels; the behaviour of the hydrogel when exposed to external elements and the classification of polymers that possess the intrinsic ability to adapt to external stimuli. Abbreviations: DMF – dimethylformamide, PCL – polycaprolactone, PMMA-b-PBA – poly(methyl methacrylate)-b-poly(butyl acrylate), PU-co-TPGDA – polyurethane-co-tripropylene glycol diacrylate, CNF-Ppy/PB – cellulose nanofiber-polypyrrole/PVA-borate, C6FPU – fluorinated polyurethane, CNT – carbon nanotube reinforced hydrogel, PVDF/PMMA – polyvinylidene fluoride/polymethyl methacrylate, HQ/HQSEA – hydroquinone/8-quinolinyl-sulfide-ethyl acrylate, Poly PTC – polymeric positive temperature coefficient. | |
In the classification mentioned above, the memorised phase of a thermosensitive shape memory polymer has a less-organised structure when the ambient temperature is lower. The flipping sections are extended and set in the polymers, while the molecular linkages are relaxed and steady with minimal heat flux in the original shape. Because the molecular motion is active and the network orientations are switching, and due to the dislocation of net points, the polymers exhibit viscoelastic properties when the temperature increases—the thermal impact results in the development of interactions among shape memory polymeric chains.38 In the case of self-healing polymers, their self-healing capacity can come from either their external or innate properties. Most patients use extrinsic healing, in which the healing substances are encapsulated in beads or nanoparticles apart from the polymer matrix.39,40
In contrast, endogenous self-healing polymers change the arrangement of molecular chains to take advantage of their mobility. The term “self-healing” originates from the fact that the natural functionalities, such as conductance, coherence of the surface morphology, or other physical characteristics of the biomaterial, are retrieved.38–40 Responsiveness of a hydrogel to external stimuli like electrical conductivity, magnetic field, humidity, reactive oxygen species, and carbon dioxide depends on the polymer used for hydrogel preparation or the introduction of sensitive/responsive substances while formulating the hydrogel. In addition, the detailed synthesis of the hydrogel is mentioned below.
2. Biotransformation to intelligent hydrogels
Traditional small-molecule chemically crosslinked biomaterials have a delayed reaction speed and weak flexibility, significantly limiting the application of hydrogels.41 To biotransform the conventional hydrogel into an intelligent hydrogel, different crosslinking methods are carried out physically/chemically to fabricate hydrogels.42 The modification is caused by covalent interactions such as differently charged ions, hydrophobic interactions, π–π interactions, electrostatic interactions, van der Waals as dipole–dipole interactions, and London dispersion forces.43,44 Further, some injectable hydrogels can be prepared by physical self-crosslinking via exchanges such as host–guest interactions.42–44 Also, the diverse environmental stressors frequently impact crosslinking-induced gelation.45
Further, to tailor the hydrogel to be sensitive to a specific disease or protein, the hydrogel matrix is programmed using CRISPR–Cas9, a well-known genome editing tool.46 CRISPR has been a well-known genome editing/engineering technique in recent times. Further, to adapt to the customisable nuclease Cas12a, researchers produced a set of stimuli-responsive hydrogels. RNA-guided endonucleases capable of multiple-turnover nucleic acid hydrolysis are a feature of the adaptive immune systems of microbes, as are CRISPR and CRISPR-associated (Cas) genes.18 CRISPR-Cas enzymes have been used as effective genome editing tools due to their sensitivity and flexibility. Soft biomaterials (hydrogels) are programmed using CRISPR-associated nucleases to control hydrogels that contain DNA as a structural component or as a hanging group attachment. Cas12a cleaves DNA in gels after being activated by guide RNA-defined inputs, translating biological information into changes in material characteristics.47 Here, the particular DNA responsible for the mechanism of disease treatments is introduced in the hydrogel to act or deliver at the target site. Following Cas12a-dependent cleavage of double- or single-stranded DNA inserted into the gel, the materials undergo microscopic to macroscopic modifications.48,49Fig. 4 explains the crosslinking mechanism of transforming a hydrogel into an intelligent hydrogel in physical, chemical and molecular aspects.
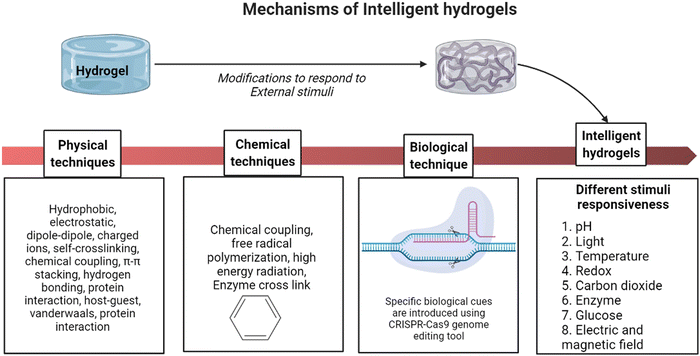 |
| Fig. 4 The mechanism of inducing intelligence in the hydrogels using different techniques/methodologies (physical, chemical and biological); the type of interaction and design approach are shown in the illustration to induce other stimuli-responsive behaviours in the biomaterial. | |
Incorporating bioactive molecules in the hydrogel enhances the mechanical strength of the scaffold. Glycosaminoglycans (GAGs) are a vital component of cartilage ECM that gives power to the architecture of the host.50 Developing a multifunctional biomaterial that can serve to integrate several biomaterials is functionally improved. Hydrogels are introduced with molecules sensitive to specific cells and tissue to control cellular behaviour and enhance the function.51 Raising RGD motifs in the hydrogel facilitates cell adhesion and increases proliferation, differentiation, migration, and expansion.52
Furthermore, smart biomaterials have been employed as tissue glue, connecting and stimulating tissue healing by inserting reactive groups and fibroblast materials like proteoglycans, collagens, and fibronectin.53 The methacrylate organic molecules of the biomaterial improve the mechanical properties by photopolymerisation. At the same time, the aldehydes aid cell integration and adherence via a reaction of Schiff bases with the amines on the tissue surface.53,54 Studies have focused on developing biomaterials with enhanced response properties or elastic modulus to circumvent such constraints. The side chains are modified to improve the responsiveness of hydrogels to fabricate comb-type hydrogels with porous networks with a separate water pathway in the hydrogels.10,43 Another strategy is developing double-network hydrogels with two interpenetrating networks or employing exfoliated clay nanoparticles as crosslinkers in nanocomposite hydrogels to improve elasticity.55 Although double-network hydrogels are very stretchy, interactions between the polymers severely decrease the stimuli-sensitivity of each polymer in the interpenetrating networks.56
Nanocomposite hydrogels with clay networks display better stimuli-responses, but their thermoresponsive equilibrium is limited. More recently, biomolecular microspheres have been employed as fundamental building blocks or covalent crosslinkers to create highly flexible hydrogels.57 On the other hand, all macromolecular microspheres were used to make microspheres. Reinforced composite hydrogels can have a size of sub-microns or even microns, far more significant than clay nanoparticles.55,58 This could be a problem for microsphere-composite hydrogels that require a high modulus of elasticity while also having a susceptible swelling ratio. Synthesis of intelligent hydrogels with a high responsive swelling ratio, fast response rate, and high elasticity has remained a challenge until now.10,41
CRISPR nucleases can activate hydrogels. Cas12a cleaves DNA in gels after being triggered by guided RNA-defined inputs, translating the biological material into different blend characteristics. CRISPR inserts specific DNA as a structural element or pendant group anchors Cas12a.59,60 Therefore, a well-established and successful gene-editing tool, CRISPR–Cas9, can be used to program intelligent hydrogels for targeted gene/protein communication.
2.1. Novel hydrogel materials for drug delivery applications
Intelligent hydrogels have potential application in drug delivery systems in a specific site of the host system.5 Bioresponsive hydrogels are materials that vary their characteristics in response to exposure, interaction, and concentrations of distinct molecular variables, such as swelling/shrinking behaviour, corrosion sensitivity, or disintegration.61 Biological-stimuli biomaterials are classified into three categories based on the activation factors: responsiveness to glucose, specific enzymes, or antibody-sensitive. Glucose oxidase (GOX) and pH-responsive materials are used to construct insulin-releasing biopolymers reacting to glucose level changes. When blood glucose levels rise, the polymer chain linked to GOX transforms it into gluconic acid and H2O2. Therefore, the pH inside the hydrogel architecture drops, ionisation of certain positively charged functional groups develops, the material swells, and insulin penetrates the circulation.29 Novel polymers used in the preparation of thermoresponsive, pH-responsive and enzyme-responsive hydrogels are listed in Table 1.
