DOI:
10.1039/D3SC02707D
(Edge Article)
Chem. Sci., 2024,
15, 9087-9095
Facile synthesis and characterization of aza-bridged all-benzenoid quinoidal figure-eight and cage molecules†
Received
29th May 2023
, Accepted 27th April 2024
First published on 31st May 2024
Abstract
Synthesis of conjugated compounds with unusual shape-persistent structures remains a challenge. Herein, utilizing thermodynamically reversible intermolecular Friedel–Crafts alkylation, a dynamic covalent chemistry (DCC) reaction, we facilely synthesized a figure-eight shaped macrocycle FEM and cage molecules CATPA/CACz. X-ray crystallographic analysis confirmed the chemical geometries of tetracation FEM4+(PF6−)4 and hexacation CACz6+(SbF6−)6. FEM and CATPA displayed higher photoluminescence quantum yield in solid states compared to that in solution, whereas CACz gave the reverse result. DFT calculations showed that fluorescence-related frontier molecular orbital profiles are mainly localized on their arms consisting of a p-quinodimethane (p-QDM) unit and two benzene rings of triphenylamine or carbazole. Owing to their space-confined structures, variable-temperature 1H NMR measurements showed that FEM, CATPA and FEM4+ have intramolecular restricted motion of phenyl rings on their chromophore arms. Accordingly, FEM and CATPA with flexible triphenylamine subunits displayed aggregation-induced emission behavior (AIE), whereas CACz with a rigid carbazole subunits structure showed no AIE behavior.
Introduction
Conjugated nanostructures such as fullerenes, carbon nanotubes and graphene have had revolutionary influence in the areas of chemistry, physics and materials.1 Recently, novel unusual all-benzenoid shape-persistent structures have attracted scientists' attention, such as carbon nanohoops,2 nanobelts,3 extended helicenes,4 figure-eight4c–e,5 and bow-knot molecules,6 molecular cages,7 catenane and trefoil knots,8 and open-shell nanographenes.9 Conversely, methine- or heteroatom-bridged all-benzenoid conjugated nanostructures have been developed, which represent another class of unusual conjugated structures.10 Study of these materials could elaborate basic chemical concepts such as global aromaticity10f,g and bond tautomerization10f owing to their alternative quinoidal and aromatic structures. Besides, these novel shape-persistent structures are also endowed with novel physical properties, such as supramolecular behavior owing to their specified cavity10h,i or interesting photophysical and optoelectronic properties resulting from the introduction of heteroatoms.10d,e
The synthesis of conjugated shape-persistent structures has always been challenging. Kinetically controlled C–C bond reactions for the synthesis of conjugated nanostrucutres usually need expensive metal catalysts or tedious synthetic steps.2–10
Dynamic covalent chemistry (DCC) refers to chemical reactions performed reversibly under equilibrium-controlled conditions with self-rectifying character.11 Friedel–Crafts alkylation is a thermodynamically controlled DCC reaction12 that has been utilized for the synthesis of macrocyclic compounds. The DCC method also has potential for application in the design and synthesis of more complex and challenging unusual shape-persistent structures. Herein, we report an efficient approach to synthesize figure-eight (FEM) and cage (CATPA and CACz) molecules via a metal-free Friedel–Crafts alkylation as the key step (Fig. 1). The FEM molecule contains a space-confined pentagon-like geometry on each half. Three-armed CATPA shows a flexible conjugated structure, whereas CACz exhibits a more rigid structure. Their photophysical properties, redox properties, and molecular dynamics in neutral and cationic states were investigated. Furthermore, these structure-confined molecules provided us good templates to investigate their aggregation-induced emission (AIE) behaviour.
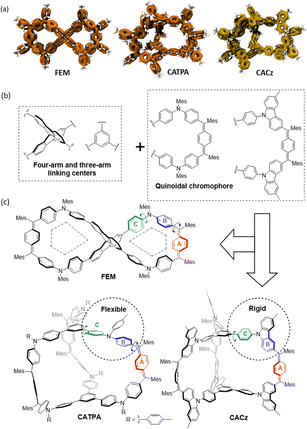 |
| Fig. 1 (a) Localized orbital locator (LOL)-π isosurface maps, (b) building blocks and (c) chemical structures of figure-eight shape (FEM) and cage (CATPA and CACz) molecules. | |
Results and discussion
Synthesis
All compounds were synthesized by intermolecular Friedel–Crafts alkylation followed by oxidative dehydrogenation reaction (Scheme 1). The intermediate 3 was first prepared via a four-fold Suzuki coupling reaction between 1 and 2 in 83% yield. Then, BF3·Et2O mediated the two-fold intermolecular Friedel–Crafts alkylation between 3 and diol 4 in a dilute dichloromethane (DCM) solution to give the figure-eight macrocycle precursor 5. It was subsequently purified by flash column chromatography and further separated by preparative GPC, yielding 31%. FEM was obtained in 54% yield by oxidative dehydrogenation of 5 with 2.2 equivalents of 2,3-dichloro-5,6-dicyano-1,4-benzoquinone (DDQ). Similarly, to synthesize cage compounds CATPA and CACz, intermediates 9/10 were synthesized by three-fold Suzuki coupling reaction between 1,3,5-benzenetriboronic acid tris(pinacol) ester 6 and 7/8 in yields of 73%/63%. Subsequent intermolecular Friedel–Crafts cyclization between 9 and diol 4 afforded 11 in 23% yield, and further oxidative dehydrogenation of 11 afforded the target compound CATPA in 46% yield. However, intermolecular Friedel–Crafts cyclization between 10 and diol 4 afforded 12 with a yield of around 5%. The relatively low yield of 12 could probably be ascribed to the low reactivity of 10 towards electrophilic cations. Further oxidative dehydrogenation of 12 gave an insoluble yellow solid, and CACz could be obtained by recrystallization from toluene with 20% yield. Limited by its poor solubility in common deuterated solvents, 1H NMR measurement of CACz did not show any peaks even in hot toluene-d8; however, the structure of CACz6+(SbF6−)6 could be identified by its 1H NMR spectra (Fig. S47 in ESI†) and X-ray crystallographic analysis. The structures of FEM and CATPA were identified by 1H and 13C NMR measurements in C6D6 (Fig. S30, S31, and S39 in ESI†), and all the protons were assigned by 2D ROESY NMR spectra (Fig. S48 and S51 in ESI†). It is worth noting that FEM is racemic with two enantiomers (P and M), which give identical NMR spectra. However, they could not be resolved after some attempts.
