DOI:
10.1039/D3MA00937H
(Review Article)
Mater. Adv., 2024,
5, 5339-5350
Sensing methods for stress biomarker detection in human saliva: a new frontier for wearable electronics and biosensing
Received
31st October 2023
, Accepted 21st February 2024
First published on 29th February 2024
Abstract
The human stress response triggers a complex array of physiological, psychological, and biochemical reactions, involving neuroendocrine pathways such as the sympathetic adrenomedullary axis and hypothalamic–pituitary–adrenal axis. Prolonged experience of stress can have adverse effects on overall cognitive and physical well-being, making the routine monitoring of everyday stress highly desired for the delivery of personalised health management solutions. Saliva, as a diagnostic fluid, has garnered considerable attention in this regard due to its non-invasiveness, ease of collection and high compliance among diverse populations. Salivary biomarkers, including cortisol, salivary α-amylase, chromogranin A, brain-derived neurotrophic factor, and immunoglobulin A, are responsive to acute and/or chronic stressors, and thus, are prerequisite biomarkers in stress-related research. A number of sensing technologies are available for stress biomarker monitoring in saliva and other fluids. Enzyme-linked immunosorbent assays, colorimetric techniques, surface plasmon resonance sensing, and molecular imprinted polymers offer sensitive and selective cortisol detection in saliva. The integration of aptamers with electrochemiluminescence biosensing provides a label-free and cost-effective approach to biomarker detection. Wearable sensors that couple on-body sampling with edge computing for real time analytics open up new avenues for ambulatory monitoring and predictive diagnostics. The development of sensitive and reliable, saliva-based biosensing technologies in form of smart dentures and braces, holds the potential to revolutionize stress research with potential to provide personalized stress management solutions for health management in the future.
1. Background
Physiological and behavioural stress responses can be adaptive and function as prerequisites for ongoing resilience and growth.1 However, there is an imperceptible line beyond which stress can become maladaptive, compromising health and welfare. There is acceptance that elevated levels of stress experienced over a prolonged period could lead to diseases, such as cancer, coronary heart disease, respiratory disorders, cirrhosis of the liver, and depression.2,3 The human stress response is a continuous process that triggers a myriad of psychological, physiological, and biochemical responses mediated by overlapping neuroendocrine pathways.4 The first is the sympathetic adrenomedullary axis (SAM), which activates the release of epinephrine and norepinephrine into the bloodstream, acting immediately on heart rate and blood flow.5 The second is the hypothalamic–pituitary–adrenal (HPA) axis, which triggers a cascade of hormones like corticotrophin (CRH) and adrenocorticotropic hormone (ACTH) to activate the pituitary gland to secrete cortisol; a major stress or metabolic hormone.6,7 These neuroendocrine pathways also work with the immune system to develop and maintain homeostasis.8
Whilst excessive stress is synonymous with poor health, intermittent exposures to stress are highly adaptive.3 In addition, some individuals show resilience to different life stressors and may well thrive under repeated challenges. Therefore, the routine monitoring of stress in everyday life and related symptoms is crucial to unpack not only the causes and consequences of poor health outcomes, but also to understand why some individuals are predisposed to these outcomes and others are not.
Fig. 1 shows a wide array of sensors, ranging from highly engineered wearable sensors for biosensing, applied across the body and each targetting a particular fluid compartment.
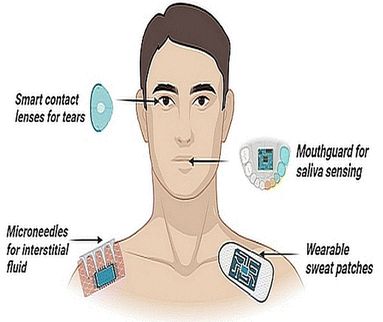 |
| Fig. 1 Non-invasive wearable sensors with embedded electronics and edge computing for the detection of biomarkers from different biological fluids, namely- tears, saliva, sweat and interstitial fluid. | |
By far, the principal fluid source of measurements for stress diagnostics is blood and there is a clear dearth of sensors that can measure stress non-invasively and in real-time. Moreover, traditional approaches such as venipuncture blood sampling themselves can be stress inducing and those who have a phobia of needles may produce a false positive cortisol response. Emerging approaches that utilize capillary blood sampling are more tolerable, but are highly variable in stress biomarker detection.9 Cannulated sampling of blood is another option but is generally limited to laboratory and clinical set-ups. Interstitial fluid (ISF) can overcome such limitations, but correlations with reference biomarkers in the blood are at an early developmental stage.10 Sweat as a biofluid offers another sampling alternative, although factors such as humidity, temperature and pH can elicit variable signals.11 Similarly, tears have shown some potential for stress hormone detection,12 but are limited by analytical challenges such as lower concentrations of biomarkers (e.g., cortisol and glucose), and drawing clinical outcomes from tear samples remains elusive.12,13
The correlation between saliva and blood-derived biomarkers is analyte-specific and complex. While extensive research has focused on blood and saliva testing, inherent challenges exist such as matrix effects, interferences, and sample viscosity.14 Despite the less invasive collection, not all analytes in saliva correlate well with blood (or urine) measures due to factors like partitioning rates, moiety conversion, and food intake.15 Consideration should also be given to the potential issue of blood leakage in the oral cavity, as it may lead to false signals due to saliva contamination as well as biomolecular transportation through the gingival crevicular fluid, which is derived primarily from microvascular (postcapillary venule) leakage.16,17 The presence of various proteins in saliva, along with food debris, poses another challenge in the practical application of wearable biosensors.18 This challenge arises from nonspecific adsorption, causing sudden biofouling of devices and affecting device reliability.19
In recent years, there has been exponential interest in the development of wearable sensors and biosensing technologies aimed at measuring different states of human physical performance. Recent developments in wearable electronics, in the form of electronic-skin (e-skin) and sol called performance patches, can overcome limitations that have restricted real-time measurements and monitoring of physical and cognitive stress in ambulatory state. Most recently, measurement of cortisol in nanomolar concentrations, with data transferred into smartwatch technology, has been realised, and putative steps towards other analytes, including serotonin and dopamine, have been made.20–22 Similarly, advances in polymers and graphene-based biosensing designs offer further potential, with polymers having proven themselves in other commercial markets (e.g. anesthetic monitoring).23–26 In this review, we focus on biosensing of stress biomarkers using saliva and present current and emerging sensing technologies that can collect and analyse biochemical data, longitudinally. We hope that this timely review will encourage further development of wearable electronic sensors using saliva as a sample matrix, a concept that could see our understanding of stress move from evolution to revolution and present new opportunities in the interdisciplinary area of sensor manufacturing and real time biosensing.
This review is organised in such a way as to give readers an understanding of the suitability of saliva as a diagnostic fluid and the rich prospects it offers for cognitive and physical performance prediction. Next, we review biosensing approaches starting from enzyme-linked immunosorbent assays (ELISA) that are still considered the gold standard of immunoassays and moving to emerging sensing platforms, including optical, electro-chemical approaches that are well suited for real-time sensing and compatible with wearable electronics platforms.
Fig. 2 shows the schematics of biosensors and relevant biosignal transduction principles that are at the focus of this review article.
