DOI:
10.1039/D0BM01852J
(Review Article)
Biomater. Sci., 2021,
9, 4246-4259
Biomimetic hydrogels designed for cartilage tissue engineering
Received
31st October 2020
, Accepted 12th February 2021
First published on 12th February 2021
Abstract
Cartilage regeneration and repair remain a clinical challenge due to the limited capability of cartilage to self-regenerate. Worldwide, the costs associated with cartilage regeneration per patient are estimated on average £30
000 for producing and supplying cells. Regenerative approaches may include the use of cell therapies and tissue engineering by combining relevant cells, scaffolds and instructive biomolecules to stimulate or modulate cartilage repair. Hydrogels have been of great interest within these fields to be used as 3D substrates to cultivate and grow cartilage cells. Currently, biomimetic hydrogels with adequate biological and physicochemical properties, such as mechanical properties, capable of supporting load-bearing capability, are yet to succeed. In this review, biomaterials’ advantages and disadvantages for the manufacturing of biomimetic hydrogels for cartilage regeneration are presented. Different studies on the formulation of cartilage-like hydrogels based on materials such as gelatin, chondroitin sulfate, hyaluronic acid and polyethylene glycol are summarised and contrasted in terms of their mechanical properties (e.g. elastic modulus) and ability to enhance cell function such as cell viability and GAG content. Current limitations and challenges of biomimetic hydrogels for cartilage regeneration are also presented.
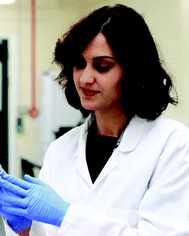 Ana M. Ferreira | Ana Ferreira-Duarte is a Lecturer in the School of Engineering at Newcastle University, UK. She obtained a degree in Chemical Engineering and M.Sc. in Biomedical Engineering from the Universidad Simon Bolivar (Republic of Venezuela), and her PhD in Biomedical Engineering from the Politecnico di Torino (Italy). She received the Award of High Qualification Research Doctorate by the Scuola Interpolitecnica di Dottorato for her dissertation. She moved to the UK in 2013 and joined Newcastle University and the Arthritis Research UK Tissue Engineering Centre. Her research focuses on biomaterial design and biointerfaces, manufacturing and functionalization of biomimetic systems for tissue engineering and regenerative medicine. |
Introduction
Articular cartilage is a non-vascularised and low cellular density connective tissue in the joints. It is frequently damaged due to injury or normal wear and tear.1 Articular cartilage resists compressive forces and protects the underlying subchondral bone. It also provides a low friction surface for a diarthrodial joint to pivot. One of the most common joint diseases affecting articular cartilage is for example osteoarthritis, which affects approximately 300 million people worldwide and is the leading cause of disability among the elderly.2 This condition is caused by cartilage injury in the joints due to stress, especially in the weight-bearing joints. Due to its avascular nature, low cell proliferation, and migration, repairing cartilage has been one of the challenges for improving the clinical outcome of the patient.3
Articular cartilage is made up of chondrocytes contained in the cartilage lacunae, residing in an extracellular matrix (ECM) produced by the chondrocytes. Chondrocytes reside in the cartilage in small cavities known as lacunae and reside cytoplasmically isolated from the neighbouring cells. Chondrocytes are normally rounded with a high matrix to cell volume ratio. In the cartilage, chondrocytes play roles in synthesizing and maintaining the ECM to prevent tissue deformation and supporting tissue function. They also influence epiphyseal plate growth by cell proliferation, matrix secretion, and cell volume increment during hypertrophy.4
The ECM is highly hydrated and contains proteoglycans (e.g. hyaluronic acid and glycosaminoglycans), collagen fibres and elastin. Glycosaminoglycans consist of chondroitin sulphate and keratin sulfate.5 ECM is essential for cartilage as it plays a role in cell signalling and it can regulate cell adhesion, growth, differentiation and migration. The ECM also maintains the physiological homeostasis of the cell microenvironment.6 Unlike most tissues, cartilage does not have blood vessels, nerves, or lymphatics. The composition of cartilage differs between four zones (Fig. 1A), which are mostly composed of proteoglycan content and collagen fibre alignment. In the superficial zone, collagen fibres are aligned parallel to the articulating surface, while in the middle zone, they are unaligned. In the deep zone, the fibres are radially arranged, and lastly, in the zone of calcified cartilage, an interface region between cartilage and bone is formed with the presence of mineralised fibres. The composition and organisation of each zone layer are to be considered when mimicking native cartilage tissues, as they impart distinctive zone mechanical properties, e.g. the compressive modulus increases with depth from the articular surface.7
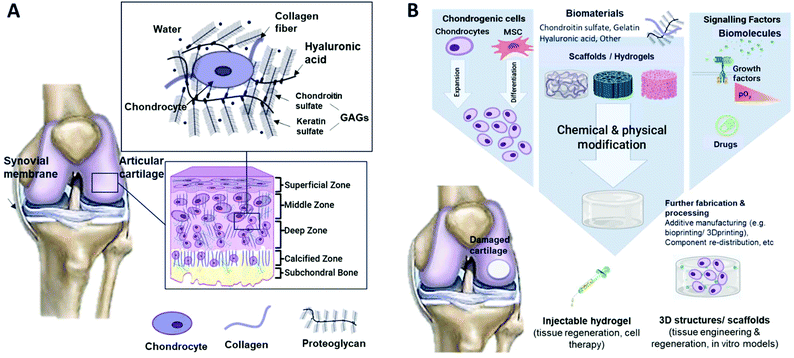 |
| Fig. 1 Schematic illustrations of (A) cartilage composition and typical tissue zones and (B) the tissue engineering approach for cartilage repair. | |
In superficial and mid-zone cartilage, chondrocytes function to produce collagen type II, IX, and XI, and proteoglycans of the ECM. Collagen type II makes up 90–95% of the collagen in the ECM and helps to stabilize the matrix, providing tensile and shear strength to the tissues.8 Collagen type IX and XI are less abundant in articular cartilage, and function to stabilize fibrillar collagen crosslinking. In the deep zone, chondrocytes are terminally differentiated and synthesize collagen type X, which plays a role in calcification.9 Type X collagen is also a hallmark for proteolytic enzyme production, which breaks down the cartilage ECM and allows for vascularization and calcification of tissues.8
Tissue engineering (TE) is an interdisciplinary field that combines materials science and cell biology to improve or replace biological tissues. The main elements that build up TE are based on cell source, scaffold, and signalling molecules (Fig. 1B). The 3D porous scaffold acts as a template for tissue formation as it provides a suitable environment for the tissues or organs to grow. For example, scaffolds used in cartilage regeneration can be differentiated into sponges, membranes, and non-woven, non-injectable and injectable hydrogels. A hydrogel is a hydrophilic polymer that is capable of holding a large amount of water.10 This property of hydrogels is crucial for mimicking the high water content (up to 80%11) present in cartilage tissue. The scaffold is seeded with cells and optional signalling factors such as growth factors or external stimuli.12 The use of scaffolds is also considered in other cartilage regenerative medicine approaches, such as in cell therapies like autologous or allogeneic chondrocyte implantation. The scaffold can be seeded with cartilage cells and placed in defective areas. So, the hydrogel is to be tuned to modulate cell behaviour such as cell migration and adhesion, providing temporary support for cellular function by polymer degradation.13
In cartilage tissue engineering, scaffolds or hydrogels with suitable properties to improve cellular function to regenerate cartilage and support load-bearing capability are yet to succeed. This review aims to give an overview of hydrogel usage for cartilage tissue regeneration, covering materials, techniques, and the future outlook of hydrogels as a tissue engineering approach.
The use of hydrogels in cartilage tissue engineering
Hydrogels are attractive scaffolds as they can be structurally similar to the ECM of various tissues, and can be delivered arthroscopically, making it a minimally invasive treatment.13 Hydrogel properties can be tuned to a particular application including drug delivery and tissue repair. The usage of hydrogels for cartilage regeneration research has been increasing in the last decade. For instance, after a keyword search on the “Web of Knowledge” using a combination of “Scaffold”, “Cartilage”, “Hydrogel” and “Regeneration” on 25th of September 2020 (shown in Fig. 2), it was found that the number of research outputs on cartilage scaffolds on average nearly triples the number of studies on cartilage hydrogels. Based on this, the number of publications on hydrogels used as cartilage scaffolds is about 64% of the overall total of cartilage scaffold articles. Studies on hydrogels for the regeneration of cartilage have been steadily increasing up to 1.4 times between 2016 and 2020, representing about 74% of the total number of studies of cartilage hydrogel outputs.
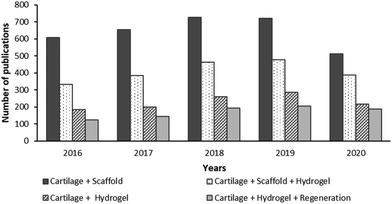 |
| Fig. 2 The topic of research from the last five years in the “Web of Knowledge” on the use of hydrogel for cartilage tissue (last accessed on 25th September 2020). | |
Hydrogels offer the advantage of being tunable, so their properties can be modified to suit the application requirements. Some of the adjustable factors are the composition of the hydrogel, degree of crosslinking and methods of crosslinking that make the structure rigid, and density of the cells in the hydrogel. Ideally, for cartilage applications, the hydrogel composition should mimic the ECM whilst being able to withstand mechanical stress and loads, especially in load-bearing joints.14 For instance, desired compressive stress between 0.4 and 2.0 MPa, tensile stress between 5 and 25 MPa, and shear stresses in the order of 3 MPa have been reported.15–17
Biomimetic hydrogels for cartilage TE
Materials used to create biomimetic hydrogels
Broadly, hydrogels can be differentiated, according to the nature of the material composition, into natural, synthetic and biosynthetic. The natural hydrogels are commonly used for cartilage regeneration purposes;50 these include protein-based, polysaccharide-based, and decellularized hydrogels. The protein hydrogel includes collagen, elastin, fibrin, gelatin, and silk fibroin, while the polysaccharide hydrogel includes, for instance, glycosaminoglycans, alginate, chitosan, and agarose.51 In addition, biodegradable synthetic materials have been explored such as poly(ethylene glycol) (PEG), poly(N-isopropylacrylamide) (pNiPAAm), and poly(vinyl alcohol) (PVA). In addition, polyesters such as polylactide acid (PLA) and polycaprolactone (PCL) and its derivatives are incorporated into hydrogels to reinforce mechanical properties or as carriers for drug delivery.52 In Table 1, some of the common materials used for tissue engineering are summarised, reflecting their strengths, weaknesses and potential applications. In the last 5 years, innovative bioinspired hydrogels for cartilage applications have been formulated including mussel-inspired polydopamine-incorporated hydrogels32,53,54 to facilitate cell adhesion and tissue integration, incorporating sugar-based additives such as manuka honey to provide antibacterial properties55 and improved mechanical properties,56 and to mimick biological structures57,58 and networks59 for improved mechanical and biological performance.