Table 1 Novel polymers used in the preparation of thermoresponsive, pH-responsive and enzyme-responsive hydrogels
Type of stimuli |
Polymer materials used |
Mechanism of stimuli responsiveness |
Ref. |
Thermoresponsive hydrogel |
Poly(ethylene oxide)–poly(propylene oxide)–poly(ethylene oxide) [PEO–PPO–PEO, poloxamer] |
|
62–71
|
Poly(ε-caprolactone)–poly(ethylene glycol)–poly(ε-caprolactone) [PCL–PEG–PCL, PCEC] |
Poly(ethylene glycol)methyl ether–poly(ε-caprolactone)–poly(ethylene glycol)methyl ether [mPEG–PCL–mPEG, PECE] |
Poly(ethylene glycol) methyl ether–poly(lactic acid-co-glycolic acid)–poly(ethylene glycol)methyl ether [mPEG–PLGA–mPEG] |
Poly(organofosfazene) bearing α-amino-ω-methoxy-PEG and L-isoleucine ethyl ester[NP(AMPEG350)(IleOEt)]n |
pH-sensitive hydrogel |
Acrylic acid derivatives |
|
2 and 72–79
|
Poly(acrylic acid) |
Poly(methacrylic acid) |
Poly(ethylacrylic acid) |
Poly(propyl acrylic acid) |
Poly(butyl acrylic acid) |
2-Acrylamido-2-methylpropylsulfonic acid |
2-Methacryloxyethylsulfonic acid |
3-Methacrylic-2-hydroxypropylsulfonic acid |
Styrenesulfonic acid |
Sulfonamides |
Sulfapyridine |
Sulfisomidine |
Sulfamethoxypyridazine |
Sulfamethazine |
Sulfadimethoxine |
|
Polycation sensitive polymers |
|
|
Poly(N,N′-dimethyl aminoethyl methacrylate) PDMAEMA (PDMA) |
Poly(N,N′-diethyl aminoethyl methacrylate) PDEAEMA (PDEA) |
Poly(2-aminoethyl methacrylate) (PMAA) |
Poly(ethylene imine) (PEI) |
Poly(vinylamine) (PVAm) |
Poly(acrylamide) (PAAm) |
Pyridine and imidazole derivatives |
Poly(2-vinyl pyridine) (P2VP) |
Poly(4-vinyl pyridine) (P4VP) |
Enzyme responsive hydrogels |
MIONPs coated with acrylate-PEG-GGGPQGIWGQGK-PEG-acrylate, PEG-diacrylate, and acrylate-PEG-RGDS conjugates |
|
70 and 74
|
MMP-sensitive octapeptide copolymerised with Pluronic P104 |
3. Synthesis of multi-stimuli-responsive hydrogels
3.1. Thermoresponsive hydrogels (TRH)
Temperature-responsive biomaterials are among the most intelligent hydrogels because they can modify their structure and construction in reaction to temperature differences.80 The hydrogels undergo phase transitions with the temperature change. Hydrophobic regions such as methyl, ethyl, and propyl groups distinguish them. TRH are often positively thermosensitive, negatively thermosensitive, or thermally reversible. Positively thermosensitive hydrogels, such as crosslinking polymerisation connections (IPNs) built on poly(acrylamide-co-butyl methacrylate) [poly(N-isopropyl acrylamide) (P(NIPAAm-co-BMA)] and polyacrylic acid (PAAc), swell at high temperatures and shrink at low temperatures.81,82
On the other hand, negative temperature-dependent hydrogels expand when the temperature decreases and vice versa and this can be made from natural materials like gelatin, cellulose, and chitin and synthetic materials like poly(N-isopropyl acrylamide) (PNIPAAm) and polyfluorene polymers.83 Standard poly(N-isopropyl acrylamide) (PNIPAM) hydrogels linked covalently by chemical crosslinkers such as N,N-methylene bisacrylamide are indeed elastically weak and compress/swell slowly when heated/cooled to the low critical solution temperature (LCST). PNIPAM is a classic thermo-responsive intelligent biomaterial that can perform a changeable volume phase transition near the LCST.11,42,80 The alternatives to invasive operations include hybrid thermal hydrogels made of chitosan with collagen, PEG, and carboxy methylcellulose. Additionally, polymers can be used to deliver specific drugs into the bloodstream via fluid administration. When the body temperature rises above its low critical solution temperature, the polymer gels (LCST).84
3.2. Photoresponsive hydrogels (LRH)
When subjected to luminance such as ultraviolet rays, near-infrared, and visible light, hydrogels sensitive to light display bidirectional or reversible anomalies from a liquid to a gel state. Photosensitive moieties are found with one type of light responsive hydrogel (LRH), whereas near-IR absorptive nanomaterials are embedded in thermally sensitive hydrogels.85 Photochromism makes light-sensitive hydrogels by adding photosensitive molecules into the gel network. Chemical linking or dynamic mechanisms can be used, and the correct chromophores, wavelength, light intensity, and chromophore–polymer interactions can all help. The photoisomerisation of the photochrome causes this hydrogel to stretch and expand. In another work, metal nanoparticles, including gold, platinum, copper, and silver, were used to make light-sensitive hydrogels because they had better light adsorption and dispersion capabilities than polymer fluorophores. The photosensitivity of hydrogels depends on trans–cis photoisomerisation and sol–gel and gel–sol transitions.84,85 Photoinitiated azobenzene cis–trans isomerisation has been used in various gel structures and it was investigated how to make light-responsive hydrogels.86,88
3.3. Electrically conductive hydrogels
Electrosensitive hydrogels are commonly polymer mixtures or co-networks of conductive polymers and highly hydrophilic hydrogels that respond to electrical stimuli.87 The electroactive component is responsible for conductance and on–off optic–electronic switch, whereas the hydration component is responsible for expansion, cytocompatibility, and tiny molecular diffusion.89,90 A current gradient is maintained when a liquid covalently bonded polyelectrolyte gel is placed over two electrodes and exposed to DC power. The hydrogel shrinks randomly and depends on the charge releasing the moisture content.91 Biomaterials carrying ionic compounds undergo this compression process, but charged neutral hydrogels have no contraction.61 Hydrogels compress considerably towards the anode and enlarge to a minor degree near the cathode when polyanions are present. Electric field sensitive/responsive hydrogels, such as poly(2-(acrylamide)-2-methyl propane sulfonic acid) (PAMPS) hydrogels, were manufactured using free radicals and a chemical crosslinking technique.43,61,89
3.4. Magnetic responsive hydrogels
Magnetic responsive behaviour is usually achieved by dispersing magnetic NPs (MNPs) in a hydrogel matrix, which results in sensitive behaviour to magnetic stimuli.74 Because the magnet portion operates as a mixture with the gel network, the physical, thermal, and sonic behaviours are altered concurrently when the magnetism condition changes.92 Due to the addition of magnetic nanoparticles, the hydrogel usage is diversified through several fields. Furthermore, ensuring the homogeneous dispersion of magnetic nanoparticles to create a hybridised hydrogel matrix can achieve the desired results.93 As a result, in magnetic NPs implanted in hydrogels, it is essential to modify the dispersion of nanoparticles throughout the polymer matrix by establishing a magnetic field outside.94 These magnetic gels can be made by adding magnetic-responsive NPs into an inter-gel network. Further, to create a magnetic nanoparticle (MNP) hybridised hydrogel, three main fabrication procedures are used: mixing, entrapment/encapsulation, and grafting.95
The diffusion pattern strongly influences the magnetic and thermal behaviour of hydrogels when comparing biopolymers with randomly dispersed MNPs to those with homogeneously dispersed MNPs.95 Therefore, in preparing magnetic NPs implanted in a biopolymer, it is possible to redesign the distribution of NPs inside the hydrogel matrix by establishing magnetism outside. In addition, parameters like the size and concentration of both the polymer and particles can impact the characteristics of such a gel.96 As a result, magnetothermal factors may be controlled by regulating the magnetism and altering the distribution of MNPs within the hydrogel. MNPs can be constructed into a one-dimensional framework to improve their unified magnetism, dispersibility, and uniformity.96–98
3.5. pH-Responsive hydrogels
Many polymeric materials in thermoresponsive hydrogels modify their characteristics when exposed to pH. Acidic or basic moieties within polymeric chains can donate or accept protons.75 Thus, pH-sensitive materials are divided into polyanions and polycations, called polyacids and polybases. In alkaline conditions (above the pKa of polyacids), polyanions are deprotonated and the acidic groups are charged negatively. The reverse occurs in the particular instance of polycations. The modification of the polymer molecule effects as a function of ionisation and dissolution rates significantly increases. It turns out that ionisation causes the hydrogel to swell; primary carriers at high pH will absorb moisture. When the pH of the surrounding rises, the acidic moieties of polyanions are often negatively charged and begin to counteract one another. Moreover, hydrophobic interactions between chains destabilise, and hydrogen bonds are established among ionised groups and water molecules. As a result of dissociation, the osmotic pressure inside the hydrogel structure rises; an osmolarity gradient between the inner and outer layers of the polymeric matrix induces water to flow into the inner surface of the material, and the hydrogel swells.74,76
Polybases are a class of pH-sensitive biopolymers that comprise alkaline moieties that can be protonated in an acidic environment. The free-electron pair of the nitrogen atom constructs a coordinate bond with H+, which defines the basic properties of the polymeric matrix. Polymeric materials containing nitrogen-containing aromatic heterocycles are just another type of polycation. The single pair of electrons in the nitrogen atom is capable of proton binding. In this class, the most exhaustively studied polybasic smart polymers are pyridine-based or imidazole-based macromolecular derivatives. Primary, secondary, and tertiary polymerised amines, such as poly(N,N′-dimethyl aminoethyl methacrylate) (PDMAEMA), poly(2-aminoethyl methacrylate) (PMAA), and poly(ethylene imine), are among the most common polycations (PEI).74,99,100
Hydrogels with pH-responsive characteristics can be made from polymers derived from synthetic and natural sources and contain ionisable groups.101 Reactive oxygen species crosslinked chemically and oxygen radicals polymerised by physical crosslinking, grafted polymerisation, injection sol–gel processes, radiological polymerisation, template polymerisation, covalent linkages, click reactions, and other methods can all be used to prepare pH-responsive hydrogels.102 Polymeric materials with the ionic functional groups of –OH, –COOH, –NH2, –CONH2, –N, and –SO3 commonly produce pH-sensitive hydrogels.103 When a gel is subjected to a solvent with a specific pH and ionic strength, its pendant/functional groups become ionised with fixed charges, which display repulsive forces, resulting in a bidirectional swelling–deswelling mechanism dependent on the acidic or basic nature of the medium.104 The presence of cationic and anionic attachment sites in polymers controls pH depending on how hydrogels expand. Hydrogels with cationic pendant groups as opposed to those with anionic pendant groups.101,103,105Fig. 5 explains the hydrogel behaviour concerning the influence of temperature, ions and pH.