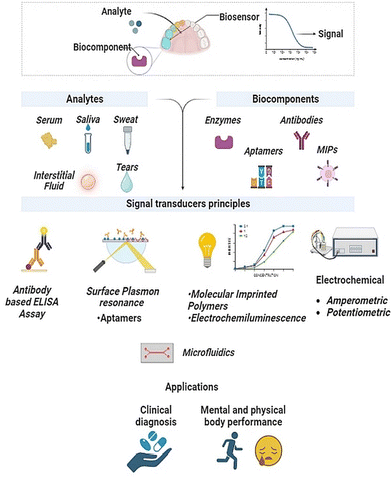 |
| Fig. 2 Potential state of art biosensing applications and their essential components as per sensing methodologies for point-of-care diagnostics. | |
2. Saliva as a diagnostic fluid
Saliva has garnered considerable attention as a diagnostic fluid, as it offers several advantages over other sampling methods.27,28 Firstly, it is relatively stress-free and easy to collect in reasonable quantities, and can be self-collected with relatively little training, making it accessible to a wider range of patients, including children and elderly.29 Moreover, samples can be collected at home, work, or other ecological settings. Saliva also contains a wide range of biomolecules, including proteins, hormones, and enzymes linked to stress, disease and poor health.30 These benefits have led to the development of saliva-based diagnostic tests for a range of medical conditions (e.g. oral and systemic diseases, stress and mental health).31 Whilst saliva presents novel sampling opportunities, caveats remain as to time lags between blood and saliva changes, individual concentration gradients and artifactual contaminants.32–34 Overall, saliva has the potential to revolutionize the field of medical diagnostics, providing a simple and accessible means for individuals to monitor their health status.17,27
Salivary biomarkers indicative of sympathetic (i.e. salivary α-amylase, chromogranin A [CgA]) and HPA (i.e. cortisol) activity, alongside proxy measures of cellular and humoral immunity (i.e. brain derived neurotrophic factor [BDNF], immunoglobulin A [IgA]), are widely used in the broad scoping field of stress research35 (Fig. 3). This review article describes each of these stress biomarkers and outlines different quantification techniques, with particular emphasis on salivary cortisol detection; being arguably the most widely researched and clinically applied biomarker of stress reactivity and adaptation.
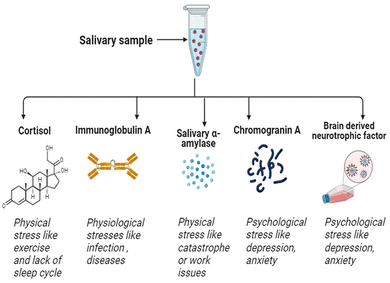 |
| Fig. 3 Salivary composition offers a plethora of stress biomarkers such as salivary α-amylase (sAA), cortisol, immunoglobulin A (IgA) and brain derived neurotropic factor (BDNF) and chromogranin A (CgA). | |
Saliva, at its source, is an optically clear and slightly acidic fluid, comprising water (99%), proteins (0.3%) and inorganic content (0.2%).36,37 This fluid, which is produced in the acinar cells of the salivary glands, has a daily secretory volume of 1–1.5 litre a day. Approximately 90% of total saliva secretion comes from the three major salivary glands (i.e. parotid, submandibular, sublingual), while the remaining 10% is produced in minor salivary glands. In a resting (unstimulated) state, 66% of total saliva volume originates from the submandibular glands.60 During stimulation, at least 50% of saliva is produced by the parotid glands. Sublingual (gland) contributions to total saliva are relatively small under both unstimulated and stimulated conditions.61 According to biochemical studies, saliva contains both organic (e.g. IgA, lactoferrin, amylase, mucins, and steroids) and inorganic molecules (e.g. sodium, potassium, calcium, magnesium, chloride, and phosphate).62 The salivary proteome also exhibits extreme diversity, with approximately 3000 different proteins and peptides identified, some of which can be used for disease prognosis and diagnosis.63
Stress in general can be defined by the period of exposure, among other factors (e.g. intensity, frequency). Here we define acute stress as transient exposure that lasts for a relatively short period of time. Such stressors include an examination, physical exercise or a laboratory task. Chronic stress, on the other hand, represents a more consistent repeated pressure, both real and perceived. This includes relationship problems, long-term physical training, and perhaps some medical conditions, such as diabetes, depression or high blood pressure.64 Here we focus on common biomarkers of stress, based on the broad classification of acute and chronic stress responsivity; see text below and Table 1 for an overview.
Table 1 Salivary stress biomarkers and their response to acute and/or chronic stressors
Biomarker |
Acute stress |
Chronic stress |
Cortisol |
Cortisol levels rise rapidly and then return to baseline38–40 |
Decrease in baseline cortisol due to HPA-axis dysregulation41 |
Immunoglobulin A |
Decreases IgA levels in different stressors42,43 |
Down regulation in IgA secretion42,44 |
Salivary α amylase |
Sympathetic system activates and increases amylase levels40,45–47 |
Sympathetic system activates and increases amylase levels48–50 |
Chromogranin A |
Co-activation of the SAM and HPA-axis can increase CgA51–53 |
The HPA-axis is activated in response to ongoing stressors40,54 |
BDNF |
BDNF is elevated, along with cortisol and adrenaline55,56 |
Chronic stress downregulates BDNF production57–59 |
2.1. Salivary α-amylase
Salivary α-amylase is the most abundant enzyme found in saliva, produced within the gastrointestinal system (i.e. pancreas) and oral cavity by the salivary glands.65,66 The role of this enzyme is to cleave α-linked carbohydrates into the by-products, glucose and maltose. Amylase is also secreted from the SAM following nerve stimulation and this source of amylase is independent of saliva flow.67 Evidence indicates no correlation between amylase measurements from both the axes,68 suggesting different functional roles. Nevertheless, research shows that salivary α-amylase is a highly sensitive stress biomarker that increases in response to acute46 and chronic psychosocial stress exposure.48,69 Salivary α-amylase increases abruptly at stress onset,70 thereby highlighting the utility of this biomarker for rapid stress detection.
2.2. Chromogranin A
Chromogranin A is an acidic, soluble glycoprotein produced from sympathetic nerve terminals and the adrenal medulla.71 A diverse array of acute stressors, such as public speaking, sports, noise, medical intervention, or driving a car, can all elicit a rapid and specific increase in CgA release.51,53,72–74 Consequently, salivary CgA offers some promise as a sensitive biomarker of ecological stressors in daily life. In addition, elevated CgA levels have been observed in patients experiencing conditions linked to chronic stress, such as anxiety disorders, posttraumatic stress disorder, and certain chronic diseases.75 A rise in CgA levels may further reflect other stress-related pathways, such as increased SAM activity and release of energy-related hormones (e.g. cortisol, adrenaline)76 (Table 1). The effect of chronic stress on CgA does, however, appear to be quite variable and contingent on factors like the duration and type of stress exposure.77–79
2.3. Cortisol
Cortisol is a fat soluble glucocorticoid, often termed the primary stress or metabolic hormone.80 Cascading signals from the hypothalamus (CRH) and pituitary gland (ACTH) stimulate the production and release of cortisol from the adrenal cortex. The primary role of cortisol is to mobilize energy stores via carbohydrate, fat and lipid metabolism; hence, salivary cortisol levels are typically elevated during, or after, an acute challenge.81,82 One may also expect a preparatory rise in cortisol concentration (e.g. pre-competition, pre-speech) to meet the expected demands of an impending challenge. Chronic stress, as characterized by real or perceived persistent demands, can lead to dysregulation of the HPA axis and higher basal cortisol levels.39
There are strong correlations between serum and salivary measures of cortisol concentrations,83–85 but these are complicated by hormone transport mechanisms. In blood, more than 90% of cortisol in circulation is bound to carrier proteins (e.g., albumin, corticosteroid binding globulin) and the remaining fraction (<10%) circulates in the unbound or “free” form.83,86 Free cortisol enters saliva via intracellular mechanisms and the measurement of salivary cortisol provides a proxy for serum-free cortisol that is biologically active in target tissue.84 Supporting evidence comes in the form of more dynamic changes in salivary (vs. total) cortisol with HPA stimulation85 or physical exercise.83 Salivary cortisol levels are unaffected by salivary flow rate and are relatively resistant to enzyme degradation from multiple freeze–thaw cycles. Salivary cortisol is stable for 3 months at 5 °C and for 1 year at −20 °C.86 It is also stable when saliva is stored at room temperature for a short period of time.87
2.4. Brain-derived neurotrophic factor
Saliva contains a plethora of growth factors including epidermal growth factor, nerve growth factor and insulin growth factor.88 The neurotrophin family, a subgroup of the broader growth factor family, includes BDNF.89 In addition to its mature form, which supports cell development and differentiation, survival, and plasticity, BDNF is present in its N-glycosylated pro-form, which stimulates cellular apoptosis.90 Blood platelets and circulating plasma both contain detectable quantities of BDNF. Recent studies have shown that pro- and mature BDNF are present in human saliva using immunoblotting and enzyme digestion.91 Acute stress such as physical exercise, can increase salivary BDNF level.56 Nakagawa et al.58 demonstrated that chronic stress can decrease BDNF levels in socially isolated rats. Other models of stress-induced behavioral and brain alterations can also reduce BDNF levels in saliva, blood and the brain cortex.92,93 Although salivary BDNF provides a sensitive biomarker of stress-induced change, it occurs in a relatively low concentration range (0.067–2.74 ng mL−1).91 See Table 2 for an overview.