Table 1 Comparison of strengths and weaknesses of different biomaterials used in cartilage tissue engineering
Natural material |
Material |
Type |
Strengths |
Weaknesses |
Collagen |
Protein-based |
Low antigenicity, low inflammatory response, excellent biological properties, biodegradability and biocompatibility18–20 |
High cost, thrombogenic potential, low mechanical strength and modification difficulty21,22 |
Gelatin |
Protein-based |
Low cost, minimal immunogenicity, degradability and compatibility23 |
Stability decreases at high temperatures24 |
Silk fibroin |
Protein-based |
Excellent mechanical properties, low immunogenicity,25 and low thrombogenicity26 |
Source difficulty and slow gelation27 |
Glycosaminoglycans (GAGs)–hyaluronic acid (HA) and chondroitin sulphate (CS) |
Polysaccharide-based |
Mimics cartilage ECM composition, biodegradable,28 reabsorbable, easy to scale up,29 and binds signalling factors30 |
Rapid degradation in vivo and require crosslinking to be stable31,32 |
Alginate |
Polysaccharide-based |
Non-toxic, non-inflammatory and rapid gelation33,34 |
Poor cell adhesion and mechanical properties35 |
Chitosan |
Polysaccharide-based |
Anti-bacterial, low cost, biocompatible and tuned biodegradability36 |
Poor mechanical properties37 |
Decellularized hydrogels |
Protein-based |
Recreate native ECM cartilage38 and can deliver biochemical signal |
Require complex processes and potential immunogenicity38 |
Synthetic material |
Material |
Strengths |
Weaknesses |
Poly(ethylene glycol) (PEG) |
Biodegradable and non-immunogenicity39 adjustable properties40 |
Lack of adhesion support40 |
Poly(glutamic acid) (PGA) |
Biodegradable by hydrolysis, thermoplastic, adjustable mechanical properties,41 porosity42 and localized inflammation43 |
Weak physically crosslinked gel, hydrolysis product can cause inflammation and fast degradation44 |
Poly(lactic acid) and copolymers |
Biodegradable by hydrolysis,45 good mechanical properties46 and solubility in organic solvents,47 and reinforce hydrogels48,49 |
Hydrolysis by-products can cause inflammation44 |
The use of crosslinking in biomimetic hydrogels
In hydrogels, the degree of crosslinking can control the swelling properties, while increasing the stability, and controlling the degradation rates and the level of porosity.60 These are fundamental properties for tissue regeneration, since these will influence cellular function and the ability to provide support for new tissue formation. For instance, uncontrolled hydrogel swelling causes hydrostatic pressure in the surrounding tissue, which can cause inflammation and limited regeneration. The hydrogel polymer(s) chains can be crosslinked covalently or non-covalently (i.e. ionic bonds) involving either a chemical or physical reaction.61 Chemical crosslinkers are commonly used to establish covalent bonds between polymer chains, whereas physical crosslinkers facilitate physical interactions between chains or trigger chemical polymerizations. Cross-linking with free-radical polymerizations or copolymerization is commonly used by reacting hydrophilic monomers to multifunctional cross-linkers to produce cross-link junctions and physical interactions. The condensation reaction is often used to crosslink hydroxyl groups or amines with carboxylic acids present in proteins and some polysaccharides. For instance, the carbodiimide reaction may use the N,N-(3-dimethylaminopropyl)-N-ethylcarbodiimide (EDC) reagent to crosslink water-soluble polymers with amide bonds. The O-acylisourea intermediate, the carbodiimide activated acid, becomes deactivated fast, hence hindering the occurrence of amine reactions. The addition of N-hydroxysuccinimide (NHS) allows for more stable esters compared to the O-acylisourea intermediate and more reactivity towards amines.62
In situ crosslinking of injectable hydrogels allows for a minimally invasive procedure and cell encapsulation and transplantation, and enables the ability to match irregular defects.6 Different crosslinking methods can be used to prepare injectable hydrogels, including physical and chemical crosslinking63 such as photo-crosslinking, enzymatically crosslinked hydrogels, ion- or pH-sensitive hydrogels, click chemistry, etc. For instance, recent works on click-crosslinked modified HA hydrogels for cartilage tissue engineering have shown suitable mechanical properties and porous structures leading to high GAG expression and chondrogenic differentiation of human periodontal ligament stem cells (hPLSCs).64 Similarly, enzymatic crosslinking of hydrogels has drawn significant attention in the development of injectable hydrogels for tissue engineering, as it enables a quick gelation under physiological conditions with high specificity and low cytotoxicity.65 Optimal enzyme selection still needs to be achieved to maintain cell bioactivity during encapsulation.66 Some of the recent works on enzymatically crosslinked collagen-HA hydrogels showed promising results as injectable bone marrow mesenchymal stem cell (BMSC)-laden hydrogels for cartilage tissue regeneration.67 These results were observed in vitro and in vivo when implanted in rats’ cartilage defects, in which the hydrogel supported BMSC chondrogenic differentiation and hyaline cartilage repair. Similarly, studies on enzyme-crosslinked biomimetic hydrogels based on gelatin enriched with the cartilage extracellular matrix evidenced promising results in hyaline cartilage formation and GAG content in rabbit knee joint models.68
Another approach is interpenetrating network (IPN) hydrogels, in which two or more polymers in the networks are partly interlaced on the molecular scale within the matrix; these are not covalently bonded and these polymers cannot be separated without breaking their chemical bonds in the matrix.69 Multiple networks such as IPN are better than a single network in terms of mechanical strength and swelling ability.70,71 Broadly, the high water content tends to reduce the mechanical properties of single network hydrogels. Thus, novel approaches such as the “double network” (DN) have been lately developed to improve their mechanical performance. This type of IPN hydrogel consists of a preparation first of a densely cross-linked polyelectrolyte network, followed by a neutral and loosely cross-linked (fully swollen) network.72 This approach, initially promoted by Gong et al.,73 has led to promising results towards mimicking the native cartilage mechanical properties,74 including stiffness, high resistance to wear, and compression limit.75,76 The polymer DN approach has been applied as a tissue-reinforcement mechanism by interpenetrating the damaged cartilage tissue, showing an increase of the tissue's equilibrium compressive modulus and wear resistance, while maintaining the cartilage volume when subject to harsh articulation conditions.77 DN synthetic hydrogels have been used to mimic native mechanical properties and lubricity of cartilage obtaining exceptional cartilage-like properties suitable even for repairing chondral defects in the load-bearing regions of the body.76 Lastly, DN hydrogels can be also enzymatically crosslinked for repairing cartilage. This was demonstrated recently by encapsulating human articular chondrocytes into biosynthetic DN hydrogels for the 3D bioprinting of cartilage engineering constructs, obtaining highly cytocompatible hydrogels with fast kinetics when compared to compositionally similar materials.78
Mimicking cartilage composition
Cartilage is a highly hydrated tissue mostly composed of a combination of proteins and GAGs, such as collagen, HA, CS, and KS. In particular, CS and KS (keratan sulfate) are two types of glycosaminoglycans (GAGs) that are normally attached to a protein core forming proteoglycans namely aggrecan.5 This KS/CS proteoglycan is linked to the HA core protein to form a larger proteoglycan aggregate. In particular, CS and HA are two types of glycosaminoglycans (GAGs) that are normally attached to a protein chain forming the proteoglycans found in cartilage.79 Gelatin has been widely used in TE research as it is a derivate of collagen, a prominent ECM molecule of cartilage that has adhesion sites to chondrocytes.80 The combination of CS, HA, and gelatin displays great potential for cartilage TE as derivatives of the ECM. However, like most natural polymers, they lack good mechanical properties. To develop biomimetic hydrogels with improved mechanical properties, these are usually combined with water-soluble synthetic polymers, e.g. such as polyethylene glycol (PEG).81 Below, a further description is given of these materials used for cartilage regenerative approaches.