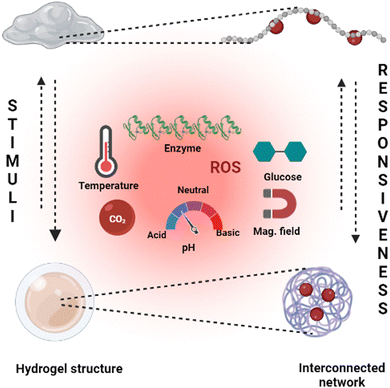 |
| Fig. 5 Graphical abstract illustrates the structural changes of a hydrogel based on the environmental stimuli and its behaviour based on the influence of the external stimuli like temperature, pressure, pH, glucose, light, ROS, antigen and pressure. | |
3.6. Redox responsive hydrogels
ROS are incredibly reactive reagents that are abundant in the human body. They determine cell signalling pathways but also cause oxidative damage to liquids, proteins, nucleic acids, and other things.106 As a result, high ROS production causes body functions to be disrupted and is linked to chronic complications. When an oxidant is introduced to a redox-responsive hydrogel, its viscosity decreases and it transitions to a solution phase; on the other hand, when a reductant like glutathione is introduced to this mixture, its fluidity decreases and it gels.107 Identifying reactive oxygen species (ROS) has evolved in redox responsive hydrogels, which aids cell-protective effects. Integrating redox-sensitive components into a thermally sensitive environment during fabrication is part of the synthesis of a redox-sensitive hydrogel.10,107,108 The crosslinking of a polymer generated by ring-opening polymerisation can be done by a thiol-yne reaction to embed the oxidative responsive features in the hydrogel.109 The resultant polymer can demonstrate oxidation sensitivity when exposed to substances such as hydrogen peroxide.110
3.7. Glucose-responsive site hydrogels
Hydrogels are widely employed in cutting-edge research, including monitoring blood glucose by the self to regulate the blood sugar levels of diabetic patients and as efficient insulin and therapeutic vehicles.111 Dynamic covalent connections are one of the most commonly used synthetic processes. The polyethylene oxide-b-polyvinyl pyrrolidone block polymer, cyclodextrin, and the phenylboronic acid (PBA)-terminated polyethylene oxide (PEO) crosslinker can be combined to prepare a hydrogel solution. The incorporation takes place between PEO and cyclodextrin, whereas polyvinyl alcohol (PVA) and phenylboronic acid (PBA) collectively generate the necessary linker.112 This reaction is crucial for the creation of hydrogels and their stability. In converting carbohydrates, the gel structure dissolves, making it particularly effective for releasing the loaded therapeutic proteins at the targeted location. Glucose-responsive hydrogels are one of the advanced stimuli-responsive concepts in hydrogels with diverse applications.113Fig. 5 gives a broad outline of the structural changes and the different stimuli types to induce the stimuli-responsiveness of a hydrogel.
3.8. Pressure responsive hydrogels (PRH)
The capacity of biomaterials to sense the pressure applied to them and display different reactions at different pressures is a newer version.114 This behaviour can be observed in super-elastic, cellular structured, and nanofibrous hydrogels, such as those made from alginate and bendable silicon dioxide (SiO2) nanostructures. Due to the apparent higher moisture content, hydrogels designed for high water content lack substantial mechanical properties and substantially reversible deformation.114 Peptides, nanoparticles, polysaccharides, conducting polymers, and other polymeric components are assembled into 3-dimensional networks to create these biomaterials. They tend to lose mechanical properties in high water-containing conditions while having a uniform dispersion of networks.115 In this circumstance, cell fibrous network biomaterials can quickly improve the mechanical and physical properties and show considerable responsiveness to load.114 The compaction and expansion are visible when an external force is applied to hydrogels possessing vast mono-domains. A physical shear approach was used to synthesise half-ester nanocrystallised cellulose. Ultraviolet gelation of a monodomain asymmetrical gel transformed by shearing is an example. While pressed across the bridge, this hydrogel exhibits quick and reversible colour changes and expansion which reflect the extent of orientation.10
3.9. Antigen-responsive hydrogels
Foreign body (antigen) responsive hydrogels change in volume or weight in immunological response or complement. The mechanical trapping of the antigens in the gel network is modified, and chemical coupling of the antigens and the resistant cell pair as a changeable crosslinker is116 commonly used. They have wide applications as biosensors. It is feasible to create an antigen-responsive architecture using supramolecular hydrogels. In a study, the sustainability of supramolecular hydrogel capsules in a cell culture medium enables it to be upgraded to an enzymatic and cell-sensitive hydrogel with an acceptable methodology for releasing luminous medicines driven by prostate-specific foreign bodies subjected to proteolytic cleavage.117 The hydrogel was created by combining polymeric segments with linker genes, aptamers or protease enzymes. The liberation of the preloaded platinum NPs, which have strong stability and high catalytic activity for decomposing hydrogen peroxide to oxygen, is enabled by the polymer sensitivity to the target material. The created molecular oxygen subsequently causes a significant pressure increase in a confined setting which can be quickly measured with a pressure gauge.118Fig. 6 illustrates the antigen sensitive hydrogels and their composition and morphological changes upon encountering the environmental stimulation by an antigen. The structural changes and behaviour of antigen-responsive hydrogels are shown in Fig. 6.
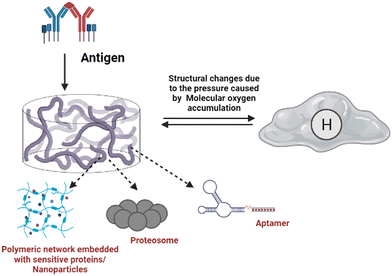 |
| Fig. 6 Antigen-sensitive hydrogels and their functional composition. | |
3.10. Enzyme-responsive hydrogels
Enzymes are employed as a cue to produce biomimetic reactions in intelligent materials because enzymes are responsible for all significant changes in biological cells.119 This includes biopolymers that change structurally and respond to the environment when exposed to certain enzymes. Enzymes are crucial in stimulating and maintaining cell and molecular microenvironment activities. The concentration of enzymes and their function vary based on the cellular or organ unit, and the activity of enzymes differs between pathologic and healthy situations.2,76 Cancer cells are not in equilibrium, and their enzyme concentrations are abnormally high compared to regular cells. As a result, enzyme-sensitive hydrogels tend to be intriguing because they are specific and may be triggered by certain inputs.75,76,120 A primary key in tissue regeneration was a PEG-based hydrogel sensitive to the concentration of metalloproteinases (MMPs). MMP-sensitive sequences were used as crosslinking agents in its production. This culminated in a compostable matrix under the effect of MMPs such as gelatinase and collagenase. An MMP-sensitive polymeric system with PEG-coated magnetic iron oxide nanoparticles (MIONPs) can be used for delivery of anticancer drugs. The addition of the particular polypeptide sequence (GGGPQGIWGQGK) (PQ), which is sensitive to proteolytic cleavage, enabled MMP responsiveness.74,121Fig. 7 illustrates the mechanism of an MMP-sensitive hydrogel in cancer drug delivery.