Table 2 A summary of selected stress related biomarkers present in human saliva and their concentration ranges compared with serum concentrations
Family |
Stress biomarker |
Saliva concentration |
Serum concentration |
Sympathetic |
Salivary α-amylase |
2–400 U mL−1 94 |
40–140 U L−1 95 |
Chromogranin A |
Not commonly measured in saliva |
25–140 ng mL−1 96 |
Adrenal |
Cortisol |
0.012–3.000 μg dL−1 97 |
0.4–40 μg dL−1 98 |
Immune |
Brain derived neurotrophic factor |
0.067–2.74 ng mL−1 99 |
0.06–16 ng mL−1 100 |
Immunoglobulin A |
2.5–600 μg mL−1 101 |
1.6–100 ng mL−1 102 |
2.5. Immunoglobulin A
Immunoglobulin A is the most abundant antibody isotype in the mucosal immune system. When the body is in contact with any infectious disease or an allergen, IgA antibodies, are released as the first line of defense.103 As an important serum immunoglobulin, IgA also mediates several protective functions through interactions with specific receptors and immune mediators. A study on primates revealed a reduction in IgA levels in the presence of social stress.104 Whilst chronic stress can activate the HPA-axis and increase cortisol production, the immune system is suppressed, as indicated by a decline in salivary IgA level.44,86 Chronic stress also causes an increase in inflammatory cytokines, such as interleukin-6 (IL-6). Interleukins are indicators of immune function and are released in the presence of stress, injury and inflammation.105 Therefore, exposure to both acute and chronic stressors can lead to transient depression in this immunity biomarker.
2.6. Point-of-care diagnostics for salivary amylase
There are a few biosensors that target salivary α-amylase with point-of-care (PoC) applications. The PoC detection of α-amylase is accomplished through three main principles. Firstly, specific binding reactions between α-amylase and antibodies are utilized, with subsequent signal detection indicating the binding event.106 Another approach leverages the enzymatic activity of α-amylase, measuring starch consumption through methods like recording resonance frequencies or monitoring aggregation times of starch-stabilized CdTe nanogels.107 Lastly, detection involves analysing products generated from the α-amylase-catalysed hydrolytic reaction, such as maltose, glucose, or coloured substance such as 2-chloro-4-nitrophenyl-4-O-β-D-galactopyranosylmaltoside) which develops a yellow color with time.108
Recent developments of a smartphone-based sensor followed the same principle of an α-amylase-catalysed hydrolytic reaction.109 This sensor has a potentiometric reader and sensing chip with preloaded reagents, which convert amylase to maltose, and give test results in under 5 min.109 These diverse methods offer some flexibility in designing PoC assays for salivary α-amylase, allowing for tailored applications based on sensitivity, specificity, and ease of use in various diagnostic settings.
To our knowledge, there are no available PoC diagnostics for sensing salivary IgA, Chromogranin A and BDNF, probably due to their low concentration in saliva making detection more challenging. Also, they may not have reached the same level of commercial demand and established diagnostic potential, as compared to salivary cortisol and α-amylase. As research interest and clinical applications grow, there may be a greater incentive for the development of biosensors targeting these biomarkers in the future.
3. Point-of-care diagnostics for salivary cortisol
Nuclear magnetic resonance, liquid or gas chromatography, X-ray diffraction, and mass spectrometry are examples of preeminent analytical techniques for identifying and evaluating fluid biomarkers.110 With excellent selectivity and precision across a broad dynamic range, these analytical methods can extract a plethora of information about a given target analyte, including its structure, molecular weight, concentration, and identification. However, the equipment needed represents a substantial financial outlay, specialized lab space, and requires highly skilled operators. As such PoC diagnostics of stress biomarkers are gaining popularity, particularly via portable labs and more recently biosensing.
Biosensors represent compact, self-contained tools used to identify and quantify a target analyte. Biosensing offers an alternative solution for detecting stress biomarkers with relative ease and cost-effectiveness.13 Enzymes, antibodies, nucleic acids, and other biological sensing components are brought into close contact with a transducer (such as an optical, electrochemical, or piezoelectric component) to convert the biorecognition event into a more straightforward and quantifiable signal.111 In general, the relationship between the signal's output strength and concentration of the target analyte is linear. The outcome is then processed utilizing electronics and embedded software systems, which offer straightforward digital feedback using a reader device. Depending on the biomarkers present in saliva, and their chemistry, a biosensor can be designed for analytes with a low limit of detection (LOD) and high sensitivity, high selectivity, and appropriate response time.
Cortisol is the most widely accepted measure of stress, due to its role in the energetic pathways that allow us to prepare for, and respond to, challenges in everyday life and links to broad health outcomes. Hence, cortisol has been specifically targeted in the field of sensor development to better understand and manage stress-related disorders and other cortisol-associated conditions.39,41 This work has expanded into PoC diagnostic platforms for human stress and performance physiology, bringing biomarker measurements closer to the individual, whilst reducing patient costs. In primary care settings, PoC diagnostics are crucial for directing prompt patient care. These efforts include miniaturization of devices, device portability, and onsite sample handling and preparation. Collectively, these benefits help make PoC diagnostic devices useful for both clinical and home settings.112,113
3.1. Enzyme linked immunosorbent assay (ELISA)
Solid-phase benchtop ELISAs represent the most common method of salivary cortisol detection, based on the principle of competitive binding. The microtiter wells (in a 96-well plate) are coated with an antibody targeting the cortisol molecule. During an incubation period, cortisol present in a sample competes with a cortisol-horseradish peroxidase conjugate for binding to these antibodies. Next, the unbound conjugate is washed off and a chromogenic substrate solution, typically tetramethylbenzidine, is added and this yields a color change (from clear to blue). Upon stopping the reaction with 0.16 M sulphuric acid, a yellow color appears, and optical density in each well is read at 450 nm on a plate reader. For a competitive ELISA, the amount of cortisol present is inversely proportional to color density. Most commercial ELISAs have high selectivity and high sensitivity for cortisol. They also require small sample volumes (<50 μL per well) and cover an extended cortisol range (from 30–100 ng mL−1) to capture physiological changes or differences in most populations.114,115
Measurement bias is one consideration when using commercial ELISA kits. One study116 compared four commercial ELISAs (DSL, Salimetrics, IBL and DRG) and an in-house method (DELFIA) for assessing salivary cortisol in the same samples. Substantial differences in mean cortisol level, expressed in nmol L−1, were reported (DSL = 48.13, Salimetrics = 17.12, IBL = 23.46, DRG = 22.86, DELFIA 15.97). The ELISA-derived results were also inflated compared to reference values (mean = 13.44) determined using tandem liquid chromatography–mass spectrometry, although all measurements of salivary cortisol were strongly interrelated.
