The impact of nTiO2 and GO (graphene oxide), and their combinations, on freshwater Chlorella sp.: a comparative study in lake water and BG-11 media†
Received
27th January 2024
, Accepted 20th May 2024
First published on 20th May 2024
Abstract
Titanium dioxide nanoparticles (nTiO2) and graphene oxide (GO) are extensively used nanomaterials in various products and applications. Freshwater ecosystems are a crucial sink for these pollutants, posing severe threats to aquatic organisms. Although multiple studies have investigated the pristine toxicity of nTiO2 and GO in freshwater organisms, the combined toxicity of these materials remains unexplored. Interaction media is a crucial factor in evaluating toxicity nanomaterial toxicity towards algae. In this study, we have investigated the comparative effect of sterilized and filtered freshwater and BG-11 medium on the pristine and combined toxicity of nTiO2 and GO on freshwater algae Chlorella sp. Results indicated that the combination of nTiO2 and GO showed more toxicity when compared to their respective pristine forms. This could be due to the additive effect exhibited by nTiO2 and GO on Chlorella sp. The enhanced growth inhibition for the combined toxicity was in the order of 1 mg L−1 nTiO2 + 1 mg L−1 GO > 1 mg L−1 nTiO2 + 0.1 mg L−1 GO > 0.1 mg L−1 nTiO2 + 1 mg L−1 GO > 0.1 mg L−1 nTiO2 + 0.1 mg L−1 GO. All test groups that interacted in BG-11 media exhibited less toxicity when compared to corresponding groups in the lake water medium. This could be attributed to the cushioning effect of BG-11 medium, providing supplementary nutrition to the algal cells. This signifies that the environmentally relevant conditions could be more detrimental than the laboratory conditions. This study elucidates valuable insights into the potential detrimental effects associated with the combination of nTiO2 and GO on freshwater algae. Furthermore, we have evaluated the growth inhibition, oxidative stress, and photosynthetic activity of Chlorella sp. in both environmentally relevant interaction medium and well-defined culture medium.
Environmental significance
The introduction of nTiO2 and GO nanoparticles in the freshwater systems have raised concerns regarding their potential toxicity to aquatic organisms, particularly algae. These nanoparticles, often used in various industrial applications, can enter water bodies through runoff or direct discharge. To the best of our knowledge, this is the first study to investigate the combined toxicity of nTiO2 and GO in freshwater alga Chlorella sp. and compared the effects of interaction medium in modulating the toxicity on algae. Most freshwater algal toxicity studies used a defined culture medium, limiting insight into the effects of environmentally relevant mediums like freshwater. To address this gap, we have compared the effect of interaction medium (lake water and BG-11) on modifying the toxicity of nTiO2 and GO and their combination.
|
1 Introduction
Engineered nanomaterials (ENMs) have been increasingly incorporated into various consumer and industrial products.1 Among metal oxide nanoparticles (NPs), titanium dioxide NPs (nTiO2) are the most extensively produced nanomaterial globally.2 Projections suggest that the annual production of nTiO2 is expected to reach 2.5 million tons by 2025.3 nTiO2 finds diverse applications in various sectors, such as cosmetics, food, textiles, photocatalytic processes, and different personal care products.2,4,5 Reports suggest that the environmentally relevant concentration of nTiO2 was up to 1.2 mg L−1.6 Notably, nTiO2 can be discharged into aquatic systems through wastewater treatment plants (WWTP) effluents, surface runoff, the mismanagement of industrial waste, and various anthropogenic activities.7,8
Similarly, in the domain of carbon-based materials, graphene oxide (GO) plays a pivotal role as a central intermediate in the formulation of various other graphene-based substances, contributing to its widespread commercial applications.9 GO finds extensive applications in photocatalysis, antibacterial functions, sensor technology, and various environmental and biomedical applications.10,11 The environmentally relevant concentration of GO was found to be in the range of 0.001–10 mg L−1.12,13 GO can be discharged into aquatic systems through diverse applications, such as WWTP, water purification, seawater desalination, and water filtration processes.14
The widespread use and incorrect disposal of nTiO2 and GO increases the likelihood of their release into freshwater systems through various means, such as WWTP, solid-phase extraction materials, and as a catalyst used to oxidize and break down organic pollutants in water. This can result in their combined toxicity towards aquatic organisms.15–22 TiO2 NPs are well-known for their photocatalytic and other physiochemical properties.23 Similarly, GO is highly dispersible and mobile in aquatic environments. It is reported that GO sheets could form hetero-aggregates with nTiO2.24 The distinctive features of the materials would definitely influence the bio-toxicity of an organism when interacting with each other.