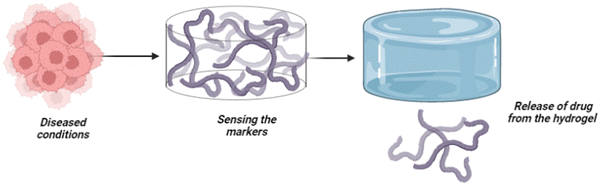 |
| Fig. 7 Mechanism of action of a disease-specific and specific marker/sequence sensitive hydrogel in the drug delivery system to the target site. | |
Enzymes and enzyme-catalysed processes have proven to be possibly activating factors in the generation of new sensitive materials.122 Enzymes are naturally occurring biological molecules that function as stimuli. Because enzymes are macromolecules, managing physical properties and harmonising the material response allows them to be released wherever required.123 Because the biochemical process requires adaptability and rearrangement, enzyme-sensitive hydrogels with low molecular weight gelators (LMWG) are new advances in this sector.124
4. Current trends and biomedical applications of intelligent hydrogels
Tissue engineering is a branch that aims to maintain the proper functioning of organs and tissues by combining engineering and biomedicine principles.125 For instance, hydrogels are intelligent biomaterials that are frequently utilised in medical services and bioengineering because of their tissue-like qualities, ability to create scaffolds for various tissues, and unique capability to fine-tune physicomechanical properties to fulfil the requirements of a specific application.126 Hydrogels are most often used in bones, connective tissues, and the vascular system. Tissues, cartilage, tendons, the epidermis, eyes, and soft tissues are all soft tissues. Hydrogels have been employed as scaffolds for several different applications.127
4.1. Intelligent hydrogels in tissue engineering and regenerative medicine applications
Owing to its integrated use of biological and physiological traits of the hydrogel, including biocompatibility and bioinspired nature, on-site pass capabilities, expanding mechanical properties, bulging efficiency, and degenerative characteristics for epidermal biomaterial and skin rejuvenation. Hydrogel substrates act as matrices/scaffolds for cells, allowing them to grow and multiply while repairing injured cells.105 Cell attachment and motility are aided by physiologically active constituents.128 Due to the general challenges associated with using auto- and allograft implants, where the donor site experiences severe discomfort, frequent infections, and scarring over time, the implementation of hydrogels in tissue engineering applications has been employed. Wound healing has significantly benefited from tissue-engineered skin substitutes.129,130
4.2. Intelligent hydrogel applications in the delivery of small molecules and biological molecules
In general, hydrogel drug delivery systems can be therapeutically beneficial. They can regulate the discharge of numerous medicinal substances spatially and temporally.131 To have therapeutic potential, traditional drug use frequently necessitates large doses or several dosages and has the drawbacks of adverse effects and toxicity concerns. Because hydrogels can be designed for chemical, structural, and biodegradation applications, polymers can be used as substrates for diverse physicochemical interactions with the encapsulated pharmaceuticals to regulate their delivery.132 These drug delivery systems can manipulate how medications are delivered to tissues and cell spanning time and space which are the most desirable features. They have been employed in various medical fields like cardiac, cancer, immunotherapy, and pain control.133 The possibility of drug decomposition and clumping when exposed to chemicals is reduced since they are generated in aqueous systems. The crosslinked matrix of these polymer systems’ polymeric network obstructs peptide entry and is thought to preserve active pharmaceuticals from early breakdown. Drug delivery systems relying on hydrogels allow the regulated release of the drug, and their influence would only increase in the future, making them a suitable framework for local delivery of drugs.13,74,134
Fibrocartilage is an avascular tissue that lines the joint's muscle mass portions. It is made up of chondrocytes, which are a special type of cartilage connective tissue cell.97 They are the isolated cells in the healthy meniscus and are involved in extracellular matrix (ECM) biosynthesis and renewal.135 The potential of the articular cartilage to self-heal is reduced due to a shortage of blood circulation, and restoring meniscus abnormalities is a serious difficulty. Regenerative medicine is a treatment option for osteochondral abnormalities and arthritis.135 In articular cartilage regeneration, the synergistic and symbiotic activity of mesenchymal stem cells (MSCs) and three-dimensional scaffolds is critical. Nevertheless, this method has drawbacks, including the weak mechanical properties of the manufactured cartilage.136,137 To promote collagen regeneration, articular cartilage repair necessitates the introduction of bioactive growth factors and the engagement of mesenchymal stem cells and biomaterials. Several constraints limit immediate supply, including quick deterioration and the necessity for higher dosages than those of an equal substance existing in the system. Immune and inflammatory responses, and the potential to spread to non-target locations can compromise their treatment efficacy.138Fig. 8 illustrates the applications of intelligent hydrogels and their enhanced properties for treatment strategies.
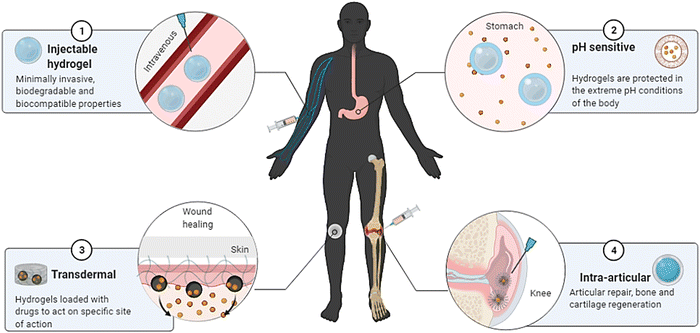 |
| Fig. 8 Applications of intelligent hydrogels in wound healing, and oral and intravenous drug delivery applications to administer therapeutic agents, and the injectability of the intelligent hydrogels for targeted treatment strategies. | |
A potential strategy to regulate the delivery of active biomolecules is synergistic coupling of biochemical factor-controlled delivery with other new technologies such as nanotechnology, physical activation, and different sophisticated approaches.14,139,140 The tissue repair microenvironment comprises various signals produced by the living process in the inflammatory process. This principle should be carefully addressed while building 3D frameworks for regeneration research. The necessity for tissue healing to use controlled delivery mechanisms appears to enable a regulated source of active substances to particular target areas, overcoming the transport challenges.131 A potential strategy for controlling the distribution of active biomolecules is the synergistic coupling of biochemical factor-controlled delivery with other new technologies such as nanotechnology, physical activation, and sophisticated approaches. The tissue repair microenvironment comprises a variety of signals produced by the living process in the inflammatory process.141,142 Hydrogels appear to be promising platforms for molecular imprinting and the production of bioactive matrices. Natural polymer-based hydrogels appear to provide good results. 3D printing techniques are a hot topic in research because of their capacity to supply and deposit biomaterials more efficiently, which is crucial for tissue regeneration.136
4.3. Intelligent hydrogel applications in three-dimensional cell culture
Hydrogel matrices can manage cellular functions and activity, a fundamental concept in tissue regeneration. Also, they possess highly hydrophilic gel networks and resemblance to biological tissues. Hydrogel constructs have been employed as transporters of cell components for homogeneous cellular loading in 3D cell culture.143 In a complex microenvironment, cells in tissues are surrounded three-dimensionally by the extracellular matrix (ECM). As a result, the biomaterials utilised to transport cells should resemble the milieu present in natural tissues.17,144 Synthetic hydrogel systems are biocompatible, biodegradable, and capable of simulating the milieu of genuine tissues, making them ideal for advanced applications like tissue engineering.145 These constructs will serve as a navigator and a matrix for cells to adhere to, migrate, and increase. The biomaterials also allow cells to self-assemble by providing structural stability. This technology can be used in molecular genetics, disease diagnosis, high-throughput screening, and bioengineering, among other fields. Hydrogel systems show promise in three-dimensional cell culture and could be used in various therapeutic applications.146
5. Stimuli-responsive hydrogel characteristics and applications
In thermo-responsive hydrogels, the tunable properties of the hydrogels are altered by temperature changes (fast polymerisation/gelation), printability with excellent shape integrity, high definition, cell adaptability with the ability to replicate the micro-environment, natural shape, and extravasation; thermoresponsive hydrogels have become suitable candidates for sophisticated bioprinting applications.146 The polymeric substances derived from biological origins include collagen, agarose, gelatin, and their derivatives. Moreover, the synthetic results include Pluronic and its products, poly(ethylene glycol oxide) (PEO) derived block polymers, and poly(N-isopropyl acrylamide) [poly (N-PNIPAAm)] and its variants, which can all be utilised to prepare responsive hydrogels for bioprinting applications.11,88 The heat dissipation of polyacrylamide (PAAm) hydrogel nanocomposites has been improved by PAAm hydrogel composites incorporating silica nanoparticles (NPs).13,43 Cancer treatment employs a wide range of strategies; embolisation is one of the tumour therapy techniques.147 The therapy includes starvation by shutting the lumen of a blood artery and supplying blood to the tumour. Researchers have developed a thermoresponsive polymeric system based on PNIPAAm which can be employed as an embolisation factor in hepatocellular carcinoma (HCC) therapy by transcatheter artery embolisation (TAE).148 In a study, researchers have applied cellulose nanowhiskers grafted with PNIPAAm polymers from Acetobacter xylinum cellulose. The developed system possessed good mechanical characteristics and an LCST close to the human body.69,148,149
Because of their improved conductance elasticity, electroresponsive hydrogels are used in soft devices. Elastic strain sensors benefit from these features, which are helpful in soft robotics and human–machine contacts.150 Microorganism diagnosis, blood glucose concentration, virus detection, therapeutic delivery, and surveillance systems are all being worked on with biosensors and bioelectronics-based hydrogels.151 A pH-stimulated reversible compression function is used in pH-sensitive hydrogels to distribute therapeutic medicines protectively.152 The capacity to provide medications specific to protein and peptide delivery orally while protecting them via the gastrointestinal tract is a significant advantage of pH-sensitive hydrogels.109,153 In addition, using a pressure-responsive hydrogel (PRH), a SiO2 nanofiber hydrogel was created using the sol–gel electrospinning approach.58 The applications of PRH are in flexible pressure sensors, tissue-engineered scaffolds within the spectrum of pressure stimuli, drug carriers, artificial organs, and so on, which can benefit from the pressure-sensitive properties of biomaterials.115,154,155Fig. 9 gives a broad spectrum of biomedical applications of intelligent hydrogels.