3.2. Electrochemical cortisol sensing
The basic principle of electrochemical sensing relies on leveraging the electrochemical changes that occur in response to binding events between the target molecule and bio probes. It also involves the use of nanomaterials and a modified working electrode to quantify the change in concentration of the target analyte.117 The labelled compounds can be immobilized on the electrode. Fig. 5 details assay strategies like the sandwich assay and redox labels, like methylene blue and ferrocene, that have been explored for electrochemical sensing of cortisol.118
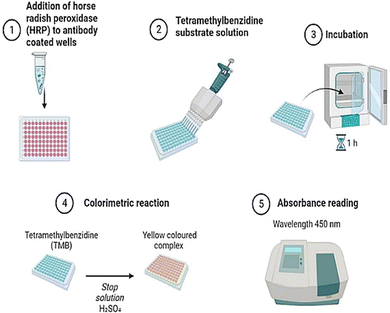 |
| Fig. 4 Enzyme linked immunosorbent assay for detection of salivary cortisol. The graphical representation showcases the observable color change of the assay plate, indicative of cortisol presence, along with corresponding absorbance readings at 450 nm. | |
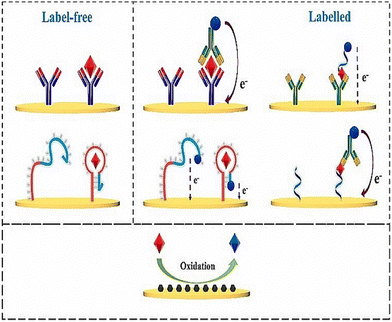 |
| Fig. 5 Electrochemical sensing mechanism with the help of labelled biorecognition elements, such as antibodies and aptamers, and unlabelled elements, such as using inherent material properties. Adapted and reprinted with permissions118 Copyright (2015), with permission from Elsevier. | |
Label-free electrochemical sensing methodologies have emerged as a compelling substitute for expensive optical detection protocols. These approaches are attributed to their remarkable features, including increased sensitivity, selectivity, and a low LOD. These label-free electrochemical immunosensing techniques offer a cost-effective and efficient means of detecting and quantifying target molecules. Sun et al.119 introduced an innovative immunosensing approach utilizing Au electrodes and alkaline phosphatase enzyme (AP) for the detection of cortisol in saliva. The electrochemical measurement in this method generates p-nitrophenol (pNP) as a measurable byproduct, serving as an indicative marker for cortisol levels.119 Chaker Tlili et al. designed a CNTs-based immunosensor using the chemiresistor principle for rapid, label-free cortisol detection in saliva at the POC application.120 However, the immunosensor exhibits limitations in matrix selectivity testing, as its resistance remained unchanged when exposed to ten-fold diluted synthetic saliva samples. Usha et al. created an optical sensor on fibres to identify salivary cortisol, employing the traditional method of resonance loss mode along with ZnO and Polypyrrole molecular nanocomposites.121 This sensor demonstrated an impressive LOD of 25.9 fg mL−1.121 It's noteworthy that all reported approaches have utilized either standard metallic electrodes or metallic fibres as sensing electrodes.122
3.3. Colorimetric technique
Cortisol, when added to organic reagents results in the formation of colored complexes that are fast, quantitative, and qualitative methods of detection.123 Thus, cortisol concentrations can be differentiated by observing corresponding changes in color. The end point of the chemical reaction is indicated by the formation of a bright colored complex. Chromogens such as sulfuric acid, Porter–Silber reagent, Prussian blue and blue tetrazolium yield different colored complexes of cortisol. Spectroscopic analysis can be performed using an UV-Vis spectrometer.124 Blue tetrazolium is the most favorable reagent for colorimetric detection giving a magenta color complex. The time for color development is around 10 minutes with an LOD of 97 ng mL−1 and low toxicity.124 The inclusion of gold nanoparticles, which can bind to cortisol, can give red colored complexes. These colorimetric methods can be adapted to form lateral flow assays to detect cortisol in saliva.125
3.4. Surface plasmon resonance (SPR) sensing
Surface plasmon resonance (SPR) is a label-free method for measuring salivary cortisol.126 Analyte binding produces a change in a refractive index that is measured by an optical detector. Receptors for binding have been amalgamated on a gold coated surface for target detection.127 These receptors can be aptamers or antibodies. Cortisol is a small molecule that does not elicit measurable changes in the refractive index. Thus, a competitive assay is used to enhance analyte binding (to antibodies) and enhance the signal with the help of a secondary marker. SPR occurs when p-polarized light is bombarded on the cortisol complex. The photons of p-polarized light can interact with the free electrons of the metal layer (Fig. 6), thereby inducing a wave-like oscillation of the free electrons, which reduces the reflected light intensity.128 SPR eliminates the use of markers or probes for cortisol detection.129
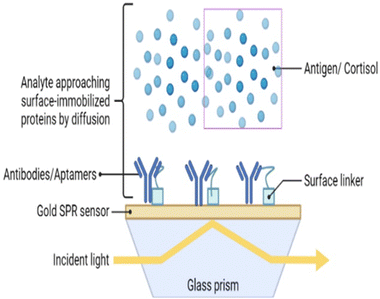 |
| Fig. 6 Surface plasmon resonance technique for specific analyte detection with the gold nanoparticles and antibody/aptamer as a biorecognition element. The detector measures the reflected wave and absorbed wave that travels through the prism. | |
There are limitations of this technique, namely the high cost and complex process for sampling. This has led to the development of an aptamer-based SPR sensor.130 The SPR aptasensor consisted of gold nanoparticles of various sizes, and the properties of the plasmonic sensor were optimized to improve its sensing capacity (Fig. 4). The LOD of this aptamer functionalized SPR biosensor with respect to salivary cortisol, was 0.1 nM. In addition, the SPR aptasensor selectively detected cortisol among other steroids (e.g. testosterone, aldosterone, and progesterone), thereby maintaining high selectivity and specificity. Salivary cortisol levels measured by the SPR aptasensor were validated with immunoassays.131,132
3.5. Molecular imprinted polymers technique
Molecular imprinted polymers (MIP) are bound with monomers, linkers and complexes in a covalent or noncovalent fashion to form a moiety.133 These are highly stable and present an ideal design for forming a receptor binding complex to detect macromolecules and micromolecules in a sample. The three main steps involved in formulating a MIP are prepolymerization, polymerization, and template removal.134 Prepolymerization is at the start the reaction between monomers into polymers. Polymerization is carried out by converting these monomers into polymers. The washing step involves the removal of template molecules. These scaffolds, when produced, give an attractive three-dimensional network for the binding of cortisol. These MIPs have lower production costs than antibody sensing. A working MIP-OFET sensor was designed as a proof of concept for salivary cortisol.133 The organic field effect transistor (OFET) had good amplification ability and detectability. Five monomers of 1, 2 diaminobenzene were used to bind to a single molecule of cortisol. The extended gate OFET produced a reasonable LOD of 0.72 μg L−1, coupled with excellent selectivity and no interference from analytes present in saliva.133 A nano gold-doped MIP film based on poly-o-PD (poly-phenylenediamine) was described by Yeasmin et al. to detect trace levels of cortisol in saliva135 (Fig. 7). Detection responsiveness and sensitivity increased by the in situ reduction and co-deposition of gold nanoparticles in MIP, which encouraged polymerization in the film and facilitated charge transfer. The authors demonstrated that the response current and cortisol concentration are directly proportional to each other, in the range of 1 pM–500 nM, with an LOD of approximately 200 fM.136 In other work, molecular imprinted polypyrrole was doped with cortisol and hexacyanoferrate ions as a redox probe coupled to β-cyclodextrin and reduced graphene oxide.137 The biosensor response was evaluated by changes in redox current measured by cyclic voltammetry. The biosensor demonstrated a broad detection range from 5 pg mL−1 to 5000 ng mL−1 and a low LOD of 19.3 pM, as well as similar results to that of salivary cortisol.137
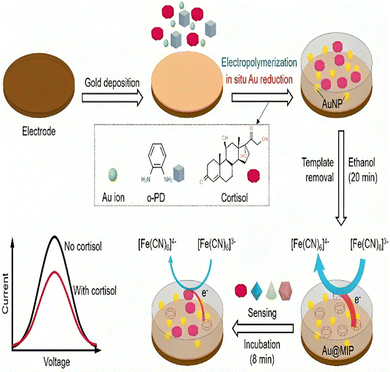 |
| Fig. 7 Fabrication process and sensing mechanism of a cortisol sensor on gold doped MIP layer. Adapted and reprinted with permissions135 Copyright (2015), with permission from Elsevier. | |
3.6. Aptamer based sensing
Aptamers have attracted considerable attention in the biosensing field, as signal recognition elements.21 The first cortisol aptamer sequence was found in 2014 by Martin et al.138 Aptameric sensing of cortisol was developed to overcome limitations against antibodies. Single-stranded DNA or RNA aptamers are highly selective and affine towards the matching target. Aptamers are isolated in vitro and have reversible denaturation characteristics. Aptamers are stable at room temperature and exhibit no batch-to-batch variation like antibodies. Aptamers can be an effective replacement for corresponding antibodies in cortisol diagnostic assays because of their superior signal enhancement qualities to those of antibodies (Fig. 8). The size of the cortisol molecule is 362.46 Daltons. Due to their small size, aptamers are typically chosen by Systematic Evolution of Ligands by Exponential Enrichment (SELEX).150 Gold nanoparticles are compatible with aptamers giving a colorimetric analysis of cortisol (Table 3).