Chondroitin sulphate
Chondroitin sulphate (CS) is a polysaccharide molecule and the most abundant GAG found in the human body, which makes up 80% of GAGs in adult articular cartilage.82,83 CS is a linear sulphated GAG with repeating D-glucuronic acid and D-N-acetylgalactosamine units.84 It has the ability for tissue integration and exhibits an anti-inflammatory response.85 With ageing, the content of CS in cartilage decreases due to degeneration.86 CS is reported to be more effective in improving MSC chondrogenesis compared to heparan sulphates, especially in low stiffness. This is beneficial because a new matrix deposition accompanied by increasing mechanical properties occurs due to the neocartilage deposited by the cell.87 Due to its high charge density, it has a high water content, which results in mechanical weakness. Hence, CS is usually combined and crosslinked with other materials for enhanced mechanical properties.82,88,89 Chondrogenic regeneration has been widely studied using CS-based hydrogels. Previous studies on CS combined with pullulan hydrogels showed good viability of the encapsulated chondrocytes, displaying enhanced chondrogenesis in both self-crosslinked and enzymatically crosslinked hydrogels.90,91 CS-based hydrogels have been shown to exhibit the highest gene expression and matrix accumulation throughout the cartilage zones compared to HA- and type I collagen-based hydrogels.92
Hyaluronic acid
Hyaluronic acid (HA) is a non-sulphated GAG with a repeating disaccharide pattern, which is distributed within cartilage. In cartilage formation, HA is reported to induce MSC differentiation into chondrocytes, to maintain chondrocyte phenotype, and to increase the ECM deposition in cartilage.116 However, the in vivo use of HA remains a challenge due to unsatisfactory cell adhesion ability as it can be degraded by hyaluronidase, nitrogen and reactive oxygen. It can induce inflammation due to foreign objects.93
Gelatin
Gelatin is a biodegradable natural polymer with single-stranded protein from collagen as a result of partial hydrolysis, preserving Arg-Gly-Asp (RGD) motifs for cells to adhere to.94,95 It has been widely used in tissue engineering applications, as it is a low-cost polymer with minimal immunogenicity and tunable degradability.51 Furthermore, it is easily soluble in water at 37 °C and amphoteric. Gelatin consists of fractions derived from collagen alpha chains, maintaining a typical aminoacidic sequence of proline, hydroxyl proline and glycine.96 Promising results for in vitro MSC proliferation have been shown in the Pierce et al. study, where they use a gelatin-based hydrogel with few modifiable mechanical properties at various concentrations of ethyl lysine diisocyanate. Bone marrow-derived MSCs were viable for nine days and displayed low toxicity.97 Hydrogels using bovine-sourced GEL-MA crosslinked with UV polymerisation displayed the most similar environment to native cartilage after 28 days of culture compared to porcine-derived GEL-MA.98 Moreover, the addition of gelatin to other composite materials, such as alginate, has shown to increase their viability and chondrogenesis compared to alginate alone.99
The use of synthetic and water-soluble polymers such as PEG has become attractive due to their biocompatibility and ability to prevent the nonspecific absorption of protein and neutral charge.100 They can act as spacer agents, hence resulting in a more effective and controllable way to link two molecules.81 PEG is the most used synthetic hydrogel for tissue engineering, given its inert nature chemically and physically.101 It is also biodegradable and non-immunogenic. PEG also displays a non-toxic and highly soluble nature with good mechanical properties.102,103
Biomimetic hydrogels: mechanical and biological properties
Biomimetic hydrogels might mimic cartilage ECM composition and/or organisation to achieve biological and mechanical properties similar to those of the native tissue. For instance, natural materials such as CS and gelatin are non-toxic with tunable biodegradability; however, they have poor mechanical properties, which limit their use for tissue engineering.104 For this reason, the combination of natural materials with synthetic materials is used for achieving improved overall hydrogels’ biological and mechanical properties. The reported compressive modulus of articular cartilage105 is 0.02–1.16 MPa in the superficial zone and 6.44–7.75 MPa in the deep zone,7 while in osteoarthritic cartilage, it may range from 2 MPa to 30 MPa.17 A summary of selected works, in which natural materials such as CS, gelatin and HA are combined with synthetic ones such as PEG, is shown in Table 2 and extended to Fig. 3.
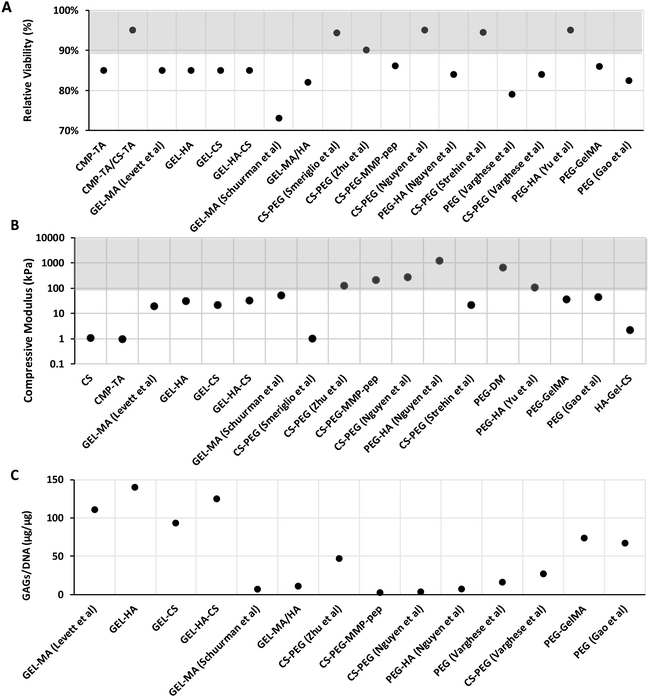 |
| Fig. 3 Compressive modulus, viability, and GAG/DNA of selected hydrogels. (A) Cell viability; (B) compressive modulus; and (C) GAG/DNA of hydrogels for cartilage regeneration; gray-shaded areas indicate the desired value for cartilage applications. | |
Table 2 Summary of selected hydrogels based on PEG, CS, HA and gelatin for cartilage regeneration
Hydrogel |
Crosslinkers |
Time-point |
Cells |
Findings |
Viability, ECM Production (μg μg−1), compressive modulus |
CS: Chondroitin sulfate; CMP: Carboxymethyl pullulan; DM: Dimethacrylate; EDAG: Ethylenediamine graphene; GEL: Gelatin; GELMA: Gelatin methacrylates; HA: Hyaluronic acid; LA: 4, 5 lactic acid; MA: Methacrylates; MMPpep: Matrix metalloproteinase-sensitive peptides; PEG: Polyethylene glycol; TA: Tyramine. |
CS-based (with graphene)106 |
EDC/NHS |
3 weeks |
MSCs |
EDAG-CS porous hydrogels exhibit higher mechanical properties, but degrade slower than CS-CS. EDAG-CS and CS-CS produce type II collagen and MSCs do not differentiate to endochondral ossification |
Cell viability and ECM production not reported |
Compressive modulus: 1.12 kPa |
Carboxymethylated pullulan CMP-TA/CS-TA90 |
Enzymatic crosslinking (horseradish peroxides and H2O2) |
14 days |
Porcine auricular chondrocytes |
Hydrogel with a CMP-TA/CS-TA weight ratio of 3/1 provided best host tissue-mimetic microenvironment. |
Viability: 80–95% (CMP-TA), >95% (CMP-TA/CS-TA) |
ECM production not reported |
Compressive modulus (CMP-TA): 0.96 kPa |
GEL-based107 |
UV photo-polymerization |
3 days, 4 weeks |
Chondrocytes |
Supplementation of HA to increase viscosity and PCL polymer for structural support enables the build-up of a layered hydrogel structure. Cell viability detected until 28 days using GAG/DNA. |
Viability: 73 ± 2% (GEL-MA), 82 ± 8% (GEL-MA-HA) (day 3) |
GAG/DNA: 7.1 ± 3.3 (GEL-MA), 10.5 ± 5.2 (GEL-MA-HA) (week 4) |
Compressive modulus: 32.28–53.54 kPa (GEL-MA) |
CS-PEG108 |
UV photo-polymerization |
24 hours, 3 weeks |
Juvenile, adult, and osteoarthritic chondrocytes |
7% w/v of PEGDA and 3% w/v. Deposition of ECM and long-term cell viability are reported. |
Viability: 94% |
ECM production not reported |
Compressive modulus: 0.73–1.04 kPa (day 1, week 3) |
CS-PEG109 |
Photo-polymerization |
3 weeks |
Neonatal bovine chondrocytes |
Dual gradient hydrogel with interconnected chambers (20% w/v PEG, 3% w/v CS; zone 1; to 2% w/v PEG, 8% w/v CS; zone 5) with better mechanical properties, cell proliferation and matrix deposition than CS hydrogel gradient alone. |
Viability: >90% in all zones (7 days) |
GAG/DNA: 40.58–53.72 kPa (zone 1–zone 5) |
Compressive modulus: 9.2 kPa–124.7 kPa (zone 1–zone 5) |
CS-PEG |
UV photo-polymerization |
2, 4, 6 weeks |
D1 mouse bone marrow progenitor cell |
Higher collagen II content was detected in the superficial layer (PEG : CS : MMP-pep), collagen X content and GAG production were increasing from the superficial layer, transitional (PEG : CS) to the deep layer (PEG : HA) mimicking the cartilage layer. |
Viability: 86% (PEG-CS-MMP-pep), >95% (CS-PEG), 84% (PEG-HA) |
PEG-CS-MMP-pep |
GAG/DNA: PEG-CS-MMP-pep: 2.6; CS-PEG: 2.8; PEG-HA: 6.6 (2 weeks) |
PEG-HA110 |
Compressive modulus: PEG-CS-MMP-pep: 199.71 (120–209 kPa), CS-PEG: 203.95 (121–270 kPa); PEG-HA: 942.83 (271–1227 kPa) |
CS-PEG111 |
EDC/NHS |
24 hours (in vitro) |
Bovine articular chondrocytes |
Cells within hydrogel retain viability and metabolically active, minimal inflammatory response compared to PEG, stronger adhesion compared with fibrin in vivo. |
Viability: 93–96% |
4 weeks (in vivo) |
ECM production not reported |
Compressive modulus: 11.31–22.28 kPa |
CS-PEG112 |
UV photo-polymerization |
24 hours, 3, 6 weeks |
MSCs |
PEG-CS gels have 10 times stronger adhesion to cartilage tissue compared to fibrin, and decreased inflammatory response compared with PEG. |
Viability: 79% (PEG), 84% (CS-PEG) (24 hours) |
GAGs/DNA: PEG: 10.75 (week 3), 20.12 (week 6). CS-PEG: 18.11 (week 3), 36.18 (week 6) |
Compressive modulus not reported |
PEG-LA-DM |
UV photo-polymerization |
6 weeks (in vitro) |
Juvenile swine articular chondrocytes |
Encapsulated chondrocytes in 60/40 and 70/30 PEG-LA-DM/PEG-DM polymer ratios |
Cell viability and ECM production not reported |
PEG-DM113 |
6, 12, 18 weeks (in vivo) |
Improved cartilage matrix formation of aggrecan and collagen type II/VI |
Compressive modulus: 60–670 kPa (ref. 92) |
PEG-HA114 |
Enzymatic crosslinking (HRP and H2O2) and Diels–Alder click chemistry |
7 days |
Chondrogenic teratocarcinoma cells |
Enzymatic crosslinking hydrogels result in 5 minutes of gelation time, has great shape memory and anti-fatigue properties |
Viability: >95% |
No ECM production reported |
Compressive modulus: 60.7–109.4 kPa |
PEG-GelMa |
Photo-polymerization |
7 days, 3 weeks |
Human MSCs |
Inkjet bioprinted MSCs of PEG-MA show better mechanical properties than PEG, increased 63% under chondrogenic differentiation after 21 days. |
Viability: 85.87% (PEG-GelMa), 82.43% (PEG) |
GAGs/DNA: 73.81 (PEG-GelMa), 67.64 (PEG) (7 days) |
PEG115 |
Compressive modulus: 36.70 kPa (PEG-GelMa), 45.31 kPa (PEG) |
GEL-CS116 |
EDC/NHS and click chemistry |
1, 3 and 7 days |
Fibroblasts and macrophages |
Overall good cytocompatibility, mimicking cartilage ECM composition |
Viability: 10% CS–gelatin (79% MTT); |
Compressive modulus: not measured. |
ECM production: not measured |
GEL-CS117 |
Chemical crosslinking (glutaraldehyde, dithiothreitol/2-propanol); and photo-crosslinking (UV-light) |