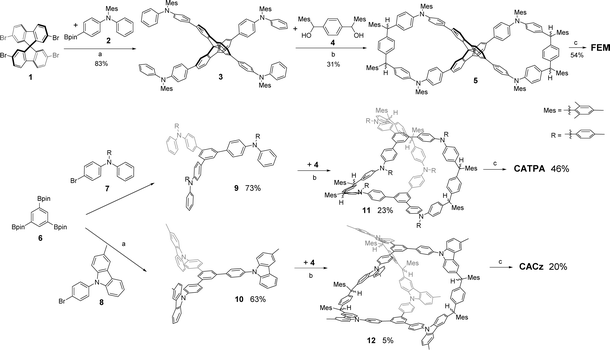 |
| Scheme 1 Synthetic routes for FEM, CATPA and CACz. (a) Pd(PPh3)4, Na2CO3, ethanol, and H2O. (b) BF3·Et2O and DCM. (c) DDQ, toluene, and acetonitrile. DCM = dichloromethane; DDQ = 2,3-dichloro-5,6-dicyano-1,4-benzoquinone; and Mes: mesityl. | |
Photophysical properties
UV-vis absorption spectra for FEM, CATPA and CACz were recorded in THF solution (Fig. 2 and Table 1). FEM, CATPA and CACz all show two intense absorption bands centered at 384/490 nm, 358/472 nm and 310/466 nm, respectively (Fig. 2a). DFT calculations indicated that the long-wavelength absorption band of FEM is correlated to the synergistic contribution of HOMO → LUMO and HOMO−1 → LUMO+1 electronic transitions (Fig. S12a and Table S3 in ESI†). Similarly, the long-wavelength absorption bands of CATPA and CACz are correlated to the multiple electronic transitions of HOMO−n to LUMO+m (n, m = 0, 1 and 2). (Fig. S12b, c, Tables S4 and S5 in ESI†). Furthermore, these frontier molecular orbitals are mainly localized along the two half-arms of FEM or one-third arms of CATPA and CACz consisting of the p-QDM ring A and two benzene rings B of triphenylamine or carbazole, respectively (Fig. S11 in ESI†). The absorption spectrum of CACz exhibits a hypsochromic shift, probably due to a weak donor–acceptor effect between carbazoles and p-QDM units. In the thin-film state, FEM and CACz show similar absorption bands and wavelength of absorption maxima to those in solution (Fig. 2a and c), indicating that there is no obvious intermolecular electronic coupling between neighbouring molecules. The wavelength of the absorption maximum of CATPA in the thin-film state exhibits a 34 nm red shift, indicating possible intermolecular electronic coupling between adjacent molecules.
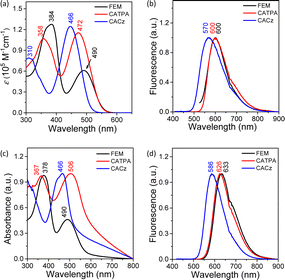 |
| Fig. 2 (a) UV-vis absorption spectra and (b) fluorescence spectra for FEM, CATPA and CACz in THF solution with an excitation wavelength (λex) at 365, 395 and 400, respectively; (c) UV-vis absorption spectra and (d) fluorescence spectra for FEM, CATPA and CACz in the thin-film state (λex = 400 nm for all three compounds). | |
Table 1 Photophysical data of FEM, CATPA and CACz
|
Solution |
Film |
λ
abs
(nm) |
ε
max
(105 M−1 cm−1) |
λ
em
(nm) |
Φ
F
(%) |
τ
(ns) |
k
r
(s−1) |
k
nr
(s−1) |
λ
abs (nm) |
λ
em (nm) |
Φ
F (%) |
τ (ns) |
k
r (s−1) |
k
nr (s−1) |
λ
abs: absorption maximum wavelength.
ε
max: molar extinction coefficient (M−1 cm−1) at the wavelength of the absorption maximum in the low-energy absorption band.
λ
em: emission maximum wavelength.
Φ: photoluminescence quantum yield.
τ: fluorescence lifetimes were measured with time-correlated single-photon counting operation mod.
k
r: radiative rate constants were calculated with kr = ΦF/τ.
k
nr: nonradiative rate constants were calculated with knr = (1 − ΦF)/τ.
|
FEM
|
384, 490 |
0.61 |
600 |
1.0 |
1.73 |
5.8 × 106 |
5.7 × 108 |
378, 490 |
633 |
6.2 |
1.54 |
4.0 × 107 |
6.1 × 108 |
CATPA
|
358, 472 |
1.15 |
600 |
1.2 |
1.60 |
7.5 × 106 |
6.2 × 108 |
367, 506 |
626 |
4.8 |
1.40 |
3.4 × 107 |
6.8 × 108 |
CACz
|
310, 466 |
1.24 |
570 |
7.4 |
0.63 |
1.2 × 108 |
1.5 × 109 |
466 |
586 |
4.8 |
2.35 |
2.0 × 107 |
4.1 × 108 |
The fluorescence spectra of FEM, CATPA and CACz in THF solution are shown in Fig. 2b. FEM exhibits one emission peak at 600 nm with a photoluminescence quantum yield (PLQY) of 1.0%. DFT calculations indicated that this emission band is mainly correlated to a synergistic contribution of LUMO+1 → HOMO and LUMO → HOMO−1 radiative transitions (Fig. S12a and Table S6 in ESI†). CATPA and CACz show one emission peak at 600 and 570 nm with PLQY of 1.2% and 7.4%, respectively. The radiative transitions of CATPA and CACz are contributed by the multiple electronic transitions of LUMO+m to HOMO−n (n, m = 0, 1 and 2) (Fig. S12b, c, Tables S7 and S8 in ESI†). The Stokes shifts of FEM, CATPA and CACz in THF solution are 110 nm, 128 nm and 104 nm, respectively, indicating the flexible structure of CATPA. Furthermore, the fluorescence-related frontier molecular orbital profiles of three compounds are also localized along the arm consisting of rings A and B (Fig. S11 in ESI†). Therefore, the intramolecular dynamic motions of these moieties would have a significant influence on their fluorescence properties.