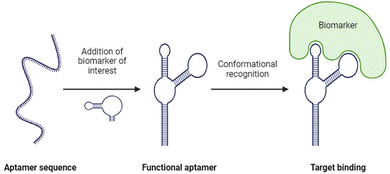 |
| Fig. 8 Functionalization of aptamer sequence for selective binding with cortisol that enhances limit of detection of the system. | |
Table 3 Comparative analysis of sensing technologies for cortisol detection depicting their limit of detection from immunoassays to MIP-based approaches
Sensing technologies |
Principle/method |
Detection range |
Sample type |
Ref. |
Enzyme-linked immunosorbent assay (ELISA) |
Antibody-based |
1–22.5 ng mL−1 |
Saliva, urine, blood, ISF |
114
|
Electrochemical sensors |
Electrochemical detection |
0.2–44.6 ng mL−1 |
Saliva, sweat, ISF |
139–142
|
Optical sensors |
Fluorescence, colorimetric, or chemiluminescence |
0.05–2 μg mL−1 |
Saliva, urine, blood, ISF |
124, 140 and 143
|
Mass spectrometry |
Mass spectrometric analysis |
0.5–20 nmol L−1 |
Saliva, urine, blood, ISF |
144 and 145
|
Microfluidic systems |
Microfluidic-based platforms |
0.25–0.5 μg mL−1 |
Saliva, blood, sweat, ISF |
146 and 147
|
Molecularly imprinted polymers (MIPs) |
Polymer-based recognition |
1 pM–500 nM |
Saliva, urine, sweat, ISF |
133, 148 and 149
|
Surface plasmon resonance (SPR) |
Optical detection |
1.5–10 ng mL−1 |
Saliva, blood, urine, ISF |
126 and 131
|
A novel quantitative method for detecting cortisol has been developed, utilizing aptamers without the need for target labelling, capture probe immobilization, or preliminary wash steps before obtaining results.150 In this approach, gold nanoparticles are functionalized with aptamers and pre-bound with electro-active triamcinolone, forming a recognition system. Cortisol levels are measured through competitive binding with the aptamer, and the signal is tracked by observing the displaced triamcinolone. This detection process is carried out using square wave voltammetry on patterned graphene-modified electrodes. What sets this method apart from contemporary biosensors, is its application in microfluidic or nanoslit devices, offering a streamlined and efficient approach to cortisol detection.151
Some aptamers have been amalgamated with other sensing technologies, such as SPR, electrochemical, and field effect transistors, to improve measurement specificity and selectivity.152 The surface of the sensor chip streptavidin (SA) can be immobilized with a biotinylated aptamer against cortisol. The aptamer immobilized chip can be used to inject a sample containing cortisol, with ensuing variations in reflectivity used to calculate the biomolecular interaction. The aptamer-based lateral flow biosensor works on the principle of DNA duplex dissociation.138 In the presence of cortisol binding with the gold nanoparticle aptamer complex, the DNA2 which is bound to the DNA1 aptamer gold nanoparticle complex is detached when bound to the cortisol molecule. The dissociated AuNP–DNA1 molecules released by the cortisol–aptamer interaction, are then free to be captured by the T-DNA on the test line through binding between complementary sequences. The color changes and is directly proportional to the concentration of cortisol.150 Fernandez et al.153 introduced a rapid POC biosensor for salivary cortisol detection on a paper substrate. This sensor, inkjet-printed on flexible paper, utilizes a metalloprophyrin-based macrocyclic catalyst ink capable of electrochemical interaction with cortisol. The cortisol is captured by aptamer-functionalized magnetic microbeads. Proof-of-concept experiments were carried out to assess salivary cortisol levels in individuals at varying risks of sleep apnea. Notably, the sensor incorporates a thin magnet disc positioned at the back of the electrode to concentrate the magnetic nanoparticle-bound cortisol onto the electrode sensing area. Aptamers and quantum dots detect cortisol from saliva through fluorescence quenching.122,143
3.7. Electrochemiluminescence (ECL)
Electrochemiluminescence (ECL), also known as electrogenerated chemiluminescence, has been ramped out in diagnostic devices due to the properties of an electron transfer step at the electrochemical interface.154 ECL involves the use of a co-reactant, which upon oxidation or reduction produces a moiety that on reaction with luminophore produces excitation. Oxidation or reduction takes place at the interface, and this generates reactive charged species that interact with luminophore to emit light.155 ECL has an inherent property of high sensitivity and low background noise. The most common luminophore used in ECL assays is the ruthenium(II) complex, which has three bipyridine rings and tripropylamine as the coreactant that exerts excellent results in both electrochemical and photophysical avenues.156 Other ECL approaches involve the luminol and hydrogen peroxide, both of which can be amalgamated with other oxidases for detection of specific proteins and drugs. The intensity of this emission can be measured by a photomultiplier tube with high voltage power supply. There are also sensors which involve aptamer integration with ECL, offering a novel method to measure salivary cortisol with low detection levels.21
Currently, there are few diagnostic devices available to measure salivary cortisol through chemiluminescence157 or electrogenerated chemiluminescence.158 A novel biosensor has been developed for the precise detection of salivary cortisol, employing a chemiluminescent lateral flow immunoassay (LFIA) method seamlessly integrated into a smartphone.159 This biosensor relies on a direct competitive immunoassay, utilizing a peroxidase–cortisol conjugate, and detection is facilitated by introducing a chemiluminescent substrate (luminol/hydrogen peroxide). The smartphone functions as a dual-purpose device, serving as both a light detector and a data handler through a designated application.159 The method is characterized by its simplicity, rapidity, and an impressively low LOD of 0.3 ng mL−1. Furthermore, it allows for quantitative analysis within the range of 0.3–60 ng mL−1, making it well-suited for detecting salivary cortisol within the clinically accepted range.
Detecting cortisol using antibody- and aptamer-based electrochemical methods faces significant challenges. Antibodies, which are commonly used as bioreceptors, are highly sensitive to changes in temperature and environmental conditions. They require careful handling during both transportation and use to prevent denaturation and alteration in structure, leading to a loss of functionality. This exclusive handling adds complexity and makes these methods impractical for wearable sensors.118 Moreover, the high cost and short shelf life of antibodies and aptamers further limit their application in wearable sensors.160 Due to these constraints, researchers are exploring MIP-ECL based methods to reliably detect cortisol. These efforts prioritize the development of detection techniques that offer better stability in various environmental conditions and improved selectivity for bioreceptors.161
4. Wearables for cortisol sensing through saliva
Wearable sensing technologies for health monitoring have largely focused on cortisol levels in saliva. Wearable devices tailored for this purpose often integrate sensing mechanisms, as discussed above, that are designed to directly interface with saliva. In these devices, cortisol, in conjunction with specific binding materials functions as biosensors inducing redox currents, altering surface charge, or changing impedance. Wearable sensors may employ microfluidic channels for saliva collection or strategically position themselves in areas where saliva is easily accessible. It's worth noting that despite the wearable nature of these sensors, some may still necessitate response time or additional power supply for real-time analysis. Emerging technologies like NFC or Bluetooth are being explored for power supply, although challenges such as saliva accumulation at the sensor site require resolution.162
While widely adopted wearable stress-monitoring devices primarily rely on heart rate, heart rate variability and electrodermal measurements, the potential of wearable sensors for continuous cortisol monitoring holds promise for disease detection and management through real-time health monitoring. Addressing current limitations, such as intermittent measurements and potential integration with therapeutic devices for closed-loop systems, remains crucial for the further development of cortisol sensor technology in the context of saliva analysis.163
5. Prospects for the embedded sensors for biosensing from the oral cavity
The continuous, ambulatory monitoring of stress biomarkers can be achieved using a raft of wearable sensors. Fig. 9 shows example of a wearable sensor designed for sensing salivary biomarkers. This is a growing area as research interest in standalone biosensors has increased dramatically over the last two decades with the advent of mobile devices and smartphones, alongside the miniaturization of these devices and integration of microelectronics and connective circuits.112,164,165,166 These developments include sensors specifically designed for the oral cavity to leverage the unique information contained in human saliva.167 The first oral wearable sensor was made in the 1960s, as a partial denture platform for observing mastication, plaque and fluoride concentrations in the oral cavity.19 The field of sensors in the oral cavity was expanded by development of graphene-based nanosensors that detect bacteria from tooth enamel. Adequate microfluidic flow and avoidance of “deadspace” over the sensor are essential challenges in developing a denture-based sensor. A sampling fluid “hysteresis” may occur if flow equilibrations between actual saliva and that experienced by the sensor is slow or inadequate.168
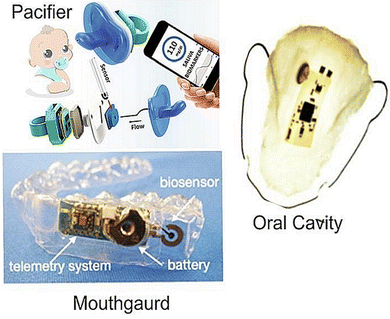 |
| Fig. 9 Wireless embedded sensors for the oral cavity to sense salivary biomarkers in vivo. Adapted and reprinted with permissions164 Copyright (2015), with permission from Elsevier. | |
A mouthguard-based sensor that detects uric acid has been developed by integrating microelectronics, Bluetooth and potentiostat.10 Other mouthguard sensors can monitor glucose levels via a glucose oxidase-modified electrode attached to the glycol surface with a wireless transducer integrated into the tooth guard.13,110 Intraoral biosensors contain a wide array of biochemical markers that help in diagnosing diseases. The oral cavity has teeth, a hard palate, a soft tongue and mucosal structures; each with well-defined movements. This knowledge helps us in designing devices for monitoring health conditions. Smart dentures need an electronic interface and biosensor mounted on a device such as a mouthguard, which detects these biomarkers smartly.169 Dental augmentation would be the most preferred site for its feasibility and requirement. Add-ons, such as braces, implants, crowns, dentures and ligatures, can also be used.169 There are currently no studies on wearable biosensors due to the omnipresence of other proteins in saliva.165,170 Hence, great opportunities exist to detect salivary cortisol through the advent of smart dentures and ligatures.