21 days |
Human mesenchymal stem cells (MSCs) |
Spatially patterned μRB scaffold enabled MSCs to produce cartilage with biomimetic zonal biochemical and mechanical properties |
Viability: not measured quantitatively. |
Compressive modulus: trilayer μRB up to 456 kPa. |
ECM production: sGAG per d.w trilayer μRB up to 0.08 μg μg−1 (1.5–7.5% dry weight) |
GEL-HA118 |
Photo-crosslinking (UV-light) |
Up to 8 weeks |
Human bone marrow–derived mesenchymal stem cells (hBMSCs) and in vivo (New Zealand white rabbits) |
MSCs chondrogenesis, scaffolds at 9 : 1 (% w/v) ratio mGL/mHA lowest hBMSC hypertrophy and highest glycosaminoglycan production, supported cartilage formation in vivo after 12 weeks |
Viability: not measured quantitatively. |
Compressive modulus: up to 25 kPa (8.5/1.5 ratio mGL/mHA) |
ECM production: up to 17 μg GAG/construct |
GEL-HA119 |
Photo-crosslinking (UV-light) |
1, 4, 7 days |
Chondrocytes and in vivo (nude mice) |
At a relatively low cell-seeding concentration, homogeneous tubular cartilage regenerated with a tracheal shape, mechanical strength and cartilage ECM deposition |
Cell viability: about 98%. |
Storage modulus (G′) = 1 kPa. |
ECM production: no reported in vitro but in vivo (about 70 mg g−1 LDTM scaffold and 200 mg g−1 for Exp) |
GEL-HA120 |
Enzymatic crosslinking (HRP and H2O2) |
Up to 14 days |
Mesenchymal stem cells (MSCs) |
70% HA and 30% gel most promising for chondrogenesis, best mechanical properties |
Viability not measured quantitatively; cell proliferation decreases in hydrogels rich in HA. Compressive modulus (G′) up to 789 ± 220 Pa. |
ECM production = GAGs about 80% in the 30/70 mixture and HA gel. |
Collagen-CS-HA (CCH)121 |
Chemical crosslinking (Genipin, 0.75 mM) |
4 weeks |
Allogeneic chondrocytes (in vivo Rabbits model) |
CCH hydrogel provides structural and mechanical similarity to cartilage; used as a carrier of chondrocytes; helps cell growth and matrix synthesis |
Viability not measured quantitatively; cell proliferation increases in CCH hydrogels. |
Compressive modulus increases (CCH about 51.53 ± 0.54 kPa (ref. 122). |
GAG/DNA ratio about 4 (7 days, approx. 6 μg μl−1) |
HA-GEL-CS123 |
Diels–Alder click chemistry |
N/A |
N/A |
HA-furan GEL-furan, MAL-PEG-MAL |
Cell viability and ECM production not reported or tested |
HA-GEL-CS with EDC NHS |
Compressive modulus: 1.62–2.21 kPa |
HA-GEL-CS124 |
Click chemistry (Cu(I) as the catalyst) |
Up to 7 days |
Chondrocytes |
Good reactivity under very mild conditions by click-chemistry. In vitro cell culture confirmed that chondrocytes could adhere and proliferate on the hydrogel. |
Viability not measured quantitatively; cell number increases overtime. |
Compressive modulus ∼7 kPa |
ECM production: not measured |
GEL-MA |
Photo-crosslinking (UV light) |
1 day, 5 and 8 weeks |
Human chondrocytes |
The addition of CS and HA to GEL-MA can enhance the differentiation, improved mechanical properties, and ECM distribution. Significant COL2A1 expression |
Viability: 80–90% |
GEL-HA |
GAGs/DNA: 110.9 (GEL-MA), 140 (GEL-HA), 92.7 (GEL-CS), 125.3 (GEK-HA-CS) (week 8) |
GEL-CS |
Compressive modulus: 20.17 kPa (GEL-MA), 31.08 kPa (GEL-HA), 21.66 kPa (GEL-CS), 32.57 kPa (GEL-HA-CS) |
GEL-HA-CS125 |
Data related to mechanical (i.e. compressive modulus) and biological properties (i.e. cell viability and GAG/DNA content) of biomimetic hydrogel studies were collected from different journal databases (PubMed, Web of Science, and Google Scholar). From this review, it was found that most of the hydrogels found in the literature have a compression modulus ranging from 10 to 1000 kPa, meaning that formulated hydrogels are below cartilage values by at least a factor of 10 (Fig. 3A). Particularly, it can be observed that cell-free hydrogels based on PEG-HA110 (217–1227 kPa) show the highest compressive modulus when compared to PEG-DM113 (60–670 kPa) and CS-PEG110 (121–270 kPa). Interestingly, materials based on CS-PEG can show a very low compressive modulus (e.g. 0.73 kPa);108 this can be improved by varying the crosslinking method.109–111 Increased PEG concentration will result in a stiffer hydrogel.
The hydrogel should provide to the cells a suitable microenvironment that stimulates ECM production and new tissue formation. For instance, scaffolds that are too stiff can affect biological behaviour, such as changing the phenotype of the cells and driving MSC differentiation into bone cells.126,127 Moreover, it was found that by increasing substrate stiffness, chondrocyte ECM production is down-regulated while promoting adhesion and stress fibre formation.128,129 However, if the modulus is not adequate in early time points, chondrocyte will produce ECM that can strengthen the hydrogel structure over time and withstand higher compressive forces.130,131
Data on cellular viability (using LIVE/DEAD assays) collected from 24 hours up to 7 days following cell seeding are shown in Fig. 3B. In general, hydrogels show good cell viability (higher than 70%), particularly those based on proteoglycans like CS and HA. From relative viability data, the overall high viability was shown for hydrogels based on CMP-TA/CS-TA,90 CS-PEG,108,110,111 and PEG-HA,123 evidencing higher survival (>90%) when compared to hydrogels such as GEL-MA107 and PEG112 (<80%). Particularly, based on the latter, GEL-MA showed the lowest cell viability amongst the group, in contrast to other gelatin-based hydrogels.132 This effect may be due to the methacrylate process, which causes cytotoxicity. Methacrylate toxicity has been reported in several in vitro studies where glutathione (GSH), a major antioxidant that prevents oxidative stress, was removed. Increased oxidative stress caused by adduct formation of methacrylate results in cell toxicity.133,134 Regarding synthetic PEG-based hydrogels, these do not contain cell adhesion motifs and so increasing the PEG concentration or polymer block length in hydrogels might result in decreased or impaired cell adhesion and protein adsorption.135,136 GAG/DNA content is presented as the total accumulated GAG content normalised to the total DNA content of a selection of biomimetic hydrogels in Fig. 3C. In terms of ECM production, the gelatin-based hydrogel seems to stimulate GAG secretion, except for GEL-MA.107 The gelatin-based hydrogel showed the highest levels of GAG/DNA deposition; particularly, GEL-HA had the highest GAG accumulation, followed by GEL-HA-CS.125 The use of HA has been shown to enhance ECM remodelling into a more ordered collagen deposition, and increased the mRNA expression of cartilage-specific genes.137,138 Consistent results were shown where the addition of HA produces a higher GAG content.107,110,125 However, it appears to be concentration-dependent, where the chondrogenic properties are only shown at low concentrations, while no effect or a negative effect on chondrogenesis is observed at higher HA concentrations.107
In contrast, the lowest GAG/DNA production was found when the cells were incubated in hydrogels containing PEG.110–112 One way to overcome this problem is to incorporate other polymers. Varghese et al. have shown that incorporation of CS into PEG hydrogels results in enhanced chondrogenic gene expression and cartilage matrix production compared to PEG alone.112 Moreover, Zhu et al. hypothesised that a stiffer hydrogel could be made by increasing the PEG and CS concentrations in a layer-by-layer manner, resulting in more ECM production using a mechanical gradient hydrogel via cellular remodelling of the ECM by degradation.109 Interestingly, the combination of GEL-MA and PEG favours ECM production and promotes MSC chondrogenic differentiation.115 Bryant et al. reported that incorporation of degradable crosslinkers can promote GAGs to diffuse out of the gel without sacrificing the mechanical properties.139 In contrast, studies have suggested that CS can inhibit ECM production, despite it not impacting the chondrocyte viability.109,112 This phenomenon may be caused by the negative charge of CS that attracts free cations from the culture medium, which increases hydrogel osmolarity and impacts cell growth negatively.140 Moreover, the addition of signalling molecules such as TGF-β1 can be considered for future research to improve cellular function such as cell proliferation and ECM secretion in synthetic-based hydrogels.141
In the last few years, improved cartilage ECM-like hydrogels based on CS, HA and gelatin have been formulated in the absence of synthetic polymers such as PEG. Improved mechanical and biological performance has been achieved by exploiting the recent advances in physical and chemical crosslinking including click chemistries, photo-crosslinking, enzyme-mediated crosslinking, and interpenetrating double networks. Mechanical properties similar to native cartilage have been obtained for biomimetic hydrogels based on ECM-derived proteins,121,125 achieving a compressive Young's modulus up to 456 kPa for spatially patterned μRB scaffolds based on CS-GEL hydrogels.117 In this context, excellent mechanical and biological performance including GAG deposition and cellular viability have also been obtained for hydrogels that combine ECM-derived materials such as HA and GEL.119,121,125 These properties were achieved by combining the biological cues present in the native cartilage ECM while reinforcing their structure with the presence of non-toxic crosslinking strategies without compromising hydration or cyto-/biocompatibility. In general, naturally derived biomaterials and combined ECM proteins in hydrogels (e.g. CS, HA, gelatin) are known as ideal biomimetic sources for cartilage tissue engineering. However, some of the key limitations, including weak mechanical properties for mimicking the surrounding tissues and control of structure and degradation rates, have limited their applications. In this overview, we have shown that recent advances in chemical or physical modifications and fabrication methods are capable of overcoming some of these limitations, demonstrating that these materials can mimic native ECM tissues and support cartilage regeneration while showing superior functional performance when compared to single or biosynthetic formulations.