Microalgae are prominently recognized as crucial model organisms in ecotoxicological assessments.25–27 Their prevalence in such evaluations can be attributed to their rapid growth, facile cultivation, and significance in providing valuable insights into the ecological impacts of various contaminants.28Chlorella sp. is a crucial green, eukaryotic, photosynthetic microorganism that adapts quickly to new environments. Chlorella sp. can multiply asexually at a high rate in optimum conditions, which makes it an ideal aquatic organism for toxicity evaluation studies.29 Interaction medium plays a crucial role in determining the toxicity of NMs in algae.30 The bioavailability of NMs in interaction media varies significantly due to the profound effect of microalgal media components; therefore, the bioassay results may vary depending on the interaction medium.31 Aravantinou et al., 2015 found that when exposed to ZnO NPs, freshwater algae Scenedesmus rubescens exhibited less toxic effect in modified Blue-Green 11 (BG-11) medium than Bold Basal Medium (BBM).32
Several investigations have documented the adverse impacts of nTiO2 on freshwater microalgae. According to AL-Ammari et al., 2021, P25 nTiO2 exhibited more growth inhibition in freshwater microalgae Chlorella pyrenoidosa when compared to bulk and ultra-fine TiO2.33 As reported by Roy et al., 2016, Chlorella sp. displayed more growth inhibition than Scenedesmus sp. when exposed to a concentration of 1 mg L−1 of nTiO2.34,35 Liu et al., 2018 reported that 1 mg L−1 nTiO2 produced significant ROS in freshwater algae Scenedesmus obliquus compared to similar concentrations of SiO2 and ZrO2.36 Li et al., 2020 reported that nTiO2 at 10 mg L−1 substantially reduced photosynthetic yield and increased reactive oxygen species (ROS) and malondialdehyde (MDA) levels in Scenedesmus obliquus.37 Several other studies have documented the detrimental effects exhibited by nTiO2 on various microalgae.38–41
According to Ouyang et al., 2015, freshwater microalgae Chlorella vulgaris exhibited growth inhibition when subjected to 1 mg L−1 of GO.42Ji et al., 2020 reported that exposure of Chlorella vulgaris to GO at 0.01, 0.1, 1, and 10 mg L−1 exhibited significant ROS and SOD production.43 Kim et al., 2022 found that exposure to GO inhibited photosynthesis in Euglena gracilis, which was the primary mechanism underlying its cytotoxic effects.44 According to Zhao et al., 2017, GO elicited a substantial shading effect in freshwater algae Chlorella pyrenoidosa, which was linked to their significant growth inhibition.45 Nogueira et al., 2015 investigated the adverse effects of GO on freshwater algae Raphidocelis subcapitata in freshwater oligo medium. Their findings indicated that cell damage, ROS generation, and shading effect are the primary mechanisms of toxicity.46 Similarly, Yan et al., 2022 reported that exposure to GO had alerted the cell permeability and ultrastructure of microalgae Microcystis aeruginosa.14
To the best of our knowledge, this is the first study to investigate the comparative effects of the interaction medium in the combined toxicity of nTiO2 and GO in freshwater alga Chlorella sp. Most of the freshwater algal toxicity studies used a defined culture medium, limiting insight into the effects of environmentally relevant mediums like freshwater. To address this gap, we have compared the effect of the interaction medium (lake water and BG-11) on modifying the toxicity of nTiO2 and GO and their combination. We hypothesize that the combination of nTiO2 and GO would enhance the detrimental effects on Chlorella sp. Additionally, we propose that there would be variations in toxicity between lake water (LW) and BG-11 due to the increased nutritional availability in the BG-11 medium, along with differences in colloidal stability. The assessment of growth inhibition, shading effect, reactive oxygen species generation, malondialdehyde levels, and photosynthetic yield are employed to ascertain the influence of the interaction medium in modulating the potential toxicity of nTiO2, GO, and their combination.
2 Materials and methods
2.1. Chemicals and materials preparation
The nTiO2 (Aeroxide P25-21 nm) purchased from Sigma-Aldrich was used in the study. The GO was prepared according to a previously published protocol (ESI, Methods S1).† Further details regarding the chemicals used in this work can be found in the ESI (Methods S2).†
A stock solution of nTiO2 and GO was prepared by taking 5 mg of respective nanomaterials with 50 mL of Milli-Q water. nTiO2 and GO were then sonicated (Probe sonicator, 130 W, Sonics, USA) for 30 and 20 min, respectively. The working concentrations of nTiO2 and GO were fixed at 0.1 mg L−1 and 1 mg L−1.
2.2. Preparation of interaction media
Two different interaction media were used for this particular study. One was the growth medium of algae, i.e., BG-11, and the other was filtered and sterilized lake water obtained from the VIT lake, Vellore (12°58′10′′N, 79°9′37′′E). Lakewater media was used to mimic natural environmental conditions without changing the physicochemical properties mentioned in our previous study.47 The BG-11 media (procured from Hi-media Pvt. Ltd) was prepared according to the pack instructions, following which it was subjected to sterilization using an autoclave at 121 °C for 15 min. The collected lake water was subjected to serial filtrations (3 times) using the Whatman no. 1 (pore size-11 μm) filter paper to remove all the larger particles. The clear, filtered water was then sterilized using an autoclave at 121 °C for 15 min to remove all unwanted microorganisms.48 This filtered, sterilized lake water was then cooled to room temperature and used as the interaction media.