6. Conclusions
A range of stress biomarkers are detectable in saliva, with cortisol being the most prominent in research and practice. Numerous optical and electrochemical immunosensors, each with various biorecognition elements (e.g. antibody, aptamer, and MIP film), have been developed for the rapid, accurate, and quantitative detection of cortisol in saliva and other body fluids. More sophisticated electronic methods and microfluidics have expanded the creation of sensing techniques and, in particular, wearable sensors to permit the ambulatory monitoring of stress biomarkers. Efforts to construct immuno-biosensors for continuous and real-time monitoring of cortisol are ongoing, as difficulties and barriers still exist around binding sites, signal-analyte dynamics, and sensor architecture.
Conflicts of interest
There are no conflicts to declare.
Acknowledgements
This research is conducted as part of the Performance Patch Project and authors acknowledge funding from the Defence Science and Technology Group (DSTG). CP acknowledges support from the National Health and Medical Research Council (APP 2002576 and APP 2012560), Cancer Australia (APP1145657), the Garnette Passe and Rodney Williams Foundation, NIH R21 and the RBWH Foundation.
References
-
A. Feder, et al., Psychobiological mechanisms of resilience to stress, in Handbook of adult resilience, The Guilford Press, New York, NY, US, 2010, p. 35 Search PubMed.
- J. G. Rabkin and E. L. Struening, Science, 1976, 194(4269), 1013 CrossRef CAS PubMed.
- H. Yaribeygi,
et al.
, Excli J., 2017, 16, 1057 Search PubMed.
- E. S. Epel,
et al.
, Front. Neuroendocrinol., 2018, 49, 146 CrossRef PubMed.
-
S. Matovic, et al., Chapter 12 – Stress, aging, and inflammation, in Stress: Immunology and Inflammation, ed. G. Fink, Academic Press, 2024, p. 99 Search PubMed.
- M. F. Juruena,
et al.
, Adv. Exp. Med. Biol., 2020, 1191, 141 CrossRef CAS PubMed.
- C. Leistner and A. Menke, Handb. Clin. Neurol., 2020, 175, 55 Search PubMed.
- P. Holzer,
et al.
, Front. Immunol., 2017, 8, 1613 CrossRef PubMed.
- M. S. F. Hoffman,
et al.
, Expert Rev. Med. Devices, 2023, 20(1), 5 CrossRef CAS PubMed.
- J. Kim,
et al.
, Adv. Sci., 2018, 5(10), 1800880 CrossRef PubMed.
- R. Haeckel and P. Hänecke, Ann. Biol. Clin., 1993, 51(10–11), 903 CAS.
- A. Barmada and S. A. Shippy, Eye, 2020, 34(10), 1731 CrossRef PubMed.
- J. Kim,
et al.
, Nat. Biotechnol., 2019, 37(4), 389 CrossRef CAS PubMed.
- M. Song,
et al.
, Int. J. Oral Sci., 2023, 15(1), 2 CrossRef CAS PubMed.
- A. G. Cardoso,
et al.
, TrAC, Trends Anal. Chem., 2023, 160, 116965 CrossRef CAS.
- J. H. Kang and H. S. Kho, Clin. Chem. Lab. Med., 2019, 57(8), 1115 CrossRef CAS PubMed.
-
C. Punyadeera and P. D. Slowey, Chapter 22 – Saliva as an emerging biofluid for clinical diagnosis and applications of MEMS/NEMS in salivary diagnostics, in Nanobiomaterials in Clinical Dentistry (Second Edition), ed. K. Subramani, and W. Ahmed, Elsevier, 2019, p. 543 Search PubMed.
- M. Gröschl,
et al.
, Steroids, 2001, 66(10), 737 CrossRef PubMed.
- C. Moonla, ECS Sens. Plus, 2022, 1, 021603 CrossRef.
- C. S. Movassaghi,
et al.
, Anal. Bioanal. Chem., 2021, 413(27), 6747 CrossRef CAS PubMed.
- B. Wang,
et al.
, Sci. Adv., 2022, 8(1), eabk0967 CrossRef CAS PubMed.
- A. Cernat,
et al.
, Bioelectrochemistry, 2020, 136, 107620 CrossRef CAS PubMed.
- M. Petcu,
et al.
, Anal. Chim. Acta, 2001, 435(1), 49 CrossRef CAS.
- M. Petcu,
et al.
, Anal. Chim. Acta, 2004, 504(1), 73 CrossRef CAS.
- J. S. Mitchell,
et al.
, Steroids, 2006, 71(7), 618 CrossRef CAS PubMed.
- J. S. Mitchell,
et al.
, Analyst, 2009, 134(2), 380 RSC.
- E. Kaufman and I. B. Lamster, Crit. Rev. Oral Biol. Med., 2002, 13(2), 197 CrossRef PubMed.
- Y. Xu,
et al.
, Rapid Commun. Mass Spectrom., 2014, 28(5), 471 CrossRef CAS PubMed.
- J. Noiphung,
et al.
, Theranostics, 2018, 8(14), 3797 CrossRef CAS PubMed.
- A. Roi,
et al.
, Dis. Markers, 2019, 2019, 8761860 Search PubMed.
-
L. Trevisan França de Lima, et al., Chapter 10 - Saliva as a matrix for measurement of cancer biomarkers, in Cancer Biomarkers, ed. L. V. Ramanathan, et al., Elsevier, 2022, p. 297 Search PubMed.
- S. Konishi,
et al.
, Am. J. Phys. Anthropol., 2012, 149(2), 231 CrossRef PubMed.
- D. Božović,
et al.
, Med. Arch., 2013, 67(5), 374 CrossRef PubMed.
- K. T. Kivlighan,
et al.
, Hormones Behav., 2004, 46(1), 39 CrossRef CAS PubMed.
- A. J. Steckl and P. Ray, ACS Sens., 2018, 3(10), 2025 CrossRef CAS PubMed.
- T. Pfaffe,
et al.
, Clin. Chem., 2011, 57(5), 675 CrossRef CAS PubMed.
- K. D. Tang,
et al.
, Cancers, 2019, 11(4), 473 CrossRef CAS PubMed.
- K. E. Hannibal and M. D. Bishop, Phys. Ther., 2014, 94(12), 1816 CrossRef PubMed.
- D. H. Hellhammer,
et al.
, Psychoneuroendocrinology, 2009, 34(2), 163 CrossRef CAS PubMed.
- M. Tammayan,
et al.
, PLoS One, 2021, 16(8), e0256172 CrossRef CAS PubMed.