Future perspective on cartilage tissue engineering
For the past 5 years, there has been growing interest in hydrogel research for cartilage regeneration. Moreover, it has been shown that hydrogels can provide a suitable environment for chondrocytes and MSCs to grow from in vitro and in vivo research. Hydrogels capable of mimicking properties and biological activities, structure and organisation of the native tissue are needed for developing TE strategies, disease models and cell therapies.
In vitro cartilage tissue models
Zonally stratified structures similar to native cartilage can be achieved by exploiting combinations of materials and composite hydrogels processed additively. Therefore, a gradient hydrogel processing technique, such as bioprinting and controlled fluid mixing, allows the creation of multiple layers with a physical and chemical composition that mimics cartilage zones.142,143 Biomimetic multiphasic hydrogels and structures have been developed to mimic the native osteochondral tissue properties,144 including monophasic, bi-phasic and tri-phasic structures. For instance, mussel-inspired hydrogels with a bilayer structure have shown potential to repair osteochondral defects following implantation in rabbit knee joints.145 Another example is the biphasic CAN-PAC hydrogel for the regeneration of osteochondral defects prepared by thermally initiated free radical polymerization, which showed a low inflammatory response and ingrowth of newly formed tissue when subcutaneously implanted in rats.146 Moreover, advances in tissue engineering by combining hydrogels and bioprinting technologies to manufacture relevant cellular microniches present new exciting avenues for tissue modelling, ageing and disease mechanism discovery and therapy towards reducing the need for costly and unethical animal models. Photo-cross-linkable GEL-HA has been fabricated as a porous scaffold for cartilage repair with a suitable internal pore structure and high mechanical strength via photocuring 3D printing and lyophilization.147 The encapsulation of chondrocytes into the scaffolds promoted mature cartilage regeneration with a typical lacunae structure and cartilage-specific ECM both in vitro and in vivo. Recent works on the bioprinting of biomimetic hydrogels for cartilage applications showed a great interest in using functionalised natural components148 such as gelatin, chondroitin sulphate, and hyaluronic acid towards recapitulating the complex zonal microarchitecture of native hyaline cartilage,129 and creating anatomically accurate constructs of stratified cartilage tissues and osteochondral tissue interfaces.149,150
Cartilage is a mechanoresponsive tissue, and dynamic stresses play an important role in cartilage development and homeostasis.151 However, the mechanotransduction pathways, including mechanisms and parameters involved are poorly understood, presenting a major growth area with the potential for discovering new therapeutic approaches in cartilage ageing and disease. Still, advancements for tissue engineering require more reliable assays for the analysis and characterisation of cell behaviour in 3D structures, and for assessing the influence of biomechanical cues on cartilage development. In terms of assessing 3D cartilage-like structures, there is a fundamental need for more reliable assays for in vitro assessment and standardisation of protocols, as most available products are specifically made for 2D culture.
In silico modelling of cartilage tissue
To achieve the desired properties of the hydrogels, generally a significant amount of iterations in multi-variant systems is needed for the design, manufacturing and characterisation of these systems.152 One promising strategy is the emerging concept of rational design that is based on computationally guided biomaterial design strategies.153 These have been shown to be very suitable when multiple design variables need to be considered. For selecting a biomaterial with suitable mechanical properties, Bas et al.154 developed a numerical model-based approach for the rational design of biomimetic soft network composites to be used in cartilage regeneration. They established a design library capable of predicting the compressive modulus of the designed biomaterials using numerical tools from compressive modulus and Poisson's ratio data of hydrogels found in the literature. This library was created specifically for soft hydrogels (E = ∼10–50 kPa) that can be applied for encapsulating different cells such as human chondrocytes.
Furthermore, a computational model can help to investigate and clarify some of the most significant mechanisms that influence the growth and degradation of the hydrogels used in regenerative medicine. In this regard, an interesting mechanics-based model of tissue growth in degradable cell-laden hydrogels has been reported recently by Sridhar et al.155 In this continuum-based multiphasic model, the authors assumed that, after encapsulating cells in a PEG-based hydrogel, hydrolysis of polymer crosslinks was taking place following a first order kinetic law.156 Then, consequently to the crosslink cleavage, they assumed a release of ECM precursors from the cells to assemble large ECM molecules of aggrecan and collagens. Interestingly, applying their model, they found that well-linked and dense cell agglomerates combined with large areas of weak cross-linking around cells could generate the right conditions/factors for the formation and growth of new tissue while maintaining structural integrity. Similarly, Akalp et al.157 developed a model for interstitial growth, based on mixture theory, which could be used to investigate the influence of cell-laden hydrogel degradation. This was also due to the crosslink cleavage mediated by sensitive enzymes and the transport/diffusion of ECM molecules released by the cells. Upon application to the cartilage tissue, their numerical results showed that the enzymatic degradation produced the presence of a very localized degradation front spreading away from the cells, which could produce immediately a front of growing neo-tissue. Even if these studies utilised real experimental data for the model validation, they did not take in to account the in vivo conditions. In this scenario, from a more etiopathological view, Stender et al.158 developed a FE model to study the changes of osteoarthritis-induced articular cartilage damage and the remodelling of subchondral cortical and trabecular bones.
Finally, in spite of the efficiency of the computational models in identifying potential growth mechanisms and supporting the experimental plan, the future models are expected to be able to be used for personalised tissue engineering. This is since the changes in cell behaviour can strongly influence the formation and growth of neo-tissues as well as the dynamic degradation mechanisms of the hydrogels.159
Cell therapies for cartilage regeneration
Degenerative skeletal diseases such as osteoarthritis affect more than 200 million people worldwide. Current strategies of joint replacements lead to the removal of cartilage and healthy bone tissues. Moreover, joint devices and implants have a limited lifespan, requiring revision surgeries within 20 years, so there is a need to move from tissue replacement to tissue regeneration. Matrix autologous chondrocyte implantation (MACI) cell therapies are as expensive as £17
740 (ChondroCelect®, withdrawn from the European Union) per procedure with insufficient evidence of cost-effectiveness.160 One of the major limitations of MACI is increased timings for patient rehabilitation, caused by poor mechanical and structural integrity of implanted materials before tissue regeneration.161 MACI, which was approved in Europe, is also suspended as of 2017.
Autologous chondrocyte implantation (ACI) is available in Europe and the UK (since 2017) for treating symptomatic articular cartilage defects (>2 cm2) of the knee.162 However, ACI is successful for approximately 80% of patients, but for the remaining 20% can produce tissue delamination and hypertrophy of the implantation site.163 Similarly, a scaffold-free spheroid, Chondrosphere (Spherox), has been approved by the EMA in 2017. Chondrosphere versus MACI was £18
000 per quality-adjusted life-year gained.164 For these cell therapies, new biomimetic hydrogels and materials have the potential to improve the cell therapy outcomes, while decreasing the associated costs. Advanced biomaterials are needed to provide the best environment and support for cartilage cells to function. It is important to note that products containing active biomolecules and/or genes require more complex procedures, regulation, and funding which can limit the sustainability.13
In the US market, FDA-approved cell-free hydrogels are Gel-One®, Synvisc and Synvisc-One®.165,166 In contrast, products that are hydrogel-based with autologous chondrocytes include CaReS®, a type I collagen, and Cartipatch®, which uses an agarose–alginate hydrogel. Cartipatch® has a phase III study terminated and CaRes® is only available in a few selected European countries, Iran, China, and Turkey167,168
In summary, this study investigated the mechanical and biological properties of widely used hydrogel materials such as PEG, CS, gelatin, and other materials, for cartilage regeneration. From the literature review, we find that the overall viability of hydrogels based on PEG, CS, or gelatin is high. GAG-based hydrogels namely CS and HA have the highest viability compared to the rest. In general, PEG-based hydrogels have the highest Young's modulus though it is still not adequate compared to the natural articular cartilage compression modulus. Incorporation of additional materials may influence the mechanical properties and viability of chondrocytes. Variation of stiffness and material composition affect chondrocytes to form the matrix. However, research on matrix stiffness and chemistry of hydrogels on cell behaviour is still conflicting. Further research on the effect of stiffness on cellular proliferation, differentiation, ECM production, and migration is needed. Therefore, future studies should focus on investigating the physical, chemical, and biological properties of PEG, CS, and gelatin for use in hydrogels as a scaffold for cartilage regeneration.
Conflicts of interest
The authors declare no conflicts of interest.
Acknowledgements
Alexander Stokes acknowledges support from the EPSRC for his Ph.D. studentship (EPSRC Grant EP/R51309X/1).
References
-
H. Huang, H. Xu and J. Zhang, in Cartilage Tissue Engineering and Regeneration Techniques, IntechOpen, 2019 Search PubMed.
- S. L. Kolasinski, T. Neogi, M. C. Hochberg, C. Oatis, G. Guyatt, J. Block, L. Callahan, C. Copenhaver, C. Dodge and D. Felson, Arthritis Rheumatol., 2020, 72, 220–233 CrossRef PubMed.
- S. L. Vega, M. Y. Kwon and J. A. Burdick, Eur. Cells Mater., 2017, 33, 59 CrossRef CAS PubMed.