The fluorescence spectra of FEM, CATPA and CACz in the solid state exhibit a red-shifted emission band with maxima at 633, 626 and 586 nm, respectively (Fig. 2d). In the solid state, FEM and CATPA show 6- and 4-fold enhanced PLQYs of 6.2% and 4.8%, respectively. Conversely, CACz exhibits a decreased PLQY of 4.8% in the solid state. Fluorescence dynamics indicated that, from solution to solid, the enhanced PLQYs of FEM and CATPA could be explained by about 7-and 4-times increased radiative rate constants, kr, from 5.8 × 106 s−1 and 7.5 × 106 s−1 to 4.0 × 107 s−1 and 3.4 × 107 s−1, respectively. Meanwhile, non-radiative rate constants, knr, of FEM and CATPA only show a slight increase (Table 1), whereas the kr of CACz (1.2 × 108 s−1) in solution is 16 to 20 times higher than those of FEM and CATPA. From solution to solid state, the kr of CACz decreases by about 6-fold to 2.0 × 107 s−1, and the knr of CACz also decreases from 1.5 × 109 s−1 to 4.1 × 108 s−1.
These phenomena could be ascribed to the following: (i) in the solution, the vibration of quinoidal ring A and free rotation of the phenyl ring B (Fig. 1) in FEM and CATPA would release some energy from their excited states and consequently result in low PLQYs; (ii) in the solid state, the motions of ring A and ring B in FEM and CATPA are restricted, causing their increased PLQYs; and (iii) due to the rigid chromophores of CACz, more energy of the excited states could be released in a radiative way, consequently causing its moderate PLQYs in solution and film state.
Electrochemical properties and chemical oxidation
FEM and CATPA display only one reversible oxidation wave with half-wave potential, E1/2ox, at −0.23 V and −0.14 V (vs. Fc+/Fc couple) and irreversible reduction wave with half-wave potential, E1/2red, at −2.36 V, −2.33 V, respectively (Fig. S6a, b and Table S2 in ESI†). CACz in 1,2-dichlorobenzene at 80 °C displays only a reversible oxidation wave with E1/2ox at 0.00 V, and no reduction wave could be observed (Fig. S6c in ESI†).
The dication FEM2(˙+)(SbF6−)2 and triradical trication CATPA3(˙+)(SbF6−)3/CACz3(˙+)(SbF6−)3 were generated in dry DCM by adding two and three equivalents of silver hexafluoroantimonate (AgSbF6) to their neutral compounds, and they showed intense ESR signals in solution with g values of 2.0027 and 2.0028/2.0030 (Fig. S3†), respectively. Additionally, FEM2(˙+)(SbF6−)2, CATPA3(˙+)(SbF6−)3 and CACz3(˙+)(SbF6−)3 exhibit similar broad long-wavelength absorption bands with λmax at 1000/1183, 990/1169 and 919/1072 nm, respectively (Fig. 3, S7a, S8a and S9a†). DFT calculations revealed that FEM2(˙+)(SbF6−)2 and CATPA3(˙+)(SbF6−)3/CACz3(˙+)(SbF6−)3 contain two or three separated mono radicals with spin densities located at each quinoidal arm, respectively (Fig. S10†). The tetracation FEM4+(SbF6−)4 and hexacations CATPA6+(SbF6−)6/CACz6+(SbF6−)6 were generated by chemical oxidation with four and six equivalents of AgSbF6, respectively. These tetra- and hexacations are all ESR silent and show clear 1H NMR spectra at 298 K (Fig. S32, S40 and S47†), indicating their closed-shell character. The protons of FEM4+(SbF6−)4 and CATPA6+(SbF6−)6 are well assigned by 2D ROESY NMR (Fig. S49, S50 and S52†). UV-Vis-NIR absorption spectra for FEM4+(SbF6−)4 display two major absorption bands with λmax at 344/511 nm. UV-Vis-NIR absorption spectra for CATPA6+(SbF6−)6 and CACz6+(SbF6−)6 show a main peak at 505 and 419 nm with a shoulder band at 600 and 500 nm extended to the near-infrared region, respectively.
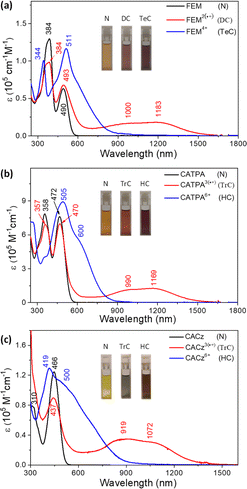 |
| Fig. 3 (a) UV-Vis-NIR absorption spectra for FEM, FEM2(˙+) and FEM4+ generated by chemical titration with AgSbF6. (b) UV-vis-NIR absorption spectra for CATPA, CATPA3(˙+) and CATPA6+. (c) UV-vis-NIR absorption spectra for CACz, CACz3(˙+) and CACz6+ (insets are their photographs in DCM). N: neutral; DC: dication; TrC: trication; TeC: tetracation; HC: hexacation. | |
Intramolecular dynamic motions
The space-confined structures of the figure-eight and cage molecules provoked us to investigate their molecular dynamics. Variable-temperature (VT) 1H NMR spectra for FEM in toluene-d8 (Fig. 4a) show that, upon cooling, protons k of mesityl groups on N atoms split into two singlet peaks (k and k′) with coalescence temperature (Tc) at 343 K, indicating the slowed-down rotation of the mesityl groups. From 363 K to 203 K, protons c and d on ring B also gradually become broad and fully coalesce at 213 K. Limited by the solubility of FEM in toluene, all the other peaks in the aromatic region become broad upon further cooling. Similar to other macrocyclic skeletons,13 this phenomenon can be explained by the flipping ceasing process of B rings at low temperature, which caused the low-field movement of proton b with a Δδ of 0.44 ppm. DFT calculated the strain energy of FEMvia hypothetical homodesmotic reactions14 (Scheme S1 in ESI†) to be −9.0 kcal mol−1, which is much smaller than those of all-benzenoid aromatic nanohoops and nanobelts5a,b,15 for the introduction of bridged atoms.