- J. González-Cabrera,
et al.
, Stress, 2014, 17(2), 149 CrossRef PubMed.
- I. Svobodová,
et al.
, PLoS One, 2014, 9(3), e90820 CrossRef PubMed.
- R. Deinzer,
et al.
, Int. J. Psychophysiol., 2000, 37(3), 219 CrossRef CAS PubMed.
- C. G. Engeland,
et al.
, Brain, Behav., Immun., 2016, 52, 11 CrossRef CAS PubMed.
- N. Takai,
et al.
, Arch. Oral Biol., 2004, 49(12), 963 CrossRef CAS PubMed.
- J. Strahler,
et al.
, Psychophysiology, 2010, 47(3), 587 CrossRef PubMed.
- Y. Tanaka,
et al.
, Neuropsychiatr. Dis. Treat., 2013, 9, 1899 Search PubMed.
- R. Vineetha,
et al.
, J. Clin. Exp. Dent., 2014, 6(2), e132 CrossRef PubMed.
- A. Jafari,
et al.
, Iran J. Psychiatry Behav. Sci., 2018, 12(1), e9350 Search PubMed.
- J. F. van Veen,
et al.
, Psychoneuroendocrinology, 2008, 33(10), 1313 CrossRef CAS PubMed.
- M. Miyakawa,
et al.
, Noise Health, 2006, 8(32), 108 CrossRef PubMed.
- K. Takatsuji,
et al.
, Biomed. Res., 2008, 29(4), 221 CrossRef CAS PubMed.
- V. Ng,
et al.
, J. Dent. Educ., 2003, 67(10), 1091 CrossRef PubMed.
- J. Hua,
et al.
, Psychoneuroendocrinology, 2014, 50, 53 CrossRef CAS PubMed.
- M. S. de Sousa Fernandes,
et al.
, Neural Plast., 2020, 2020, 8856621 Search PubMed.
- A. Moreira,
et al.
, J. Hum. Kinet., 2018, 65, 139 CrossRef PubMed.
- S. Suliman,
et al.
, Front. Integr. Neurosci., 2013, 7, 55 Search PubMed.
- Y. Nakagawa,
et al.
, J. Oral Sci., 2019, 61(4), 516 CrossRef CAS PubMed.
- F. Karege,
et al.
, Psychiatry Res., 2002, 109(2), 143 CrossRef CAS PubMed.
- E. Topkas,
et al.
, Clin. Chim. Acta, 2012, 413(13), 1066 CrossRef CAS PubMed.
- G. Iorgulescu, J. Med. Life, 2009, 2(3), 303 Search PubMed.
- T. W. Pittman,
et al.
, Theranostics, 2023, 13(3), 1091 CrossRef CAS PubMed.
- M. Castagnola,
et al.
, Acta Otorhinolaryngol. Ital., 2017, 37(2), 94 CAS.
- R. V. K. Siew,
et al.
, Psychoneuroendocrinology, 2023, 149, 106021 CrossRef PubMed.
- C. Peyrot des Gachons and P. A. Breslin, Curr. Diab. Rep., 2016, 16(10), 102 CrossRef PubMed.
- U. M. Bailey,
et al.
, J. Chromatogr. B: Anal. Technol. Biomed. Life Sci., 2012, 911, 21 CrossRef CAS PubMed.
- A. Arhakis,
et al.
, Open Dent. J., 2013, 7, 7 CrossRef CAS PubMed.
- F. A. Scannapieco,
et al.
, Crit. Rev. Oral Biol. Med., 1993, 4(3–4), 301 CrossRef CAS PubMed.
- L. Petrakova,
et al.
, PLoS One, 2015, 10(8), e0134561 CrossRef PubMed.
- U. M. Nater,
et al.
, Psychoneuroendocrinology, 2006, 31(1), 49 CrossRef CAS PubMed.
- S. Nagasawa,
et al.
, Biomed. Res., 1998, 19(6), 407 CrossRef CAS.
- S. Obara and H. Iwama, J. Clin. Anesth., 2005, 17(7), 554 CrossRef CAS PubMed.
- T. Lee,
et al.
, Acta Paediatr., 2006, 95(8), 935 CrossRef PubMed.
- Y. Kanamaru,
et al.
, Stress, 2006, 9(3), 127 CrossRef CAS PubMed.
- K. Łoś and N. Waszkiewicz, J. Clin. Med., 2021, 10(8), 1744 CrossRef PubMed.
- R. Den,
et al.
, Environ. Health Prev. Med., 2011, 16(3), 155 CrossRef CAS PubMed.
- X. Liu,
et al.
, J. Occup. Health, 2022, 64(1), e12321 CrossRef CAS PubMed.
- M. A. D'Amico,
et al.
, Endocr. Connect., 2014, 3(2), R45 Search PubMed.
- T. Yamakoshi,
et al.
, Med. Biol. Eng. Comput., 2009, 47(4), 449 CrossRef PubMed.
-
L. Thau, et al., Physiology, Cortisol, in StatPearls, StatPearls Publishing Copyright © 2023, StatPearls Publishing LLC, Treasure Island (FL), 2023 Search PubMed.
- P. Boucher and P. Plusquellec, Front. Endocrinol., 2019, 10, 749 CrossRef PubMed.
- F. P. Idris,
et al.
, OMICS, 2017, 21(2), 74 CrossRef CAS PubMed.
- B. T. Crewther,
et al.
, J. Sports Med. Phys. Fitness, 2010, 50(1), 85 CAS.
- Y. M. R. M. Estrada and P. R. Orlander, Chest, 2011, 140(5), 1216 CrossRef PubMed.
- W. S. Gozansky,
et al.
, Clin. Endocrinol., 2005, 63(3), 336 CrossRef CAS PubMed.
- S. Chojnowska,
et al.
, J. Clin. Med., 2021, 10(3), 517 CrossRef CAS PubMed.
- A. H. Garde and A. M. Hansen, Scand. J. Clin. Lab. Invest., 2005, 65(5), 433 CrossRef CAS PubMed.
- H. Kagami,
et al.
, Adv. Dent. Res., 2000, 14, 99 CrossRef CAS PubMed.
- J. Saruta,
et al.
, Jpn. Dent. Sci. Rev., 2020, 56(1), 43 CrossRef PubMed.
- S. Chakrapani,
et al.
, Cureus, 2020, 12(11), e11396 Search PubMed.
- A. L. Mandel,
et al.
, J. Immunoassay Immunochem., 2011, 32(1), 18 CrossRef CAS PubMed.
- V. Mondelli,
et al.
, J. Clin. Psychiatry, 2011, 72(12), 1677 CrossRef PubMed.
- P. Licznerski and E. A. Jonas, Proc. Natl. Acad. Sci. U. S. A., 2018, 115(15), 3742 CrossRef CAS PubMed.
- Salimetrics, Salimetrics Amylase assay, 2023, https://salimetrics.com/assay-kit/salivary-alpha-amylase-enzymatic-assay/.
- F. De Felice,
et al.
, Oncotarget, 2017, 8(52), 90496 CrossRef PubMed.
- X.-W. Qiao,
et al.
, BMC Endocr. Disord., 2014, 14(1), 64 CrossRef PubMed.
-
Salimetrics, Salimetrics cortisol assay, 2023, https://salimetrics.com/assay-kit/salivary-cortisol-elisa-kit/ Search PubMed.
-
C. C. H. P. Assays, Human Cortisol (Serum) ELISA Kit, 2023, https://www.crystalchem.com/cortisol-elisa-kit-serum.html.
- C. Vrijen,
et al.
, Psychiatry Res., 2017, 254, 340 CrossRef CAS PubMed.
- Sigma-Aldrich, Human BDNF ELISA Kit, 2023, https://www.sigmaaldrich.com/AU/en/product/sigma/rab0026.
- Salimetrics, Immunoglobulin A, 2023, https://salimetrics.com/analyte/salivary-secretory-immunoglobulin-a/.
- Thermofischer IgA Human ELISA Kit, 2023, https://www.thermofisher.com/elisa/product/IgG-Total-Human-ELISA-Kit/BMS2091.
- J. M. Woof and M. A. Kerr, J. Pathol., 2006, 208(2), 270 CrossRef CAS PubMed.