- C. W. Archer and P. Francis-West, Int. J. Biochem. Cell Biol., 2003, 35, 401–404 CrossRef CAS PubMed.
- A. J. Sophia Fox, A. Bedi and S. A. Rodeo, Sports Health, 2009, 1, 461–468 CrossRef PubMed.
- M. Liu, X. Zeng, C. Ma, H. Yi, Z. Ali, X. Mou, S. Li, Y. Deng and N. He, Bone Res., 2017, 5, 1–20 CrossRef PubMed.
- S. Chen, Y. Falcovitz, R. Schneiderman, A. Maroudas and R. Sah, Osteoarthr. Cartil., 2001, 9, 561–569 CrossRef CAS PubMed.
- D. R. Eyre, Clin. Orthop. Relat. Res., 2004, 427, S118–S122 CrossRef PubMed.
-
N. Gudmann and M. Karsdal, in Biochemistry of Collagens, Laminins and Elastin, Elsevier, 2016, pp. 73–76 Search PubMed.
- Q. Chai, Y. Jiao and X. Yu, Gels, 2017, 3, 6 CrossRef PubMed.
- A. J. Sophia Fox, A. Bedi and S. A. Rodeo, Sports health, 2009, 1(6), 461–468 CrossRef PubMed.
- F. J. O′brien, Mater. Today, 2011, 14, 88–95 CrossRef.
- D. F. Williams, Front. Bioeng. Biotechnol., 2019, 7, 127 CrossRef PubMed.
- L. Huang, J. Hu, L. Lang, X. Wang, P. Zhang, X. Jing, X. Wang, X. Chen, P. I. Lelkes and A. G. MacDiarmid, Biomaterials, 2007, 28, 1741–1751 CrossRef CAS PubMed.
-
J. Parsons, in Handbook of biomaterial properties, Springer, 1998, pp. 40–47 Search PubMed.
- W. Hayes and L. Mockros, J. Appl. Physiol., 1971, 31, 562–568 CrossRef CAS PubMed.
- S. Akizuki, V. C. Mow, F. Müller, J. C. Pita, D. S. Howell and D. H. Manicourt, J. Orthop. Res., 1986, 4, 379–392 CrossRef CAS PubMed.
- F. Couet, N. Rajan and D. Mantovani, Macromol. Biosci., 2007, 7, 701–718 CrossRef CAS PubMed.
- B. Marelli, M. Achilli, A. Alessandrino, G. Freddi, M. C. Tanzi, S. Farè and D. Mantovani, Macromol. Biosci., 2012, 12, 1566–1574 CrossRef CAS PubMed.
- D. Pankajakshan and D. K. Agrawal, Can. J. Physiol. Pharmacol., 2010, 88, 855–873 CrossRef CAS PubMed.
- M. Achilli, J. Lagueux and D. Mantovani, Macromol. Biosci., 2010, 10, 307–316 CrossRef CAS PubMed.
- A. M. Ferreira, P. Gentile, S. Sartori, C. Pagliano, C. Cabrele, V. Chiono and G. Ciardelli, Biotechnol. J., 2012, 7, 1386–1394 CrossRef CAS PubMed.
- D. A. Gyles, L. D. Castro, J. O. C. Silva Jr. and R. M. Ribeiro-Costa, Eur. Polym. J., 2017, 88, 373–392 CrossRef CAS.
- N. J. Einerson, K. R. Stevens and W. J. Kao, Biomaterials, 2003, 24, 509–523 CrossRef CAS PubMed.
- S. Kapoor and S. C. Kundu, Acta Biomater., 2016, 31, 17–32 CrossRef CAS PubMed.
- M. J. Rodriguez, J. Brown, J. Giordano, S. J. Lin, F. G. Omenetto and D. L. Kaplan, Biomaterials, 2017, 117, 105–115 CrossRef CAS PubMed.
- M. Kumar, P. Gupta, S. Bhattacharjee, S. K. Nandi and B. B. Mandal, Biomaterials, 2018, 187, 1–17 CrossRef CAS PubMed.
- A. Armiento, M. Stoddart, M. Alini and D. Eglin, Acta Biomater., 2018, 65, 1–20 CrossRef CAS PubMed.
- L. Lapčík, L. Lapcik, S. De Smedt, J. Demeester and P. Chabrecek, Chem. Rev., 1998, 98, 2663–2684 CrossRef PubMed.
- R. L. Jackson, S. J. Busch and A. D. Cardin, Physiol. Rev., 1991, 71, 481–539 CrossRef CAS PubMed.
- J. Baier Leach, K. A. Bivens, C. W. Patrick Jr. and C. E. Schmidt, Biotechnol. Bioeng., 2003, 82, 578–589 CrossRef PubMed.
- A. Scalzone, M. A. Bonifacio, S. Cometa, F. Cucinotta, E. De Giglio, A. M. Ferreira and P. Gentile, Front. Bioeng. Biotechnol., 2020, 8, 712 CrossRef PubMed.
- P. Rastogi and B. Kandasubramanian, Biofabrication, 2019, 11, 042001 CrossRef CAS PubMed.
- A. D. Augst, H. J. Kong and D. J. Mooney, Macromol. Biosci., 2006, 6, 623–633 CrossRef CAS PubMed.
- P. Gentile, C. Ghione, A. Ferreira, A. Crawford and P. Hatton, Biomater. Sci., 2017, 5, 1922–1931 RSC.
- S. Peers, A. Montembault and C. Ladavière, J. Controlled Release, 2020, 326, 150–163 CrossRef CAS PubMed.
- Y. Xie, X. Liao, J. Zhang, F. Yang and Z. Fan, Int. J. Biol. Macromol., 2018, 119, 402–412 CrossRef CAS PubMed.
- K. E. Benders, P. R. van Weeren, S. F. Badylak, D. B. Saris, W. J. Dhert and J. Malda, Trends Biotechnol., 2013, 31, 169–176 CrossRef CAS PubMed.
- C.-C. Lin and K. S. Anseth, Pharm. Res., 2009, 26, 631–643 CrossRef CAS PubMed.
- J. Zhu, Biomaterials, 2010, 31, 4639–4656 CrossRef CAS PubMed.
- Z. Fan, P. Cheng, M. Liu, D. Li, G. Liu, Y. Zhao, Z. Ding, F. Chen, B. Wang and X. Tan, New J. Chem., 2017, 41, 8656–8662 RSC.
- B. Liu, W. Huang, G. Yang, Y. An, Y. Yin, N. Wang and B. Jiang, Mater. Sci. Eng., C, 2020, 116, 111259 CrossRef CAS PubMed.
- S. P. Higgins, A. K. Solan and L. E. Niklason, J. Biomed. Mater. Res., Part A, 2003, 67, 295–302 CrossRef PubMed.
- H. Liu, E. B. Slamovich and T. J. Webster, Int. J. Nanomed., 2006, 1, 541 CrossRef CAS PubMed.
- S. A. Munim and Z. A. Raza, J. Porous Mater., 2019, 26, 881–901 CrossRef CAS.
- B. Gupta, N. Revagade and J. Hilborn, Prog. Polym. Sci., 2007, 32, 455–482 CrossRef CAS.
- B. Dhandayuthapani, Y. Yoshida, T. Maekawa and D. S. Kumar, Int. J. Polym. Sci., 2011, 2011, 290602 Search PubMed.
- A. Kosik-Kozioł, M. Costantini, T. Bolek, K. Szöke, A. Barbetta, J. Brinchmann and W. Święszkowski, Biofabrication, 2017, 9, 044105 CrossRef PubMed.
- A. Basu, K. R. Kunduru, S. Doppalapudi, A. J. Domb and W. Khan, Adv. Drug Delivery Rev., 2016, 107, 192–205 CrossRef CAS PubMed.
- W. Bao, M. Li, Y. Yang, Y. Wan, X. Wang, N. Bi and C. Li, Front. Chem., 2020, 8, 53 CrossRef CAS PubMed.
- M. C. Catoira, L. Fusaro, D. Di Francesco, M. Ramella and F. Boccafoschi, J. Mater. Sci. Mater. Med., 2019, 30, 115 CrossRef PubMed.
- B. Johnstone, M. Alini, M. Cucchiarini, G. R. Dodge, D. Eglin, F. Guilak, H. Madry, A. Mata, R. L. Mauck and C. E. Semino, Eur. Cells Mater., 2013, 25(248), e67 Search PubMed.
- L. Han, M. Wang, P. Li, D. Gan, L. Yan, J. Xu, K. Wang, L. Fang, C. W. Chan and H. Zhang, ACS Appl. Mater. Interfaces, 2018, 10, 28015–28026 CrossRef CAS PubMed.
- J. Kim, C. Lee and J. H. Ryu, Appl. Sci., 2021, 11, 21 CrossRef CAS.
- K. R. Hixon, S. J. Bogner, G. Ronning-Arnesen, B. E. Janowiak and S. A. Sell, Gels, 2019, 5, 21 CrossRef CAS PubMed.
- M. A. Bonifacio, S. Cometa, A. Cochis, P. Gentile, A. M. Ferreira, B. Azzimonti, G. Procino, E. Ceci, L. Rimondini and E. De Giglio, Carbohydr. Polym., 2018, 198, 462–472 CrossRef CAS PubMed.
- H. Fan and J. P. Gong, Macromolecules, 2020, 53, 2769–2782 CrossRef CAS.
- W. He, M. Reaume, M. Hennenfent, B. P. Lee and R. Rajachar, Biomater. Sci., 2020, 8(12), 3248–3269 RSC.
- X. Xu, V. V. Jerca and R. Hoogenboom, Mater. Horiz., 2021 10.1039/D0MH01514H.
- S. Khan and N. M. Ranjha, Polym. Bull., 2014, 71, 2133–2158 CrossRef CAS.
- W. Hu, Z. Wang, Y. Xiao, S. Zhang and J. Wang, Biomater. Sci., 2019, 7, 843–855 RSC.
- Y. H. Tan, J. R. Schallom, N. V. Ganesh, K. Fujikawa, A. V. Demchenko and K. J. Stine, Nanoscale, 2011, 3(8), 3395–3407 RSC.