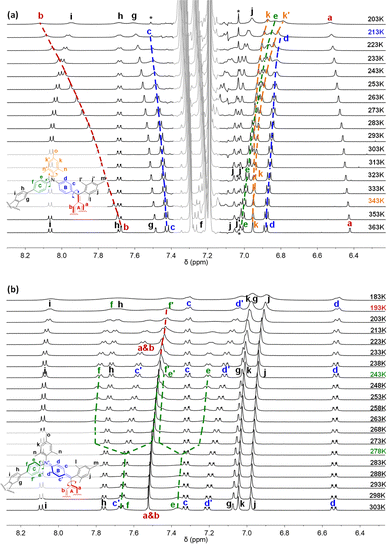 |
| Fig. 4 VT 1H NMR spectra (aromatic region) for (a) FEM and (b) FEM4+(SbF6−)4 in toluene-d8. Dashed lines showed the changes of protons on the A (red), B (blue) and C (green) rings with temperature. * indicates the residue from toluene-d8. One quarter of the chemical structure of FEM and FEM4+ is shown. | |
VT 1H NMR spectra for FEM4+(SbF6−)4 in CD2Cl2 are shown in Fig. 4b. At 298 K, protons c and d on the B rings split into two sets of signals for the formation of localized N+-doped p-QDMs, accompanied by the formation of benzenoid A rings with two protons a and b merging into one a & b peak. Upon cooling, the flipping of C rings slows down, and the resonances of protons e and f coalesce at 278 K and finally split into two sets of signals e/e′ and f/f′ at 243 K. Upon further cooling, protons a & b also gradually coalesce at 193 K. The exchange rate constants (k) of the protons on the C rings at different temperatures were obtained by line shape analysis (Section 2 in the ESI†), and fitting of the data by the Eyring equation (ln(k/T) = −(ΔH‡/R)·1/T + ln(kB/h) + ΔS‡/R) gave thermodynamic parameters ΔH‡ = 22.1 ± 0.5 kcal mol−1 and ΔS‡ = 32.8 ± 1.7 cal (mol−1 K−1) (Table S1 and Fig. S1 in ESI†). Accordingly, the interconversion energy ΔG‡ of the C rings at 243 K was estimated to be 13.0 ± 0.9 kcal mol−1. This can be explained by its more confined structure induced by two newly formed N+-doped p-QDMs and the corresponding Coulomb repulsion between two positively charges. Therefore, DFT calculations gave a larger strain energy of −24.7 kcal mol−1 (Scheme S1 in ESI†).
VT 1H NMR spectra for CATPA in toluene-d8 (Fig. S4 in ESI†) show that p-tolyl groups on N atoms can freely rotate even upon cooling due to its lower steric hindrance. Protons c and d on ring B gradually become broad, and fully coalesce around 208 K. Proton b also shows low-field movement upon cooling; however, the small Δδ of 0.17 ppm indicates the weak deshielding effect from the B rings. This could originate from its small strain energy of −7.3 kcal mol−1 (Scheme S1 in ESI†). In VT 1H NMR spectra for CATPA6+(SbF6−)6 (Fig. S5†), upon cooling, protons (c, c′, d and d′) on the ring B show slight high-field movement, which could be ascribed to the weak shielding effect of the A and C rings.
Ground-state geometry
The geometry of FEM (M-enantiomer) was optimized by DFT calculations (Fig. 5a). The dihedral angles of the rings A, B and C with respect to the plane of each half, determined using the sp3 C atom on spirofluorene (SBF) and two meso C atoms, are 13.4°, 26.9°/33.4°, and 44.1°/43.0°, respectively. Obviously, the A rings are less distorted than the B and C rings due to the quinoidal character of the A rings. In each half-cycle, five phenyl rings show alternating up-down conformation, which can help to release the strain energy caused by the steric repulsion.
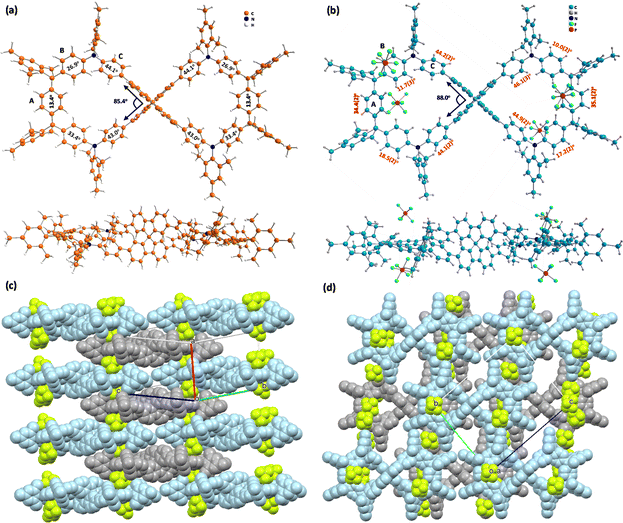 |
| Fig. 5 (a) DFT optimized structure of FEM (M-enantiomer) and (b) X-ray crystallographic structure of FEM4+(PF6−)4 with top and side views (M-enantiomer was shown only). (c and d) Packing motifs of FEM4+(PF6−)4 were shown in space filling model with side and top view. P-Enantiomers are shown in light blue colour, and M-enantiomers are shown in light grey colour; counter anions are shown in light green colour. Solvent molecules are omitted. | |
FEM4+(PF6−)4 was obtained by the chemical oxidation of FEM with four equivalents of silver hexafluorophosphate (AgPF6). The 1H NMR spectrum of FEM4+(PF6−)4 (Fig. S34 in ESI†) is almost identical to that of FEM4+(SbF6−)4. Single-crystal FEM4+(PF6−)4 (ref. 16) (Fig. 5b–d) crystalizes in the P
group, and the four counter anions PF6− are located well above and below the center of each half-cycle (Fig. 5b). The angle between the two phenyl rings of SBF in one half-cycle is 88.0°. Similarly, the phenyl rings in each half also show alternating up-down conformation. In its packing structure (Fig. 5c and d), the P-enantiomer (light blue) and M-enantiomer (grey) are arranged in a three-dimensional (3D) superstructure. Along the a axis (Fig. 5c and S16a in ESI†), P-enantiomers and M-enantiomers are overlapped alternatingly with their half of the molecules, forming a columnar structure. The top view of the packing along the bc plane is shown in Fig. 5d and 16b in the ESI.† This packing structure is stabilized by multiple [C–H⋯F] (2.294(6)–2.669(6) Å, Fig. S17a and Table S11 in ESI†) and [C⋯F] (3.048(10)/3.055(13)/3.164(11) Å, Fig. S17b and Table S12 in ESI†) and [C–H⋯C] (2.713(12)–2.896(12) Å, Fig. S17c and Table S13 in ESI†) interactions, which leads to the formation of a closely packed 3D network. For comparison, dihedral angles (Fig. 5b) of the A, B and C rings of FEM4+(PF6−)4 with respect to the plane of each half determined using the sp3 C atom on SBF and two meso C atoms are 34.4(2)°/35.1(2)°, 11.7(3)°/18.5(2)°/10.0(2)°/17.2(2)°, and 44.2(2)°/44.1(2)°/46.1(3)°/44.9(2)°, respectively. Compared to the neutral structure, the four B rings become less distorted than A rings and C rings due to their quinoidal structures.