- F. A. Guhad and J. Hau, Neurosci.
Lett., 1996, 216(2), 137 CrossRef CAS PubMed.
- S. K. Lutgendorf,
et al.
, J. Gerontol., Ser. A, 1999, 54(9), M434 CrossRef CAS PubMed.
- T. V. da Silva Santos,
et al.
, Mater. Sci. Eng., C, 2012, 32(3), 530 CrossRef.
- H. Jiang and X. Wang, Analyst, 2012, 137(11), 2582 RSC.
- M. Yamaguchi,
et al.
, Biosens. Bioelectron., 2006, 21(7), 1007 CrossRef CAS PubMed.
- L. Zhang,
et al.
, Analyst, 2015, 140(21), 7399 RSC.
- R. S. Malon,
et al.
, BioMed Res. Int., 2014, 2014, 962903 Search PubMed.
-
H. O. Fatoyinbo and M. P. Hughes, Biosensors, in Encyclopedia of Nanotechnology, ed. B. Bhushan, Springer Netherlands, Dordrecht, 2012, p. 329 Search PubMed.
- A. Kaushik,
et al.
, Biosens. Bioelectron., 2014, 53, 499 CrossRef CAS PubMed.
- M. Gröschl, Clin. Chem., 2008, 54(11), 1759 CrossRef PubMed.
- M. Shimada,
et al.
, Horm. Res., 1995, 44(5), 213 CrossRef CAS PubMed.
- T. Ozgocer,
et al.
, J. Immunoassay Immunochem., 2017, 38(2), 147 CrossRef CAS PubMed.
- R. Miller,
et al.
, Psychoneuroendocrinology, 2016, 73, 16 CrossRef CAS PubMed.
- S. Madhu,
et al.
, Pharmaceuticals, 2022, 15(12), 1488 CrossRef CAS PubMed.
- G. Karuppaiah,
et al.
, Biosens. Bioelectron., 2023, 239, 115600 CrossRef CAS PubMed.
- K. Sun,
et al.
, Sens. Actuators, B, 2008, 133(2), 533 CrossRef CAS.
- C. Tlili,
et al.
, Biosens. Bioelectron., 2011, 26(11), 4382 CrossRef CAS PubMed.
- S. P. Usha,
et al.
, Biosens. Bioelectron., 2017, 87, 178 CrossRef CAS PubMed.
- M. Sekar,
et al.
, J. Electrochem. Soc., 2020, 167, 067508 CrossRef CAS.
- C.-E. Karachaliou,
et al.
, Biosensors, 2023, 13(2), 285 CrossRef CAS PubMed.
- E. Tu,
et al.
, ACS Omega, 2020, 5(14), 8211 CrossRef CAS PubMed.
- Y. Kim,
et al.
, Biosensors, 2021, 11(5), 163 CrossRef CAS PubMed.
- M. Piliarik,
et al.
, Methods Mol. Biol., 2009, 503, 65 CrossRef CAS PubMed.
- P. Damborský,
et al.
, Essays Biochem., 2016, 60(1), 91 CrossRef PubMed.
- H. H. Nguyen,
et al.
, Sensors, 2015, 15(5), 10481 CrossRef CAS PubMed.
-
D. U. Yildirim, et al., Nanosensors Based on Localized Surface Plasmon Resonance, in Plasmonic Sensors and their Applications, 2021, p. 23 Search PubMed.
- C. C. Chang, Biosensors, 2021, 11(7), 233 CrossRef CAS PubMed.
- R. C. Stevens,
et al.
, Anal. Chem., 2008, 80(17), 6747 CrossRef CAS PubMed.
- Y. Tahara,
et al.
, Front. Bioeng. Biotechnol., 2014, 2, 15 Search PubMed.
- Y. Sasaki,
et al.
, Sens. Actuators, B, 2023, 382, 133458 CrossRef CAS.
- N. Suda,
et al.
, R. Soc. Open Sci., 2017, 4(8), 170300 CrossRef PubMed.
- S. Yeasmin,
et al.
, Biosens. Bioelectron., 2022, 206, 114142 CrossRef CAS PubMed.
- S. Yeasmin,
et al.
, Biosens. Bioelectron., 2022, 206, 114142 CrossRef CAS PubMed.
- A. Goyal and T. Sakata, ACS Omega, 2022, 7(37), 33491 CrossRef CAS PubMed.
- J. A. Martin,
et al.
, Anal. Bioanal. Chem., 2014, 406(19), 4637 CrossRef CAS PubMed.
- S. Kämäräinen,
et al.
, Talanta, 2018, 188, 50 CrossRef PubMed.
- A. Ravalli,
et al.
, Biosensors, 2016, 6(3), 39 CrossRef PubMed.
- M. Sekar,
et al.
, Sci. Rep., 2019, 9(1), 403 CrossRef CAS PubMed.
- S. Madhu,
et al.
, J. Nanostruct. Chem., 2023, 13(1), 115 CrossRef CAS.
- Y. Liu,
et al.
, Langmuir, 2020, 36(27), 7781 CrossRef CAS PubMed.
- M. M. Kushnir,
et al.
, Clin. Biochem., 2004, 37(5), 357 CrossRef CAS PubMed.
- U. Turpeinen,
et al.
, Scand. J. Clin. Lab. Invest., 2009, 69(5), 592 CrossRef CAS PubMed.
- N. Dhull,
et al.
, J. Mater. Chem. B, 2022, 10(44), 9226 RSC.
- H. Tabasum,
et al.
, RSC Adv., 2022, 12(14), 8691 RSC.
- S. Ramanavičius,
et al.
, Sensors, 2022, 22(3), 1282 CrossRef PubMed.
- X. Liu,
et al.
, Analyst, 2011, 136(24), 5204 RSC.
- S. Dalirirad,
et al.
, ACS Omega, 2020, 5(51), 32890 CrossRef CAS PubMed.
- B. J. Sanghavi,
et al.
, Biosens. Bioelectron., 2016, 78, 244 CrossRef CAS PubMed.
- C. Yu,
et al.
, Analyst, 2022, 147(4), 744 RSC.
- R. E. Fernandez,
et al.
, Sci. Rep., 2017, 7(1), 17992 CrossRef PubMed.
- A. Kotani, Anal. Sci., 2020, 36(9), 1023 CrossRef CAS PubMed.
- X. Gou,
et al.
, Chem. Biomed. Imaging, 2023, 1(5), 414 CrossRef CAS.
- W. Zhu,
et al.
, Angew. Chem., Int. Ed., 2023, 62(7), e202214419 CrossRef CAS PubMed.
- N. M. Pires and T. Dong, Biomed. Mater. Eng., 2014, 24(1), 15 CAS.
- K. Ushiki,
et al.
, Sports Med. Open, 2020, 6(1), 38 CrossRef PubMed.
- M. Zangheri,
et al.
, Biosens. Bioelectron., 2015, 64, 63 CrossRef CAS PubMed.
- G. Karuppaiah,
et al.
, Bioelectrochemistry, 2022, 145, 108098 CrossRef CAS PubMed.
- E. S. Yulianti,
et al.
, Biosensors, 2022, 12(12), 1090 CrossRef CAS PubMed.
- J. Ok,
et al.
, Adv. Mater., 2024, 36(1), 2211595 CrossRef CAS PubMed.
- K. Li,
et al.
, Int. J. Environ. Res. Public Health, 2023, 20(24), 7146 CrossRef PubMed.
- P. Swetha,
et al.
, Electrochem. Commun., 2022, 140, 107314 CrossRef CAS.
- W. Hong and W. G. Lee, Analyst, 2021, 145(24), 7796 RSC.
- Ž. V. Popović,
et al.
, Sci. Rep., 2023, 13(1), 21277 CrossRef PubMed.
- D. R. Seshadri,
et al.
, npj Dig. Med., 2019, 2(1), 72 CrossRef PubMed.
- R. Ghaffari,
et al.
, Sens. Actuators, B, 2021, 332, 129447 CrossRef CAS PubMed.
- A. A. Smith,
et al.
, Sci. Rep., 2023, 13(1), 4998 CrossRef CAS PubMed.
- E. R. Kim,
et al.
, Trends Biotechnol., 2023, 41(3), 374 CrossRef CAS PubMed.
|
This journal is © The Royal Society of Chemistry 2024 |