- J. Wu, Q. Chen, C. Deng, B. Xu, Z. Zhang, Y. Yang and T. Lu, Theranostics, 2020, 10, 9843 CrossRef CAS PubMed.
- S. H. Park, J. Y. Seo, J. Y. Park, Y. B. Ji, K. Kim, H. S. Choi, S. Choi, J. H. Kim, B. H. Min and M. S. Kim, NPG Asia Mater., 2019, 11, 1–16 Search PubMed.
- P. Nezhad-Mokhtari, M. Ghorbani, L. Roshangar and J. S. Rad, Int. J. Biol. Macromol., 2019, 139, 760–772 CrossRef CAS PubMed.
- C. Echalier, L. Valot, J. Martinez, A. Mehdi and G. Subra, Mater. Today Commun., 2019, 20, 100536 CrossRef CAS.
- Y. Zhang, Y. Cao, H. Zhao, L. Zhang, T. Ni, Y. Liu, Z. An, M. Liu and R. Pei, J. Mater. Chem. B, 2020, 8, 4237–4244 RSC.
- C.-C. Tsai, S.-H. Kuo, T.-Y. Lu, N.-C. Cheng, M.-Y. Shie and J. Yu, ACS Biomater. Sci. Eng., 2020, 6, 5110–5119 CrossRef CAS.
- R. Parhi, Adv. Pharm. Bull., 2017, 7, 515–530 CrossRef CAS PubMed.
- G. C. Ingavle, N. H. Dormer, S. H. Gehrke and M. S. Detamore, J. Mater. Sci. Mater. Med., 2012, 23, 157–170 CrossRef CAS PubMed.
- E. S. Dragan, Chem. Eng. J., 2014, 243, 572–590 CrossRef CAS.
- E. S. Dragan, Pure Appl. Chem., 2014, 86, 1707–1721 CAS.
- T. Nonoyama and J. P. Gong, Proc. Inst. Mech. Eng., Part H, 2015, 229, 853–863 CrossRef PubMed.
- Y. Zhao, T. Nakajima, J. J. Yang, T. Kurokawa, J. Liu, J. Lu, S. Mizumoto, K. Sugahara, N. Kitamura and K. Yasuda, Adv. Mater., 2014, 26, 436–442 CrossRef CAS.
- L. Li, K. Zhang, T. Wang, P. Wang, B. Xue, Y. Cao, L. Zhu and Q. Jiang, Mater. Des., 2020, 189, 108492 CrossRef CAS.
- A. K. Means, C. S. Shrode, L. V. Whitney, D. A. Ehrhardt and M. A. Grunlan, Biomacromolecules, 2019, 20, 2034–2042 CrossRef CAS PubMed.
- B. G. Cooper, R. C. Stewart, D. Burstein, B. D. Snyder and M. W. Grinstaff, Angew. Chem., 2016, 128, 4298–4302 CrossRef.
- L. Trachsel, C. Johnbosco, T. Lang, E. M. Benetti and M. Zenobi-Wong, Biomacromolecules, 2019, 20, 4502–4511 CrossRef CAS PubMed.
- J. A. Buckwalter and E. B. Hunziker, Lancet, 1996, 348, S18 CrossRef.
- A. B. Bello, D. Kim, D. Kim, H. Park and S.-H. Lee, Tissue Eng., Part B, 2020, 26, 164–180 CrossRef CAS PubMed.
- S. M. Ryan, G. Mantovani, X. Wang, D. M. Haddleton and D. J. Brayden, Expert Opin. Drug Delivery, 2008, 5, 371–383 CrossRef CAS PubMed.
- Y. Alinejad, A. Adoungotchodo, E. Hui, F. Zehtabi and S. Lerouge, Int. J. Biol. Macromol., 2018, 113, 132–141 CrossRef CAS.
- N. Kuiper and A. Sharma, Osteoarthr. Cartil., 2015, 23, 2233–2241 CrossRef CAS PubMed.
-
J. Roberts and P. Martens, in Biosynthetic Polymers for Medical Applications, Elsevier, 2016, pp. 205–239 Search PubMed.
- D.-A. Wang, S. Varghese, B. Sharma, I. Strehin, S. Fermanian, J. Gorham, D. H. Fairbrother, B. Cascio and J. H. Elisseeff, Nat. Mater., 2007, 6, 385–392 CrossRef CAS PubMed.
- E. C. Collin, O. Carroll, M. Kilcoyne, M. Peroglio, E. See, D. Hendig, M. Alini, S. Grad and A. Pandit, Signal Transduction Targeted Ther., 2017, 2, 1–8 Search PubMed.
- T. Wang and F. Yang, Stem Cell Res. Ther., 2017, 8, 1–12 CrossRef CAS PubMed.
- L. Zhou, L. Fan, F.-M. Zhang, Y. Jiang, M. Cai, C. Dai, Y.-A. Luo, L.-J. Tu, Z.-N. Zhou and X.-J. Li, Bioact. Mater., 2020, 6, 890–904 CrossRef PubMed.
- X. Bai, S. Lü, Z. Cao, B. Ni, X. Wang, P. Ning, D. Ma, H. Wei and M. Liu, Carbohydr. Polym., 2017, 166, 123–130 CrossRef CAS PubMed.
- F. Chen, S. Yu, B. Liu, Y. Ni, C. Yu, Y. Su, X. Zhu, X. Yu, Y. Zhou and D. Yan, Sci. Rep., 2016, 6, 20014 CrossRef CAS PubMed.
- T. Li, X. Song, C. Weng, X. Wang, L. Sun, X. Gong, L. Yang and C. Chen, Appl. Mater. Today, 2018, 10, 173–183 CrossRef.
- N. S. Hwang, S. Varghese, H. J. Lee, P. Theprungsirikul, A. Canver, B. Sharma and J. Elisseeff, FEBS Lett., 2007, 581, 4172–4178 CrossRef CAS PubMed.
- Z. Zhu, Y. M. Wang, J. Yang and X. S. Luo, Plast. Aesthet. Res., 2017, 4, 219–227 CrossRef.
-
S. Van Vlierberghe, G.-J. Graulus, S. K. Samal, I. Van Nieuwenhove and P. Dubruel, Biomedical Foams for Tissue Engineering Applications, Elsevier, 2014, pp. 335–390 Search PubMed.
- Z. Mũnoz, H. Shih and C.-C. Lin, Biomater. Sci., 2014, 2, 1063–1072 RSC.
- P. Jaipan, A. Nguyen and R. J. Narayan, MRS Commun., 2017, 7, 416–426 CrossRef CAS.
- B. F. Pierce, E. Pittermann, N. Ma, T. Gebauer, A. T. Neffe, M. Hölscher, F. Jung and A. Lendlein, Macromol. Biosci., 2012, 12, 312–321 CrossRef CAS.
- S. Pahoff, C. Meinert, O. Bas, L. Nguyen, T. J. Klein and D. W. Hutmacher, J. Mater. Chem. B, 2019, 7, 1761–1772 RSC.
- Y. Xu, J. Peng, G. Richards, S. Lu and D. Eglin, J. Orthop. Translat., 2019, 18, 128–141 CrossRef PubMed.
- K. R. Stevens, J. S. Miller, B. L. Blakely, C. S. Chen and S. N. Bhatia, J. Biomed. Mater. Res., Part A, 2015, 103, 3331–3338 CrossRef CAS PubMed.
- C. D. Spicer, Polym. Chem., 2020, 11, 184–219 RSC.
- C. Fiedler, E. Aisenbrey, J. Wahlquist, C. Heveran, V. Ferguson, S. Bryant and R. McLeod, Soft Matter, 2016, 12, 9095–9104 RSC.
- S. J. Bryant and K. S. Anseth, J. Biomed. Mater. Res., Part A, 2003, 64, 70–79 CrossRef PubMed.
- Y. D. Taghipour, V. R. Hokmabad, D. Bakhshayesh, A. Rahmani, N. Asadi, R. Salehi and H. T. Nasrabadi, Curr. Med. Chem., 2020, 27(16), 2658–2680 CrossRef CAS PubMed.
- A. Kerin, M. Wisnom and M. Adams, Proc. Inst. Mech. Eng., Part H, 1998, 212, 273–280 CrossRef CAS PubMed.
- C. Tang, B. D. Holt, Z. M. Wright, A. M. Arnold, A. C. Moy and S. A. Sydlik, J. Mater. Chem. B, 2019, 7, 2442–2453 RSC.
- W. Schuurman, P. A. Levett, M. W. Pot, P. R. van Weeren, W. J. Dhert, D. W. Hutmacher, F. P. Melchels, T. J. Klein and J. Malda, Macromol. Biosci., 2013, 13, 551–561 CrossRef CAS PubMed.
- P. Smeriglio, J. H. Lai, F. Yang and N. Bhutani, J. Visualized Exp., 2015, 104, e53085 Search PubMed.
- D. Zhu, P. Trinh, E. Liu and F. Yang, ACS Biomater. Sci. Eng., 2018, 4, 3561–3569 CrossRef CAS PubMed.
- L. H. Nguyen, A. K. Kudva, N. S. Saxena and K. Roy, Biomaterials, 2011, 32, 6946–6952 CrossRef CAS PubMed.
- I. Strehin, Z. Nahas, K. Arora, T. Nguyen and J. Elisseeff, Biomaterials, 2010, 31, 2788–2797 CrossRef CAS PubMed.
- S. Varghese, N. S. Hwang, A. C. Canver, P. Theprungsirikul, D. W. Lin and J. Elisseeff, Matrix Biol., 2008, 27, 12–21 CrossRef CAS.
- X. Zhao, A. Papadopoulos, S. Ibusuki, D. A. Bichara, D. B. Saris, J. Malda, K. S. Anseth, T. J. Gill and M. A. Randolph, BMC Musculoskeletal Disord., 2016, 17, 245 CrossRef PubMed.
- F. Yu, X. Cao, Y. Li, L. Zeng, B. Yuan and X. Chen, Polym. Chem., 2013, 5, 1082–1090 RSC.
- G. Gao, A. F. Schilling, K. Hubbell, T. Yonezawa, D. Truong, Y. Hong, G. Dai and X. Cui, Biotechnol. Lett., 2015, 37, 2349–2355 CrossRef CAS PubMed.