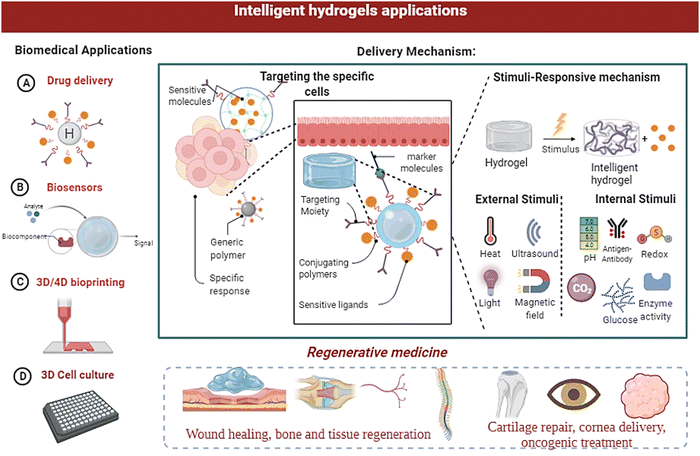 |
| Fig. 9 Biomedical applications of smart/intelligent hydrogels, as well as the structural changes in the hydrogels and their applications in diagnosis and therapeutic delivery. | |
Intelligent/smart biomaterials enable drug delivery systems (DDS) to respond to physiological factors commonly related to various diseases and dosage methods, environmental stimuli like pH, redox, and enzyme activity, and exogenous stimuli such as ultrasound or temperature.98,154,156 With the development of precision medicine, theranostics, and nucleotide-based therapies, DDS built with smart materials are gaining popularity.157 A variety of biomaterials, such as polymers, lipids, proteins, and peptides, are being used to engineer drug delivery systems (DDS) at various length scales, ranging from the nanoscale (1 nm) to the macroscale (>1 cm), for a variety of applications, e.g. enteral, parenteral and topical uses.153,158 The varying reactions of chemical components to diverse stimuli facilitate a drug delivery system.159
The development of immune-interactive smart materials has accelerated rapidly, providing a better knowledge of the immune response mechanism.160 In the realm of biomaterials with intrinsic immunogenicity and the variety in structure, dimension, and chemistry of these materials, significant advances have been made, which can be utilised to increase responses to vaccinations and other immunotherapies.12 Various biomaterials, such as polymers and lipids modified with photosensitive, pH-sensitive, or ion-sensitive materials, have aided in delivering bioactive payloads to immune cells.161,162 Smart biomaterials are also engineered to modulate the host immune system to avoid negative responses.161 In severe cases, the graft is rejected by producing inflammation and aggregation of collagen fibres over the implants causing inflammation by lowering the efficacy of the implants.163 Vast knowledge of innate immunogenicity will help researchers figure out how to control and re-program immunity mechanisms, which is crucial for creating next-generation vaccines and immunotherapeutic materials.164 In addition, the contact of antigen-presenting cells (APCs) with diverse particle morphologies is influenced by particulate geometries.165 The ability of the immune system to modify its effector activities is a determining factor of its effector functions.166 Therefore, a need for sophisticated patterns with a better understanding of the immune adaptability of the host exists.
Further, an antigen-responsive hydrogel using the dextran base with fluorescein isothiocyanate (FITC) antigens and a sheep anti-FITC IgG antibody was developed.17 This gave rise to hydrogel-based intelligent membranes with bio-specific interactions between IgG and FITC, ensuring enhanced architectural stabilisation. The formation of a polymeric gel from the copolymerisation of IgG2a and poly(N-isopropyl acrylamide) (N-PNIPAAm) by redox initiation and chemical crosslinking via N,N′-methylenebisacrylamide (MBAAm) is demonstrated in a recent work. This antigen responsive hydrogel was used in prostate cancer detection.117 A target-responsive hydrogel can be produced using a similar change in crosslinking density in reaction to targeted molecules. This breakthrough hydrogel shows good promise for quantifiable point-of-care testing that can detect cocaine, ochratoxin A, and lead ions with greater precision and specificity.118,140,167,168
In a study conducted by Sai Sai Yan et al., AD-Cu-DOX-HA, a straightforwardly built acidic tumour microenvironment-activated organosilica nanosystem, made up of an aggregation-induced emission (AIE)-active sensitiser to light, metal Cu+-engineered amino silica, the direct coordination polymer of doxorubicin (DOX), and the targeting component hyaluronic acid (HA) was prepared. In addition to accurately distinguishing between cancer and normal cells, AD-Cu-DOX-HA exhibits selective accumulation, copper ion-mediated rapid disassembly, and turn-on fluorescence in tumour tissues. This allows for effective tumour diagnosis and growth inhibition through copper ion-mediated chemodynamic therapy, DOX-enabled chemotherapy, and fluorescence imaging-navigated synergistic photodynamic therapy.169
6. Conclusion and future prospects
This review summarises intelligent hydrogels, preparation of various stimuli-responsive hydrogels, and their biomedical applications—the ability to regain shape and mechanical properties after destruction, stretchability, and ease of injection. Also, this review gives a broad perspective on hydrogels and the strategies applied to induce stimuli-responsiveness in the hydrogels with sensitivity to environmental cues which makes them “smart/intelligent hydrogels”. We also discussed the physical, chemical, and biological means to alter the design parameters of hydrogels to induce their “stimuli-responsive” behaviour to external stimuli like temperature, pH, redox, pressure, glucose concentrations, and electromagnetic fields. Further, the mechanism of crosslinking polymers with sensitive moieties and different types of materials is explained. Further, the applications of intelligent hydrogels in wound healing, drug delivery, and three-dimensional cell culture, and also the applications of stimuli-responsive hydrogels are explained.
Future perspectives and the growing technological insights give rise to broader applications of intelligent hydrogels in bioprinting modalities for various biomedical applications. Sequential advances in microcirculation and immune regulation have improved optimal framework architecture and physiological activities of scaffolds/biomaterials. Gene-editing tools like the CRISPR–Cas9 technique are used to tailor the hydrogel design to introduce specific biological cues through the RNA input mechanism in the hydrogel. Due to their biodegradable and biocompatible qualities, hydrogels are preferable to other biomaterials. In addition, the revolution in biomaterials is leading to intelligent, sustainable 3D bioprinted scaffolds with shape morphing properties, and the stimuli-responsiveness of bioengineered scaffolds will be the future scope of biomaterials research.
Conflicts of interest
The authors declare no conflicts of interest.
References
- S. Amukarimi, S. Ramakrishna and M. Mozafari, Curr. Opin. Biomed. Eng., 2021, 19, 100311 CrossRef.
- L. Jingcheng, V. S. Reddy, W. A. D. M. Jayathilaka, A. Chinnappan, S. Ramakrishna and R. Ghosh, Polymers, 2021, 13(9), 1427 CrossRef CAS PubMed.
- K. Liu, M. Tebyetekerwa, D. Ji and S. Ramakrishna, Matter, 2020, 3, 590–593 CrossRef.
- S. Tian, W. Yang, J. M. Le Grange, P. Wang, W. Huang and Z. Ye, J. Glob. Health, 2019, 3, 62–65 CrossRef.
- E. Pishavar, F. Khosravi, M. Naserifar, E. R. Ghomi, H. Luo, B. Zavan, A. Seifalian and S. Ramakrishna, Polymers, 2021, 13(16), 2680 CrossRef CAS PubMed.
- K. Joyce, G. T. Fabra, Y. Bozkurt and A. Pandit, Signal Transduction Targeted Ther., 2021, 6, 1–28 CrossRef PubMed.
- E. Rezvani Ghomi, N. Nourbakhsh, M. Akbari Kenari, M. Zare and S. Ramakrishna, J. Biomed. Mater. Res., Part B, 2021, 109, 1986–1999 CrossRef CAS PubMed.
- K. E. Papathanasiou, P. Turhanen, S. I. Brückner, E. Brunner and K. D. Demadis, Sci. Rep., 2017, 7, 2–10 CrossRef PubMed.
- K. Han, J. Nam, J. Xu, X. Sun, X. Huang, O. Animasahun, A. Achreja, J. H. Jeon, B. Pursley, N. Kamada, G. Y. Chen, D. Nagrath and J. J. Moon, Nat. Biomed. Eng., 2021, 5, 1377–1388 CrossRef CAS PubMed.
- S. Mantha, S. Pillai, P. Khayambashi, A. Upadhyay and Y. Zhang, Materials, 2019, 12, 33 CrossRef PubMed.
- F. E. Montero, R. A. Rezende, J. V. L. da Silva and M. A. Sabino, Front. Mech. Eng., 2019, 5, 1–12 CrossRef.
- R. Cui, Q. Wu, J. Wang, X. Zheng, R. Ou, Y. Xu, S. Qu and D. Li, Front. Bioeng. Biotechnol., 2021, 9, 1–11 Search PubMed.
-
C. T. Schwall and I. A. Banerjee, Micro- and nanoscale hydrogel systems for drug delivery and tissue engineering, 2009, vol. 2 Search PubMed.
-
W. Wang, R. Narain and H. Zeng, Hydrogels, Elsevier Inc., 2020, ch. 10 Search PubMed.
- J. Grenier, H. Duval, F. Barou, P. Lv, B. David and D. Letourneur, Acta Biomater., 2019, 94, 195–203 CrossRef CAS.
- Y. Zhang and Y. Huang, Front. Chem., 2021, 8, 1–13 CrossRef.
- F. Pati, J. Jang, D. H. Ha, S. Won Kim, J. W. Rhie, J. H. Shim, D. H. Kim and D. W. Cho, Nat. Commun., 2021 DOI:10.1038/ncomms4935.