Localized orbital locator (LOL) π-electrons analysis was performed based on wavefunction analysis code Multiwfn17 to investigate the π-conjugated pathway. The LOL-π isosurface maps of FEM and FEM4+ with an isovalue of 0.2 (Fig. 1 and S13 in ESI†) reveal that the π-electrons are delocalized along entire molecular backbones except for sp3 C, indicating a fully conjugated structure. For FEM and FEM4+, two twisted figure-eight π-electron pathways catenate together, and each one shows near-planar π-conjugation along two half-cycles at the same side of the molecular surface and radial π-conjugation2 along the central SBF unit (Fig. S13†). In addition, the calculated anisotropy of the induced current density (ACID)18 plots of FEM and FEM4+ (Fig. S15 in ESI†) show their quinoidal structures and localized aromatic phenyl rings. Similarly, CATPA, CATPA6+, CACz and CACz6+ are also fully conjugated cages with localized aromatic character (Fig. 1 and S14 in the ESI†).
Single-crystal CACz6+(SbF6−)6 (ref. 16) crystalizes in the P
2c group, with six SbF6− counter anions located around CACz6+, with twelve occupied positions owing to the random distribution of SbF6− (Fig. 6a and b). In its packing structure (Fig. 6c), a hexagonal lattice layer is formed with one CACz6+(SbF6−)6 molecule surrounded by six neighbour molecules in one layer. Three CACz6+(SbF6−)6 molecules form a triangle structure with one arm going to the concave position of adjacent molecules, which is also stabilized by the [C⋯F] (3.05(4) Å) interaction of SbF6− and carbons on ring A, multiple [C–H⋯F] (2.39(2)–2.55(2) Å) interactions of SbF6− and protons on the ring B, mesityl group and central phenyl ring (Fig. S18a and Table S14 in ESI†), [C⋯C] (3.22(3) Å) and multiple [C–H⋯C] interactions (2.79(2)–2.852(17) Å) between the ring B and neighbouring carbazoles (Fig. S18b and Table S15 in ESI†). Along the c axis, CACz6+(SbF6−)6 molecules arrange themselves in columnar fashion, with two neighbouring molecules featuring a rotation angle of 39.0(13)° and π–π overlap distance of 3.87(3) Å between the two central phenyl rings (Fig. 6d). Additionally, the rotation of two stacking molecules leads to three sets of carbazole moieties exhibiting π–π overlap distances of around 3.39(5) Å–3.57(2) Å, resulting in the formation of an interpenetrating column structure.
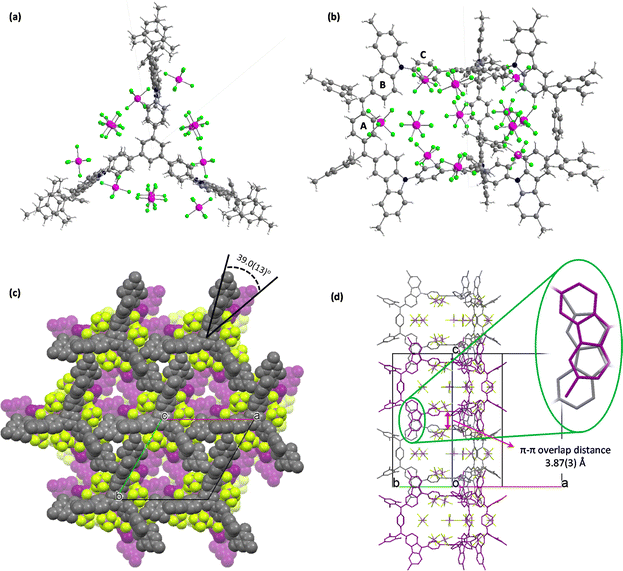 |
| Fig. 6 X-ray crystallographic structure of CACz6+(SbF6−)6 with (a) top view and (b) side view. (c and d) Packing motifs of CACz6+(SbF6−)6 are shown in a space filling model with top and side views. Two layers in alternative colours of grey and purple are shown, and counter anions are shown in light green colour. | |
Aggregation-induced emission
Considering the increased PLQYs of FEM/CATPA and decreased PLQY of CACz from solution to the solid state, photoluminescence measurements were conducted in THF/H2O mixed solvents with different water fractions to examine their potential AIE behavior.19 As shown in Fig. 7, by increasing the water fraction (fw) of the mixed solvents, the emission intensity of FEM and CATPA increased, accompanied by a slight blue shift of the wavelength of the emission maximum. Eventually, the ratio of I/I0 (I0 and I represent the emission intensity at the maximum emission wavelength when fw is 0% and corresponding water fractions, respectively) reached maximum saturation values of 38 and 23 for FEM and CATPA, respectively. Therefore, both compounds showed the typical phenomena of AIE fluorogens (AIEgens), whereas CACz did not show AIE behavior (Fig. S21†).