- S. Bang, U.-W. Jung and I. Noh, Tissue Eng. Regener. Med., 2018, 15, 25–35 CrossRef CAS PubMed.
- C. Gegg and F. Yang, Acta Biomater., 2020, 101, 196–205 CrossRef CAS PubMed.
- H. Lin, A. M. Beck, K. Shimomura, J. Sohn, M. R. Fritch, Y. Deng, E. J. Kilroy, Y. Tang, P. G. Alexander and R. S. Tuan, J. Tissue Eng. Regener. Med., 2019, 13, 1418–1429 CrossRef CAS PubMed.
- Y. Xu, Z. Wang, Y. Hua, X. Zhu, Y. Wang, L. Duan, L. Zhu, G. Jiang, H. Xia and Y. She, Mater. Sci. Eng., C, 2020, 111628 Search PubMed.
- V. Moulisová, S. Poveda-Reyes, E. Sanmartín-Masiá, L. Quintanilla-Sierra, M. Salmerón-Sánchez and G. Gallego Ferrer, ACS Omega, 2017, 2, 7609–7620 CrossRef PubMed.
- X. Jiang, J. Liu, Q. Liu, Z. Lu, L. Zheng, J. Zhao and X. Zhang, Biomater. Sci., 2018, 6, 1616–1626 RSC.
- L. Zhang, K. Li, W. Xiao, L. Zheng, Y. Xiao, H. Fan and X. Zhang, Carbohydr. Polym., 2011, 84, 118–125 CrossRef CAS.
- F. Yu, X. Cao, L. Zeng, Q. Zhang and X. Chen, Carbohydr. Polym., 2013, 97, 188–195 CrossRef CAS PubMed.
- X. Hu, D. Li, F. Zhou and C. Gao, Acta Biomater., 2011, 7, 1618–1626 CrossRef CAS PubMed.
- P. A. Levett, F. P. Melchels, K. Schrobback, D. W. Hutmacher, J. Malda and T. J. Klein, Acta Biomater., 2014, 10, 214–223 CrossRef CAS PubMed.
- A. Vedadghavami, F. Minooei, M. H. Mohammadi, S. Khetani, A. R. Kolahchi, S. Mashayekhan and A. Sanati-Nezhad, Acta Biomater., 2017, 62, 42–63 CrossRef CAS PubMed.
- M. W. Tibbitt and K. S. Anseth, Biotechnol. Bioeng., 2009, 103, 655–663 CrossRef CAS PubMed.
- Q. T. Nguyen, Y. Hwang, A. C. Chen, S. Varghese and R. L. Sah, Biomaterials, 2012, 33, 6682–6690 CrossRef CAS PubMed.
- J.-H. Kim, G. Lee, Y. Won, M. Lee, J.-S. Kwak, C.-H. Chun and J.-S. Chun, Proc. Natl. Acad. Sci. U. S. A., 2015, 112, 9424–9429 CrossRef CAS PubMed.
- B. V. Sridhar, J. L. Brock, J. S. Silver, J. L. Leight, M. A. Randolph and K. S. Anseth, Adv. Healthcare Mater., 2015, 4, 702–713 CrossRef CAS PubMed.
- D. L. Butler, S. A. Goldstein and F. Guilak, J. Biomech. Eng., 2000, 122, 570–575 CrossRef CAS PubMed.
- S. Pettersson, J. Wetterö, P. Tengvall and G. Kratz, J. Tissue Eng. Regener. Med., 2009, 3, 450–460 CrossRef CAS PubMed.
- J. Samuelsen, H. Kopperud, J. Holme, I. Dragland, T. Christensen and J. Dahl, J. Biomed. Mater. Res., Part A, 2011, 96, 395–401 CrossRef CAS PubMed.
- H. Schweikl, G. Spagnuolo and G. Schmalz, J. Dent. Res., 2006, 85, 870–877 CrossRef CAS PubMed.
- P. D. Drumheller and J. A. Hubbell, J. Biomed. Mater. Res., 1995, 29, 207–215 CrossRef CAS PubMed.
- E. Tziampazis, J. Kohn and P. V. Moghe, Biomaterials, 2000, 21, 511–520 CrossRef CAS.
- C.-S. Ko, J.-P. Huang, C.-W. Huang and I.-M. Chu, J. Biosci. Bioeng., 2009, 107, 177–182 CrossRef CAS PubMed.
- J. A. Iocono, T. M. Krummel, K. A. Keefer, G. M. Allison and H. P. Ehrlich, Wound Repair Regen., 1998, 6, 442–448 CrossRef CAS PubMed.
- S. J. Bryant, K. S. Anseth, D. A. Lee and D. L. Bader, J. Orthop. Res., 2004, 22, 1143–1149 CrossRef CAS PubMed.
- B. Balakrishnan and R. Banerjee, Chem. Rev., 2011, 111, 4453–4474 CrossRef CAS PubMed.
- S.-H. Lee and H. Shin, Adv. Drug Delivery Rev., 2007, 59, 339–359 CrossRef CAS.
- E.-Y. Chuang, C.-W. Chiang, P.-C. Wong and C.-H. Chen, Adv. Mater. Sci. Eng., 2018, 2018, 4368910 Search PubMed.
- L. Fu, Z. Yang, C. Gao, H. Li, Z. Yuan, F. Wang, X. Sui, S. Liu and Q. Guo, Regener. Biomater., 2020, 7(6), 527–542 CrossRef CAS PubMed.
- R. Longley, A. M. Ferreira and P. Gentile, Int. J. Mol. Sci., 2018, 19, 1755 CrossRef PubMed.
- D. Gan, Z. Wang, C. Xie, X. Wang, W. Xing, X. Ge, H. Yuan, K. Wang, H. Tan and X. Lu, Adv. Healthcare Mater., 2019, 8, 1901103 CrossRef CAS PubMed.
- J. Liao, T. Tian, S. Shi, X. Xie, Q. Ma, G. Li and Y. Lin, Bone Res., 2017, 5, 1–15 Search PubMed.
- H. Xia, D. Zhao, H. Zhu, Y. Hua, K. Xiao, Y. Xu, Y. Liu, W. Chen, Y. Liu and W. Zhang, ACS Appl. Mater. Interfaces, 2018, 10, 31704–31715 CrossRef CAS.
- J. Xiongfa, Z. Hao, Z. Liming and X. Jun, Regener. Med., 2018, 13, 73–87 CrossRef PubMed.
- A. C. Daly, F. E. Freeman, T. Gonzalez-Fernandez, S. E. Critchley, J. Nulty and D. J. Kelly, Adv. Healthcare Mater., 2017, 6, 1700298 CrossRef PubMed.
- Y. Wu, P. Kennedy, N. Bonazza, Y. Yu, A. Dhawan and I. Ozbolat, Cartilage, 2018, 1947603518809410 Search PubMed.
- Z. Zhao, Y. Li, M. Wang, S. Zhao, Z. Zhao and J. Fang, J. Cell. Mol. Med., 2020, 24, 5408–5419 CrossRef CAS PubMed.
- G. Hautier, A. Jain and S. P. Ong, J. Mater. Sci., 2012, 47, 7317–7340 CrossRef CAS.
- A. A. Zadpoor, J. Mech. Behav. Biomed. Mater., 2017, 70, 1–6 CrossRef CAS PubMed.
- O. Bas, S. Lucarotti, D. D. Angella, N. J. Castro, C. Meinert, F. M. Wunner, E. Rank, G. Vozzi, T. J. Klein and I. Catelas, Chem. Eng. J., 2018, 340, 15–23 CrossRef CAS.
- S. L. Sridhar, M. C. Schneider, S. Chu, G. De Roucy, S. J. Bryant and F. J. Vernerey, Soft Matter, 2017, 13, 4841–4855 RSC.
- A. T. Metters, C. N. Bowman and K. S. Anseth, J. Phys. Chem. B, 2000, 104, 7043–7049 CrossRef CAS.
- U. Akalp, S. J. Bryant and F. J. Vernerey, Soft Matter, 2016, 12, 7505–7520 RSC.
- M. E. Stender, R. D. Carpenter, R. A. Regueiro and V. L. Ferguson, J. Biomech., 2016, 49, 3502–3508 CrossRef PubMed.
- S. J. Bryant and F. J. Vernerey, Adv. Healthcare Mater., 2018, 7, 1700605 CrossRef PubMed.
- E. Hanna, C. Rémuzat, P. Auquier and M. Toumi, Value Health, 2016, 19, A487 Search PubMed.
- P. C. Kreuz, R. H. Kalkreuth, P. Niemeyer, M. Uhl and C. Erggelet, Cartilage, 2019, 10, 305–313 CrossRef PubMed.
- X. Armoiry, E. Cummins, M. Connock, A. Metcalfe, P. Royle, R. Johnston, J. Rodrigues, N. Waugh and H. Mistry, PharmacoEconomics, 2019, 37, 879–886 CrossRef PubMed.
- W. Zhang, H. Ouyang, C. R. Dass and J. Xu, Bone Res., 2016, 4, 1–14 Search PubMed.
- H. Mistry, M. Connock, J. Pink, D. Shyangdan, C. Clar, P. Royle, R. Court, L. C. Biant, A. Metcalfe and N. Waugh, Health Technol. Assess., 2017, 21(6) DOI:10.3310/hta21060.
- J. Takamura, T. Seo and V. Strand, Cartilage, 2019, 10, 417–422 CrossRef PubMed.
- O. De Lucia, J. Jerosch, S. Yoon, T. Sayre, W. Ngai and G. Filippou, Clin. Rheumatol., 2020, 1–10 Search PubMed.
- A. Clavé, J. F. Potel, E. Servien, P. Neyret, F. Dubrana and E. Stindel, J. Orthop. Res., 2016, 34, 658–665 CrossRef PubMed.
- B. J. Huang, J. C. Hu and K. A. Athanasiou, Biomaterials, 2016, 98, 1–22 CrossRef CAS PubMed.
Footnote |
† These authors have equally contributed to this work. |
|
This journal is © The Royal Society of Chemistry 2021 |