- M. A. English, L. R. Soenksen, R. V. Gayet, H. De Puig, N. M. Angenent-mari, A. S. Mao, P. Q. Nguyen and J. J. Collins, Science, 2019, 785, 780–785 CrossRef PubMed.
- P. S. Kowalski, C. Bhattacharya, S. Afewerki and R. Langer, ACS Biomater. Sci. Eng., 2018, 4, 3809–3817 CrossRef CAS PubMed.
- Y. Loo, A. Lakshmanan, M. Ni, L. L. Toh, S. Wang and C. A. E. Hauser, Nano Lett., 2015, 15, 6919–6925 CrossRef CAS.
- D. Tsiapalis, A. De Pieri, M. Biggs, A. Pandit and D. I. Zeugolis, ACS Biomater. Sci. Eng., 2017, 3, 1172–1174 CrossRef CAS PubMed.
- N. A. Peppas, P. Bures, W. Leobandung and H. Ichikawa, Eur. J. Pharm. Biopharm., 2000, 50, 27–46 CrossRef CAS PubMed.
- M. Mobaraki, M. Ghaffari, A. Yazdanpanah, Y. Luo and D. K. Mills, Bioprinting, 2020, 18, e00080 CrossRef.
- J. Yin, M. Yan, Y. Wang, J. Fu and H. Suo, ACS Appl. Mater. Interfaces, 2018, 10, 6849–6857 CrossRef CAS PubMed.
- R. Subrahmanyam, P. Gurikov, I. Meissner and I. Smirnova, J. Visualized Exp., 2016 DOI:10.3791/54116.
- J. Berger, M. Reist, J. M. Mayer, O. Felt, N. A. Peppas and R. Gurny, Eur. J. Pharm. Biopharm., 2004, 57, 19–34 CrossRef CAS PubMed.
- L. Ghasemi-Mobarakeh, D. Kolahreez, S. Ramakrishna and D. Williams, Curr. Opin. Biomed. Eng., 2019, 10, 45–50 CrossRef.
- N. Anandakrishnan, H. Ye, Z. Guo, Z. Chen, K. I. Mentkowski, J. K. Lang, N. Rajabian, S. T. Andreadis, Z. Ma, J. A. Spernyak, J. F. Lovell, D. Wang, J. Xia, C. Zhou and R. Zhao, Adv. Healthcare Mater., 2021, 10, 1–12 Search PubMed.
-
Y. Dong and W. Wang, In situ-formed bioactive hydrogels for delivery of stem cells and biomolecules for wound healing, Elsevier Ltd, 2016, vol. 2 Search PubMed.
- P. Fratzl, J. R. Soc., Interface, 2007, 4, 637–642 CrossRef CAS PubMed.
- H. K. Raut, R. Das, Z. Liu, X. Liu and S. Ramakrishna, Biotechnol. J., 2020 DOI:10.1002/biot.202000160.
- G. R. López-Marcial, A. Y. Zeng, C. Osuna, J. Dennis, J. M. García and G. D. O’Connell, ACS Biomater. Sci. Eng., 2018, 4, 3610–3616 CrossRef PubMed.
- J. H. Shim, J. Y. Kim, M. Park, J. Park and D. W. Cho, Biofabrication, 2014 DOI:10.1088/1758-5082/3/3/034102.
- D. Wu, Y. Yu, J. Tan, L. Huang, B. Luo, L. Lu and C. Zhou, Mater. Des., 2018, 160, 486–495 CrossRef CAS.
- W. B. Liechty, D. R. Kryscio, B. V. Slaughter and N. A. Peppas, Annu. Rev. Chem. Biomol. Eng., 2010, 1, 149–173 CrossRef CAS PubMed.
-
C. Yong, W. Bin and C. Shi, 2007, 23, 438–446 CrossRef PubMed; R. Colom, S. Karama, R. E. Jung and R. J. Haier, Dialogues Clin. Neurosci., 2010, 12, 489–501 CrossRef PubMed.
- N. A. Peppas, J. Z. Hilt, A. Khademhosseini and R. Langer, Adv. Mater., 2006, 18, 1345–1360 CrossRef CAS.
- M. Caldorera-Moore and N. A. Peppas, Adv. Drug Delivery Rev., 2009, 61, 1391–1401 CrossRef CAS PubMed.
- S. Liang, X. Qiu, J. Yuan, W. Huang, X. Du and L. Zhang, ACS Appl. Mater. Interfaces, 2018, 10, 19123–19132 CrossRef CAS PubMed.
- J. Tabor, K. Chatterjee and T. K. Ghosh, Adv. Mater. Technol., 2020, 5, 1–40 Search PubMed.
- L. W. Xia, R. Xie, X. J. Ju, W. Wang, Q. Chen and L. Y. Chu, Nat. Commun., 2013, 4, 1–11 Search PubMed.
- Y. Işıkver and D. Saraydın, Gels, 2022 DOI:10.3390/gels7030113.
- Z. Liu, Y. Faraj, X. J. Ju, W. Wang, R. Xie and L. Y. Chu, J. Polym. Sci., Part B: Polym. Phys., 2018, 56, 1306–1313 CrossRef CAS.
- D. Zhang, Y. Liu, Y. Liu, Y. Peng, Y. Tang, L. Xiong, X. Gong and J. Zheng, Adv. Mater., 2021, 33, 1–13 Search PubMed.
- X. Zhang, X. Guo, Y. Wu and J. Gao, Gels, 2022 DOI:10.3390/gels7040224.
- Z. Chen, F. Liu, Y. Chen, J. Liu, X. Wang, A. T. Chen, G. Deng, H. Zhang, J. Liu, Z. Hong and J. Zhou, Adv. Funct. Mater., 2017, 27, 1–9 Search PubMed.
- L. Tang, Nat. Methods, 2019, 16, 955 CrossRef CAS PubMed.
- G. F. Cerofolini, AIP Adv., 2012 DOI:10.1063/1.3699046.
- C. A. Lino, J. C. Harper, J. P. Carney and J. A. Timlin, Drug Delivery, 2018, 25, 1234–1257 CrossRef CAS PubMed.
- Y. K. Sung, D. R. Lee and D. J. Chung, Biomater. Res., 2021, 25, 1–10 CrossRef.
- K. A. Smith, M. Dang, A. E. G. Baker, T. Fuehrmann, A. Fokina and M. S. Shoichet, Biomacromolecules, 2021, 22, 5118–5127 CrossRef CAS PubMed.
- S. L. Bellis, Biomaterials, 2011, 32, 4205–4210 CrossRef CAS PubMed.
- S. Yamasaki, Bone, 2014, 23, 1–7 Search PubMed.
- J. Xu, Y. Liu and S. Hui Hsu, Molecules, 2019, 24, 1–21 Search PubMed.
- X. Zhai, C. Ruan, J. Shen, C. Zheng, X. Zhao, H. Pan and W. W. Lu, J. Mater. Chem. B, 2021, 9, 2394–2406 RSC.
- X. Huang, J. Li, J. Luo, Q. Gao, A. Mao and J. Li, Mater. Today Commun., 2021, 29, 102757 CrossRef CAS.
- T. Zhang, H. Zhang, W. Zhou, K. Jiang, C. Liu, R. Wang, Y. Zhou, Z. Zhang, Q. Mei, W.-F. Dong, M. Sun and H. Li, Front. Bioeng. Biotechnol., 2022, 9, 1–9 Search PubMed.
- Z. Zhao, C. Vizetto-Duarte, Z. K. Moay, M. I. Setyawati, M. Rakshit, M. H. Kathawala and K. W. Ng, Front. Bioeng. Biotechnol., 2020, 8, 1–22 Search PubMed.
- F. A. Ran, P. D. Hsu, J. Wright, V. Agarwala, D. A. Scott and F. Zhang, Nat. Protoc., 2013, 8, 2281–2308 CrossRef CAS PubMed.
- R. Carpa, A. Remizovschi, C. A. Culda and A. L. Butiuc-Keul, Gels, 2022, 8, 70 CrossRef CAS PubMed.
- S. Bashir, M. Hina, J. Iqbal, A. H. Rajpar, M. A. Mujtaba, N. A. Alghamdi, S. Wageh, K. Ramesh and S. Ramesh, Polymers, 2020, 12, 1–60 Search PubMed.
- S. J. Buwalda, B. Nottelet and J. Coudane, Polym. Degrad. Stab., 2017, 137, 173–183 CrossRef CAS.
- F. Lv, L. Mao and T. Liu, Mater. Sci. Eng. Carbon, 2014, 43, 221–230 CrossRef CAS PubMed.
- H. Mao, G. Shan, Y. Bao, Z. L. Wu and P. Pan, Soft Matter, 2016, 12, 4628–4637 RSC.
- X. H. Dai, H. Jin, M. H. Cai, H. Wang, Z. P. Zhou, J. M. Pan, X. H. Wang, Y. S. Yan, D. M. Liu and L. Sun, React. Funct. Polym., 2015, 89, 9–17 CrossRef CAS.
- H. Mao, P. Pan, G. Shan and Y. Bao, J. Phys. Chem. B, 2015, 119, 6471–6480 CrossRef CAS PubMed.
- S. Z. Fu, P. Y. Ni, B. Y. Wang, B. Y. Chu, L. Zheng, F. Luo, J. C. Luo and Z. Y. Qian, Biomaterials, 2012, 33, 4801–4809 CrossRef CAS PubMed.