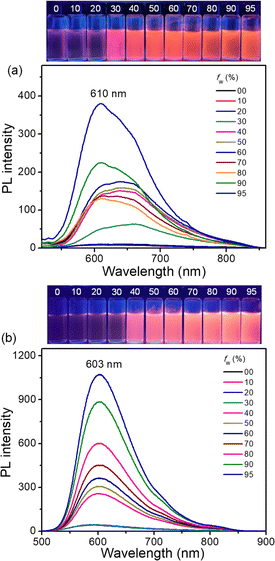 |
| Fig. 7 Photoluminescence (PL) spectra (1.5 × 10−5 m, λex = 400 nm) for (a) FEM and (b) CATPA in THF/H2O mixed solvents with different H2O fractions (fw) (insets are PL photographs of FEM and CATPA with different water fractions under 365 nm UV irradiation). | |
Molecular fluorescence dynamics indicate that the knr/kr ratios of FEM and CATPA decreased from 98 and 83 in solution to 15 and 20 in the solid state, respectively. Therefore, the weak emissions of FEM and CATPA in pure THF solution can be ascribed to the non-radiative energy consumption. Since their HOMO−n and LUMO+m (n, m = 0, 1 and 2) coefficients are mainly localized on quinoidal arms, their emission band can be significantly affected by the intramolecular motions of the A and B rings. The energy of the excited state would probably be released by free intramolecular rotations of the B rings or vibrations of p-QDM A units in the solution. With the increase of the water fraction, the formation of aggregates would limit the free motions of these rings. Therefore, the AIE phenomena of FEM and CATPA can be explained by the restriction of the intramolecular motion mechanism observed in other AIEgen systems.19 Our findings here provide a new class of quinoidal AIEgens with orange light emission.
Conclusions
Spirofluorene-bridged figure-eight shaped macrocycle FEM and 1,3,5-tribenzene-bridged cage molecules CATPA/CACz were facilely synthesized by intermolecular Friedel–Crafts alkylation followed by oxidative dehydrogenation. Four-electron and six-electron chemical oxidation produced their respective tetracation FEM4+ and hexacation CATPA6+/CACz6+. Single-crystal analysis of FEM4+ revealed its figure-eight geometry with a closely packed 3D network. Single-crystal analysis of CACz6+(SbF6−)6 revealed its cage geometry and tightly packed interpenetrating hexagonal lattice structure. VT-NMR measurements of FEM/CATPA and FEM4+ clearly revealed their unique temperature-dependent intramolecular dynamic motions, which originated from their space-confined chemical structures. This dynamic process has a significant impact on their emission properties from solution to aggregate or solid state, and AIE behavior was observed for FEM/CATPA, whereas CACz with a rigid conjugated structure showed no AIE behavior. The macrocyclic and cage-like structures reported in this work are also a new class of quinoidal AIEgens and are a good addition to the AIE family. Our dynamic covalent chemistry method provides an efficient approach that could be used to synthesize conjugated 2D or 3D architectures with different topologies and novel optoelectronic properties in the future.
Data availability
Experimental synthetic procedures, characterization data and theoretical calculation results are available in the ESI.†
Author contributions
S. Dong performed the synthetic and characterization work, J. Wang, A. Li, and Y. Zhang carried out spectroscopic measurements and data analysis, Y. Han and T. Y. Gopalakrishna conducted and analysed the computational results, H. Tian conducted the X-ray crystallographic analysis, S. Dong and C. Chi concepted the work and wrote the paper.
Conflicts of interest
There are no conflicts to declare.
Acknowledgements
The research at National University of Singapore was supported by MOE Tier 1 grant (A-8000992-00-00), MOE Tier 2 grants (MOE-MOET2EP10120-0006 and MOET2EP10222-0008) and A*STAR AME MTC YIRG project (M21K3c0128). The research in China was supported by Natural Science Foundation of China (22175128) and starting grants of Tianjin University.
References
-
(a) H. W. Kroto, J. R. Heath, S. C. O'Brien, R. F. Curl and R. E. Smalley, Nature, 1985, 318, 162 CrossRef CAS;
(b) S. Iijima, Nature, 1991, 354, 56 CrossRef CAS;
(c) K. S. Novoselov, A. K. Geim, S. V. Morozov, D. Jiang, Y. Zhang, S. V. Dubonos, I. V. Grigorieva and A. A. Firsov, Science, 2004, 306, 666 CrossRef CAS PubMed.
-
(a) S. Yamago, E. Kayahara and T. Iwamoto, Chem. Rec., 2014, 14, 84 CrossRef CAS PubMed;
(b) M. R. Golder and R. Jasti, Acc. Chem. Res., 2015, 48, 557 CrossRef CAS PubMed;
(c) Y. Segawa, A. Yagi, K. Matsui and K. Itami, Angew. Chem., Int. Ed., 2016, 55, 5136 CrossRef CAS PubMed.
-
(a) G. Povie, Y. Segawa, T. Nishihara, Y. Miyauchi and K. Itami, Science, 2017, 356, 172 CrossRef CAS;
(b) Z. Sun, K. Ikemoto, T. M. Fukunaga, T. Koretsune, R. Arita, S. Sato and H. Isobe, Science, 2019, 363, 151 CrossRef CAS PubMed;
(c) K. Y. Cheung, S. Gui, C. Deng, H. Liang, Z. Xia, Z. Liu, L. Chi and Q. Miao, Chem, 2019, 5, 838 CrossRef CAS;
(d) S. Nishigaki, Y. Shibata, A. Nakajima, H. Okajima, Y. Masumoto, T. Osawa, A. Muranaka, H. Sugiyama, A. Horikawa, H. Uekusa, H. Koshino, M. Uchiyama, A. Sakamoto and K. Tanaka, J. Am. Chem. Soc., 2019, 141, 14955 CrossRef CAS PubMed;
(e) T. Shi, Q. Guo, S. Tong and M. Wang, J. Am. Chem. Soc., 2020, 142, 4576 CrossRef CAS PubMed;
(f) H. Chen, S. Gui, Y. Zhang, Z. Liu and Q. Miao, CCS Chem., 2020, 3, 613 CrossRef;
(g) Y. Han, S. Dong, J. Shao, W. Fan and C. Chi, Angew. Chem., Int. Ed., 2021, 133, 2690 CrossRef;
(h) K. Y. Cheung, K. Watanabe, Y. Segawa and K. Itami, Nat. Chem., 2021, 13, 255 CrossRef CAS PubMed;
(i) Z. Xia, S. H. Pun, H. Chen and Q. Miao, Angew. Chem., Int. Ed., 2021, 60, 10311 CrossRef CAS PubMed;
(j) Y. Segawa, T. Watanabe, K. Yamanoue, M. Kuwayama, K. Watanabe, J. Pirillo, Y. Hijikata and K. Itami, Nat. Synth., 2022, 1, 535 CrossRef;
(k) H. Chen, Z. Xia and Q. Miao, Chem. Sci., 2022, 13, 2280 RSC.
-
(a) A. Robert, P. Dechambenoit, E. A. Hillard, H. Bock and F. Durola, Chem. Commun., 2017, 53, 11540 RSC;
(b) X. Jiang, J. D. Laffoon, D. Chen, S. Pérez-Estrada, A. S. Danis, J. Rodríguez-López, M. A. Garcia-Garibay, J. Zhu and J. S. Moore, J. Am. Chem. Soc., 2020, 142, 6493 CrossRef CAS PubMed;
(c) G. R. Kiel, K. L. Bay, A. E. Samkian, N. J. Schuster, J. B. Lin, R. C. Handford, C. Nuckolls, K. N. Houk and T. D. Tilley, J. Am. Chem. Soc., 2020, 142, 11084 CrossRef CAS PubMed;
(d) W. Fan, T. Matsuno, Y. Han, X. Wang, Q. Zhou, H. Isobe and J. Wu, J. Am. Chem. Soc., 2021, 143, 15924 CrossRef CAS PubMed;
(e) M. Krzeszewski, H. Ito and K. Itami, J. Am. Chem. Soc., 2022, 144, 862 CrossRef CAS PubMed.
-
(a) K. Senthilkumar, M. Kondratowicz, T. Lis, P. J. Chmielewski, J. Cybińska, J. L. Zafra, J. Casado, T. Vives, J. Crassous, L. Favereau and M. Stępień, J. Am. Chem. Soc., 2019, 141, 7421 CrossRef CAS PubMed;
(b) T. A. Schaub, E. A. Prantl, J. Kohn, M. Bursch, C. R. Marshall, E. J. Leonhardt, T. C. Lovell, L. N. Zakharov, C. K. Brozek, S. R. Waldvogel, S. Grimme and R. Jasti, J. Am. Chem. Soc., 2020, 142, 8763 CrossRef PubMed;
(c) L. Zhan, C. Dai, G. Zhang, J. Zhu, S. Zhang, H. Wang, Y. Zeng, C. Tung, Li. Wu and H. Cong, Angew. Chem., Int. Ed., 2022, 61, e202113334 CrossRef CAS.
-
(a) Z. Huang, C. Chen, X. Yang, X. Fan, W. Zhou, C. Tung, L. Wu and H. Cong, J. Am. Chem. Soc., 2016, 138, 11144 CrossRef CAS PubMed;
(b) W. Xu, X. Yang, X. Fan, X. Wang, C. Tung, L. Wu and H. Cong, Angew. Chem., Int. Ed., 2019, 58, 3943 CrossRef CAS PubMed;
(c) L. Wang, N. Hayase, H. Sugiyama, J. Nogami, H. Uekusa and K. Tanaka, Angew. Chem., Int. Ed., 2022, 59, 17951 CrossRef PubMed.
-
(a) E. Kayahara, T. Iwamoto, H. Takaya, T. Suzuki, M. Fujitsuka, T. Majima, N. Yasuda, N. Matsuyama, S. Seki and S. Yamago, Nat. Commun., 2013, 4, 2694 CrossRef PubMed;
(b) K. Matsui, Y. Segawa and K. Itami, J. Am. Chem. Soc., 2014, 136, 16452 CrossRef CAS PubMed;
(c) S. Cui, G. Zhuang, D. Lu, Q. Huang, H. Jia, Y. Wang, S. Yang and P. Du, Angew. Chem., Int. Ed., 2018, 130, 9330 CrossRef PubMed;
(d) M. Mastalerz, Acc. Chem. Res., 2018, 51, 2411 CrossRef CAS PubMed;
(e) N. Hayase, J. Nogami, Y. Shibata and K. Tanaka, Angew. Chem., Int. Ed., 2019, 58, 9439 CrossRef CAS PubMed;
(f) J. Zhu, Y. Han, Y. Ni, S. Wu, Q. Zhang, T. Jiao, Z. Li and J. Wu, J. Am. Chem. Soc., 2021, 143, 14314 CrossRef CAS PubMed.
-
(a) Y. Fan, D. Chen, Z. Huang, J. Zhu, C. Tung, L. Wu and H. Cong, Nat. Commun., 2018, 9, 3037 CrossRef PubMed;
(b) Y. Segawa, M. Kuwayama, Y. Hijikata, M. Fushimi, T. Nishihara, J. Pirillo, J. Shirasaki, N. Kubota and K. Itami, Science, 2019, 365, 272 CrossRef CAS PubMed;
(c) Y. Segawa, D. R. Levine and K. Itami, Acc. Chem. Res., 2019, 52, 2760 CrossRef CAS PubMed;
(d) B. P. Benke, T. Kirschbaum, J. Graf, J. H. Gross and M. Mastalerz, Nat. Chem., 2023, 15, 413 CrossRef CAS PubMed.
-
(a) M. Abe, Chem. Rev., 2013, 113, 7011 CrossRef CAS PubMed;
(b) Z. Sun, Z. Zeng and J. Wu, Acc. Chem. Res., 2014, 47, 2582 CrossRef CAS PubMed;
(c) Z. Zeng, X. Shi, C. Chi, J. T. L. Navarrete, J. Casado and J. Wu, Chem. Soc. Rev., 2015, 44, 6578 RSC;
(d) T. Kubo, Chem. Lett., 2015, 44, 111 CrossRef;
(e) T. Y. Gopalakrishna, W. Zeng, X. Lu and J. Wu, Chem. Commun., 2018, 54, 2186 RSC;
(f) C. Liu, Y. Ni, X. Lu, G. Li and J. Wu, Acc. Chem. Res., 2019, 52, 2309 CrossRef CAS;
(g) W. Zeng and J. Wu, Chem, 2020, 7, 358 CrossRef;
(h) S. Dong and Z. Li, J. Mater. Chem. C, 2022, 10, 2431 RSC.