- X. Li, W. Wu and W. Liu, Carbohydr. Polym., 2008, 71, 394–402 CrossRef CAS.
- J. Zhang, Z. Cui, R. Field, M. G. Moloney, S. Rimmer and H. Ye, Eur. Polym. J., 2015, 67, 346–364 CrossRef CAS.
- B. Shriky, A. Kelly, M. Isreb, M. Babenko, N. Mahmoudi, S. Rogers, O. Shebanova, T. Snow and T. Gough, J. Colloid Interface Sci., 2020, 565, 119–130 CrossRef CAS PubMed.
- G. Lin, L. Cosimbescu, N. J. Karin and B. J. Tarasevich, Biomed. Mater., 2012 DOI:10.1088/1748-6041/7/2/024107.
- G. E. Cinay, P. Erkoc, M. Alipour, Y. Hashimoto, Y. Sasaki, K. Akiyoshi and S. Kizilel, ACS Biomater. Sci. Eng., 2017, 3, 370–380 CrossRef CAS PubMed.
- X. Zhao, Y. Liang, Y. Huang, J. He, Y. Han and B. Guo, Adv. Funct. Mater., 2020, 1910748, DOI:10.1002/ADFM.201910748.
- A. Kasiński, M. Zielińska-Pisklak, E. Oledzka and M. Sobczak, Int. J. Nanomed., 2020, 15, 4541–4572 CrossRef PubMed.
- A. S. Puranik, L. P. Pao, V. M. White and N. A. Peppas, Eur. J. Pharm. Biopharm., 2016, 108, 196–213 CrossRef CAS PubMed.
- A. P. Mathew, K. H. Cho, S. Uthaman, C. S. Cho and I. K. Park, Polymers, 2017, 9(4), 152, DOI:10.3390/POLYM9040152.
- X. J. Ju, R. Xie, L. Yang and L. Y. Chu, Expert Opin. Ther. Pat., 2009, 19, 683–696 CrossRef CAS PubMed.
- S. Chatterjee, P. C. L. Hui and C. Wai Kan, Polymers, 2018, 10(5), 480, DOI:10.3390/POLYM10050480.
- C. Ran, J. Wang, Y. He, Q. Ren, H. Hu, J. Zhu, X. Gu, M. Li, L. Zheng and J. Li, Macromol. Biosci., 2022, 2100474 CrossRef CAS.
- K. Zhang, K. Xue and X. J. Loh, Gels, 2021, 7, 1–17 Search PubMed.
- L. Klouda and A. G. Mikos, Eur. J. Pharm. Biopharm., 2011, 68, 34–45 CrossRef PubMed.
- W. Liang, S. Bhatia, F. Reisbeck, Y. Zhong, A. K. Singh, W. Li and R. Haag, Adv. Funct. Mater., 2021, 31, 2010630, DOI:10.1002/adfm.202010630.
- H. Huang, X. Qi, Y. Chen and Z. Wu, Saudi Pharm. J., 2019, 27, 990–999 CrossRef CAS PubMed.
- M. Sponchioni, U. Capasso Palmiero and D. Moscatelli, Mater. Sci. Eng. Carbon, 2019, 102, 589–605 CrossRef CAS PubMed.
- G. Davidson-Rozenfeld, L. Stricker, J. Simke, M. Fadeev, M. Vázquez-González, B. J. Ravoo and I. Willner, Polym. Chem., 2019, 10, 4106–4115 RSC.
- B. Jiang, X. Liu, C. Yang, Z. Yang, J. Luo, S. Kou, K. Liu and F. Sun, Sci. Adv., 2020, 6, 1–10 Search PubMed.
- C. D. Abueva, P.-S. Chung, H.-S. Ryu, S.-Y. Park and S. H. Woo, Med. Lasers, 2020, 9, 6–11 CrossRef.
- D. Han, Z. Lu, S. A. Chester and H. Lee, Sci. Rep., 2018, 8, 1–10 Search PubMed.
-
R. Yu, H. Zhang and B. Guo, Conductive Biomaterials as Bioactive Wound Dressing for Wound Healing and Skin Tissue Engineering, Springer, Singapore, 2022, vol. 14 Search PubMed.
- S. Talebian, M. Mehrali, R. Raad, F. Safaei, J. Xi, Z. Liu and J. Foroughi, Front. Chem., 2020, 8, 1–11 CrossRef.
- L. Ghasemi-Mobarakeh, M. P. Prabhakaran, M. Morshed, M. H. Nasr-Esfahani, H. Baharvand, S. Kiani, S. S. Al-Deyab and S. Ramakrishna, J. Tissue Eng. Regener. Med., 2011, 17–35 CrossRef PubMed.
- J. H. Min, M. Patel and W. G. Koh, Polymers, 2018, 10(10), 1078, DOI:10.3390/polym10101078.
- J. H. Min, M. Patel and W. G. Koh, Polymers, 2018, 10, 1–36 Search PubMed.
- Q. Peng, J. Chen, T. Wang, X. Peng, J. Liu, X. Wang, J. Wang and H. Zeng, InfoMat, 2020, 2, 843–865 CrossRef CAS.
- S. Ganguly and S. Margel, Polymers, 2021 DOI:10.3390/polym13234259.
- I. M. El-Sherbiny and H. D. C. Smyth, J. Nanomater., 2011 DOI:10.1155/2011/910539.
- L. K. Narayanan, P. Huebner, M. B. Fisher, J. T. Spang, B. Starly and R. A. Shirwaiker, ACS Biomater. Sci. Eng., 2016, 2, 1732–1742 CrossRef CAS.
- H. J. Haroosh, Y. Dong, S. Jasim and S. Ramakrishna, J. Nanomaterials, 2022, 2022, 5190163 CrossRef.
- B. Kurt, U. Gulyuz, D. D. Demir and O. Okay, Eur. Polym. J., 2016, 81, 12–23 CrossRef CAS.
- M. K. Gupta, J. R. Martin, T. A. Werfel, T. Shen, J. M. Page and C. L. Duvall, J. Am. Chem. Soc., 2014, 136, 14896–14902 CrossRef CAS PubMed.
- A. Hendi, M. U. Hassan, M. Elsherif, B. Alqattan, S. Park, A. K. Yetisen and H. Butt, Int. J. Nanomed., 2020, 15, 3887–3901 CrossRef CAS.
- A. R. Hibbins, P. Kumar, Y. E. Choonara, P. P. D. Kondiah, T. Marimuthu, L. C. du Toit and V. Pillay, Polymers, 2017, 9(10), 474, DOI:10.3390/polym9100474.
- P. Ilgin, H. Ozay and O. Ozay, J. Polym. Res., 2020, 27, 251 CrossRef CAS.
- P. Colombo, R. Bettini, P. Santi and N. A. Peppas, Pharm. Sci. Technol. Today, 2000, 3, 198–204 CrossRef CAS PubMed.
- J. Feng, S. T. Nguyen, Z. Fan and H. M. Duong, Chem. Eng. J., 2015, 270, 168–175 CrossRef CAS.
-
W. Cheng and Y. Liu, Redox-responsive hydrogels, Elsevier Ltd, 2017 Search PubMed.
- M. Khodeir, B. Ernould, J. Brassinne, S. Ghiassinejad, H. Jia, S. Antoun, C. Friebe, U. S. Schubert, Z. Kochovski, Y. Lu, E. Van Ruymbeke and J. F. Gohy, Soft Matter, 2019, 15, 6418–6426 RSC.
- N. Vijayakameswara Rao, H. Ko, J. Lee and J. H. Park, Front. Bioeng. Biotechnol., 2020 DOI:10.3389/fbioe.2018.00110.
- P. M. Kharkar, M. S. Rehmann, K. M. Skeens, E. Maverakis and A. M. Kloxin, ACS Biomater. Sci. Eng., 2016, 2, 165–179 CrossRef CAS PubMed.
- M. Khodeir, H. Jia, A. Vlad and J. F. Gohy, Polymers, 2021, 13, 1–10 CrossRef PubMed.
- C. K. Sudhakar, Responsive hydrogel, Alloy, 2015 Search PubMed.
- C. Wang, B. Lin, H. Zhu, F. Bi, S. Xiao, L. Wang, G. Gai and L. Zhao, Molecules, 2019 DOI:10.3390/molecules24061089.
- A. Matsumoto, M. Tanaka, H. Matsumoto, K. Ochi, Y. Moro-Oka, H. Kuwata, H. Yamada, I. Shirakawa, T. Miyazawa, H. Ishii, K. Kataoka, Y. Ogawa, Y. Miyahara and T. Suganami, Sci. Adv., 2017, 3, 1–13 Search PubMed.
- M. C. Koetting, J. T. Peters, S. D. Steichen and N. A. Peppas, Mater. Sci. Eng., R, 2015, 93, 1–49 CrossRef PubMed.
- M. Li, W. Li, W. Cai, X. Zhang, Z. Wang, J. Street, W. J. Ong, Z. Xia and Q. Xu, Mater. Horiz., 2019, 6, 703–710 RSC.
- G. Liu, Y. He, P. Liu, Z. Chen, X. Chen, L. Wan, Y. Li and J. Lu, Engineering, 2020, 6, 1232–1243 CrossRef.