-
(a) V. A. Sergeev, V. I. Nedel'kin and O. B. Andrianova, Vysokomol. Soedin., Ser. B, 1987, 29, 357 CAS;
(b) V. A. Sergeev, V. I. Nedel’kin, A. V. Astankov, A. V. Nikiforov, E. M. Alov and Y. A. Moskvichev, Izv. Akad. Nauk, Ser. Khim., 1990, 763 Search PubMed;
(c) D. Takeuchi, I. Asano and K. Osakada, J. Org. Chem., 2006, 71, 8614 CrossRef CAS;
(d) A. Ito, Y. Yokoyama, R. Aihara, K. Fukui, S. Eguchi, K. Shizu, T. Sato and K. Tanaka, Angew. Chem., Int. Ed., 2010, 49, 8205 CrossRef CAS;
(e) P. Chen, R. A. Lalancette and F. Jäkle, Angew. Chem., Int. Ed., 2012, 51, 7994 CrossRef CAS;
(f) Y. Ni, M. E. Sandoval-Salinas, T. Tanaka, H. Phan, T. S. Herng, T. Y. Gopala-krishna, J. Ding, A. Osuka, D. Casanova and J. Wu, Chem, 2019, 5, 108 CrossRef CAS;
(g) Z. Li, T. Y. Gopalakrishna, Y. Han, Y. Gu, L. Yuan, W. Zeng, D. Casanova and J. Wu, J. Am. Chem. Soc., 2019, 141, 16266 CrossRef CAS;
(h) G. Li, T. Matsuno, Y. Han, H. Phan, S. Wu, Q. Jiang, Y. Zou, H. Isobe and J. Wu, Angew. Chem., Int. Ed., 2020, 59, 9727 CrossRef CAS;
(i) Y. Ni, F. Gordillo-Gámez, M. P. Alvarez, Z. Nan, Z. Li, S. Wu, Y. Han, J. Casado and J. Wu, J. Am. Chem. Soc., 2020, 142, 12730 CrossRef CAS.
-
(a) S. J. Rowan, S. J. Cantrill, G. R. L. Cousins, J. K. M. Sanders and J. F. Stoddart, Angew. Chem., Int. Ed., 2002, 41, 898 CrossRef;
(b) M. E. Belowich and J. F. Stoddart, Chem. Soc. Rev., 2012, 41, 2003 RSC;
(c) Y. Jin, Q. Wang, P. Taynton and W. Zhang, Acc. Chem. Res., 2014, 47, 1575 CrossRef CAS.
-
(a) Y. Jin, C. Yu, R. J. Denman and W. Zhang, Chem. Soc. Rev., 2013, 42, 6634 RSC;
(b) M. J. Earle, G. Roberts and K. R. Seddon, Chem. Commun., 1998, 2097 RSC;
(c) M. Holler, N. Allenbach, J. Sonet and J.-F. Nierengarten, Chem. Commun., 2012, 48, 2576 RSC;
(d) X. Xie, Y. Wei, D. Lin, C. Zhong, L. Xie and W. Huang, Chin. J. Chem., 2020, 38, 103 CrossRef CAS;
(e) B. K. Reddy, A. Basavarajappa, M. D. Ambhore and V. G. Anand, Chem. Rev., 2017, 117, 3420 CrossRef CAS PubMed;
(f) T. Tanaka and A. Osuka, Chem. Rev., 2017, 117, 2584 CrossRef CAS PubMed;
(g) B. Szyszko, M. J. Białek, E. Pacholska-Dudziak and L. Latos-Grażyński, Chem. Rev., 2017, 117, 2839 CrossRef CAS PubMed;
(h) S. Dong, T. Y. Gopalakrishna, Y. Han and C. Chi, Angew. Chem., Int. Ed., 2019, 58, 11742 CrossRef CAS PubMed.
-
(a) F. Lopez-Garcia, S. Dong, Y. Han, J. J. C. Lee, P. W. Ng and C. Chi, Org. Lett., 2021, 23, 6382 CrossRef CAS PubMed;
(b) Q. Guo, Z. Fu, L. Zhao and M. Wang, Angew. Chem., Int. Ed., 2014, 53, 13548 CrossRef CAS PubMed.
-
(a) V. I. Minkin, Pure Appl. Chem., 1999, 71, 1919 CrossRef CAS;
(b) P. George, M. Trachtman, C. W. Bock and A. M. Brett, Tetrahedron, 1976, 32, 1357 CrossRef CAS.
-
(a) Y. Segawa, H. Omachi and K. Itami, Org. Lett., 2010, 12, 2262 CrossRef CAS PubMed;
(b) Y. Segawa, A. Yagi, H. Ito and K. Itami, Org. Lett., 2016, 18, 1430 CrossRef CAS PubMed.
- CCDC no. for FEM4+(PF6−)4 and CACz6+(SbF6−)6 is 1991177 and 2177038. The data is provided free of charge by The Cambridge Crystallographic Data Centre (CCDC)†.
- T. Lu and Q. Chen, Theor. Chem. Acc., 2020, 139, 25 Search PubMed.
- D. Geuenich, K. Hess, F. Köhler and R. Herges, Chem. Rev., 2005, 105, 3758 CrossRef CAS PubMed.
-
(a) J. Qian and B. Z. Tang, Chem, 2017, 3, 56 CrossRef CAS;
(b) G. Feng and B. Liu, Acc. Chem. Res., 2018, 5, 1404 CrossRef PubMed;
(c) H. Feng, Y. Yuan, J. Xiong, Y. Zheng and B. Z. Tang, Chem. Soc. Rev., 2018, 47, 7452 RSC;
(d) S. Xu, Y. Duan and B. Liu, Adv. Mater., 2019, 1903530 Search PubMed;
(e) J. Li, J. Wang, H. Li, N. Song, D. Wang and B. Z. Tang, Chem. Soc. Rev., 2020, 49, 1144 RSC.
|
This journal is © The Royal Society of Chemistry 2024 |