- M. Ikeda, R. Ochi, A. Wada and I. Hamachi, Chem. Sci., 2010, 1, 491–498 RSC.
- S. F. Souza, S. Kogikoski, E. R. Silva and W. A. Alves, J. Braz. Chem. Soc., 2017, 28, 1619–1629 CAS.
- S. Taheri, G. Bao, Z. He, S. Mohammadi, H. Ravanbakhsh, L. Lessard, J. Li and L. Mongeau, Adv. Sci., 2021, 2102627, 1–13 Search PubMed.
- V. P. Torchilin, Nat. Rev. Drug Discovery, 2014, 13, 813–827 CrossRef CAS PubMed.
- A. Sgambato, L. Cipolla and L. Russo, Gels, 2016 DOI:10.3390/GELS2040028.
- D. K. Verma, A. K. Niamah, A. R. Patel, M. Thakur, K. Singh Sandhu, M. L. Chávez-González, N. Shah and C. Noe Aguilar, Food Res. Int., 2020, 133, 109136 CrossRef CAS.
- A. Tirella, G. Mattei, M. La Marca, A. Ahluwalia and N. Tirelli, Front. Bioeng. Biotechnol., 2020, 8, 1–14 CrossRef PubMed.
- P. D. Thornton, R. Billah and N. R. Cameron, Macromol. Rapid Commun., 2013 DOI:10.1002/marc.201200649.
- N. Almouemen, H. M. Kelly and C. O’Leary, Comput. Struct. Biotechnol. J., 2019, 17, 591–598 CrossRef CAS PubMed.
- A. S. Perera and M. O. Coppens, Philos. Trans. R. Soc., A, 2019 DOI:10.1098/rsta.2018.0268.
- A. Tarafdar, V. K. Gaur, N. Rawat, P. R. Wankhade, G. K. Gaur, M. K. Awasthi, N. A. Sagar and R. Sirohi, Bioengineered, 2021, 12, 8247–8258 CrossRef CAS PubMed.
-
G. Chen and N. Kawazoe, Regulation of Stem Cell Functions by Micro-Patterned Structures, 2020, vol. 1250 Search PubMed.
- R. V. Ulijn, N. Bibi, V. Jayawarna, P. D. Thornton, S. J. Todd, R. J. Mart, A. M. Smith and J. E. Gough, Rev.: Lit. Arts Am., 2007, 10, 40–48 CAS.
- A. Sultana, H. Luo and S. Ramakrishna, Antibiotics, 2021, 10, 1–24 CrossRef.
- S. Pacelli, P. Paolicelli, M. Avitabile, G. Varani, L. Di Muzio, S. Cesa, J. Tirillò, C. Bartuli, M. Nardoni, S. Petralito, A. Adrover and M. A. Casadei, Eur. Polym. J., 2018, 104, 184–193 CrossRef CAS.
- C. R. Lynch, P. P. D. Kondiah, Y. E. Choonara, L. C. du Toit, N. Ally and V. Pillay, Front. Bioeng. Biotechnol., 2020, 8, 1–18 CrossRef.
- D. Buenger, F. Topuz and J. Groll, Prog. Polym. Sci., 2012, 37, 1678–1719 CrossRef CAS.
- S. Senapati, A. K. Mahanta, S. Kumar and P. Maiti, Signal Transduction Targeted Ther., 2018, 3, 1–19 CrossRef CAS.
- R. J. Wade and J. A. Burdick, Mater. Today, 2012, 15, 454–459 CrossRef CAS.
- X. Yang, Z. Lu, H. Wu, W. Li, L. Zheng and J. Zhao, Mater. Sci. Eng. Carbon, 2018, 83, 195–201 CrossRef CAS PubMed.
- T. Gonzalez-Fernandez, S. Rathan, C. Hobbs, P. Pitacco, F. E. Freeman, G. M. Cunniffe, N. J. Dunne, H. O. McCarthy, V. Nicolosi, F. J. O’Brien and D. J. Kelly, J. Controlled Release, 2019, 301, 13–27 CrossRef CAS PubMed.
- S. Wang, J. Li, Z. Zhou, S. Zhou and Z. Hu, Molecules, 2019, 24, 1–13 Search PubMed.
- O. C. Farokhzad and R. Langer, ACS Nano, 2009, 3, 16–20 CrossRef CAS PubMed.
- Y. Zhang, M. Li, X. Gao, Y. Chen and T. Liu, J. Hematol. Oncol., 2019, 12, 1–13 CrossRef PubMed.
-
E. J. Sheehy, G. M. Cunniffe and F. J. O’Brien, Peptides and Proteins as Biomaterials for Tissue Regeneration and Repair, 2018, pp. 127–150 Search PubMed.
- R. F. Pereira, A. Sousa, C. C. Barrias, P. J. Bártolo and P. L. Granja, Mater. Horiz., 2018, 5, 1100–1111 RSC.
- E. Frentzos, Lect. Notes Comput. Sci., 2003, 2750, 289–305 Search PubMed.
- N. Di Marzio, D. Eglin, T. Serra and L. Moroni, Front. Bioeng. Biotechnol., 2020 DOI:10.3389/fbioe.2020.00326.
-
E. Abelardo, Synthetic material bioinks, Elsevier Ltd., 2018 Search PubMed.
- S. R. Caliari and J. A. Burdick, Nat. Methods, 2016, 13, 405–414 CrossRef CAS PubMed.
- H. Tekin, J. G. Sanchez, T. Tsinman, R. Langer and A. Khademhosseini, AIChE J., 2011, 57, 3249–3258 CrossRef CAS PubMed.
- X. Chen, L. Huang, H. J. Sun, S. Z. D. Cheng, M. Zhu and G. Yang, Macromol. Rapid Commun., 2014, 35, 579–584 CrossRef CAS PubMed.
- D. Lu, J. Wang, Y. Li, Y. Zhang, L. Yu, T. Xu, H. Guo, Y. Zhang, X. Wang, X. Wang, G. Teng and Z. Lei, ACS Cent. Sci., 2020 DOI:10.1021/acscentsci.0c00506.
- Z. Ying, Q. Wang, J. Xie, B. Li, X. Lin and S. Hui, J. Mater. Chem. C, 2020, 8, 4192–4205 RSC.
- M. C. Koetting, J. T. Peters, S. D. Steichen and N. A. Peppas, Mater. Sci. Eng., R, 2015, 93, 1–49 CrossRef PubMed.
- Y. Liu and S. H. Hsu, Front. Chem., 2018, 6, 1–10 CrossRef.
- N. J. N. Norman, P. Spack Daniel and E. Shumer, Physiol. Behav., 2017, 176, 139–148 CrossRef.
- E. M. Frazar, R. A. Shah, T. D. Dziubla and J. Z. Hilt, J. Appl. Polym. Sci., 2020, 137, 1–14 CrossRef PubMed.
- X. Yu, Y. Zheng, H. Zhang, Y. Wang, X. Fan and T. Liu, Chem. Mater., 2021, 33, 6146–6157 CrossRef CAS.
- M. T. Cook, P. Haddow, S. B. Kirton and W. J. McAuley, Adv. Funct. Mater., 2021 DOI:10.1002/adfm.202008123.
- H. J. Huang, Y. L. Tsai, S. H. Lin and S. H. Hsu, J. Biomed. Sci., 2019, 26, 1–11 CrossRef CAS PubMed.
- F. Scheffold, Nat. Commun., 2020, 11, 1–13 CrossRef PubMed.
- J. Li and D. J. Mooney, Nat. Rev. Mater., 2016, 1, 1–18 Search PubMed.
- G. G. Genchi, A. Marino, C. Tapeinos and G. Ciofani, Front. Bioeng. Biotechnol., 2017, 5, 1–8 Search PubMed.
- X. Li, Y. Shou and A. Tay, Adv. NanoBiomed Res., 2021, 1, 2000073 CrossRef.
- L. Li, J. M. Scheiger and P. A. Levkin, Adv. Mater., 2019 DOI:10.1002/adma.201807333.
- E. Mariani, G. Lisignoli, R. M. Borzì and L. Pulsatelli, Int. J. Mol. Sci., 2019 DOI:10.3390/ijms20030636.
- Q. Shi, H. Liu, D. Tang, Y. Li, X. J. Li and F. Xu, NPG Asia Mater., 2019 DOI:10.1038/s41427-019-0165-3.
- C. Mora-Solano and J. H. Collier, J. Mater.
Chem. B, 2014, 2, 2409–2421 RSC.
- W. Ahmed, Z. Zhai and C. Gao, Mater. Today Bio, 2019, 2, 100017 CrossRef CAS PubMed.
- X. Min, D. Fu, J. Zhang, J. Zeng, Z. Weng, W. Chen, S. Zhang, D. Zhang, S. Ge, J. Zhang and N. Xia, Biomed. Microdevices, 2018, 20, 1–9 CrossRef CAS.
- N. Bajwa, N. K. Mehra, K. Jain and N. K. Jain, Artif. Cells, Nanomed., Biotechnol., 2016, 44, 758–768 CAS.
- S. Yan, P. Sun, N. Niu, Z. Zhang, W. Xu, S. Zhao, L. Wang, D. Wang and B. Z. Tang, ACS Nano, 2022 DOI:10.1021/acsnano.2c03550.
|
This journal is © The Royal Society of Chemistry 2022 |
Click here to see how this site uses Cookies. View our privacy policy here.