2.3. Characterization of nTiO2, GO, and their mixture
Characterization of pristine materials and their mixtures in both media, i.e., BG-11 and lake water (LW), are provided in the ESI (Methods S3).†
2.4. Test organism
Freshwater microalga Chlorella sp. was used in the current work as a test organism. The Chlorella sp. was isolated from the lake in VIT, Vellore. The isolation was done by adapting the protocol by Sadiq et al., 2011.49 To ensure proper growth, algal cultures were subcultured in sterilized BG-11 media and kept at 23 ± 2 °C with a photoperiod of 16 h. White fluorescent lamp (3000 lx illumination; Philips TL-D Super 80, linear fluorescent lamp, India)50 was used as a light source.
2.5. Toxicity studies
2.5.1. Experimental setup.
The algal culture was taken from the exponential phase for the toxicity evaluation in both the media, i.e., LW and BG-11, followed by centrifugation for 10 min at 7000 rpm at 4 °C. The collected pellet was re-suspended in the lake water, and BG-11 media, separately. The optical density was adjusted as 0.5 at 610 nm using a colorimeter. The interaction between nTiO2, GO, and the binary mixtures of these two were conducted in two different media, i.e., (a) BG-11 and (b) lake water media. Experiments conducted in the BG-11 medium were maintained with respective control groups in the BG-11 medium. Similarly, experiments conducted in the lake water medium possess corresponding control groups in the lake water medium. All the control and test groups were incubated for 72 h under the visible light condition (3000 lux).51 To replicate actual environmental conditions, lake water was chosen as an interaction medium.52 Furthermore, to compare the effect of defined culture medium with environmentally relevant lake water medium, BG-11 medium was chosen. In both media, the working concentrations of nTiO2 (0.1 and 1 mg L−1) and GO (0.1 and 1 mg L−1) were selected based on environmentally relevant concentrations. In both the media, combinations of these two materials were: (i) nTiO2 (0.1 mg L−1) + GO (0.1 mg L−1); (ii) nTiO2 (0.1 mg L−1) + GO (1 mg L−1); (iii) nTiO2 (1 mg L−1) + GO (0.1 mg L−1); and (iv) nTiO2 (1 mg L−1) + GO (1 mg L−1).
2.5.2. Evaluation of cytotoxicity and shading effects.
After 72 h exposure, a volume of 10 μL of the control and the treated samples from the BG-11 and lake water interaction medium were collected. These collected samples were then placed on a hemocytometer and observed under an optical microscope (Zeiss Axiostar Microscope, USA) to find the number of healthy viable cells (devoid of any deformities). The number of viable cells in the control samples was considered 100%, and the test samples for both the interaction media were measured concerning the control.53 The details regarding the same are provided in the ESI section (ESI Methods S4).† The results of the shading of light by the nanomaterials on the algal cells and its impact on growth inhibition in both the interaction medium were studied.54
2.5.3. Analysis of oxidative stress.
The overall ROS generation in both control and test groups was assessed utilizing the 2′,7′-dichlorofluorescein diacetate (DCFH-DA) dye.55 The amount of MDA produced within the control and treated cells was assessed using 2-thiobarbituric acid (TBA) reactivity (TBAR) assay.48 The details of both the assays are provided in the ESI (Methods S6).†
2.5.4. Analysis of photosynthetic parameters.
The electron transport rate and the effective quantum yield (Y(II)) of the photosystem II of the control and the treated algal cells for both the interaction media were quantified using the mini-PAM instrument.56,57 The details of the same are provided in the ESI (Methods S7).†
2.6. Mathematical modeling
Abbott's model was used to evaluate the nature of the interaction between nTiO2 and GO in the combination.48,58 Based on the growth inhibition data, the IA model assessed the interaction between the components in the binary mixture, i.e., between nTiO2 and GO. | 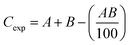 | (1) |
|  | (2) |
Eqn (1) was utilized to determine the expected toxicity of the mixture of GO and nTiO2, where A and B signify the individual toxicity of GO and nTiO2, respectively. CObs represent the observed toxicity of the mixture. The nature of the interaction between the two nanomaterials was identified using the inhibition ratio (RI), which was determined using eqn (2). The ESI (Methods S5)† provides detailed information on the same. Pearson modeling was performed to determine the correlation between the biological parameters.
2.7. Statistical analysis
All the experiments done for this study were performed in triplicates (n = 3). The results were reported as mean ± SD (standard deviation). A two-way ANOVA test with a Bonferroni post-test was employed in GraphPad 8.0 to determine the statistical significance between the control and test samples for both interaction media.
3 Results and discussion
3.1. Characterization of nTiO2 and GO
FESEM images (Fig. 1) revealed the cubic or spherical morphology of nTiO2 (Fig. 1A (LW) and 1D (BG-11)) and sheet-like structure for GO (Fig. 1B (LW) and 1E (BG-11)), in both the interaction media i.e. LW and BG-11. A combination of these two materials at 1 mg L−1 concentration (Fig. 1C (LW) and 1F (BG-11)) showed the attachments of nTiO2 on the surface of GO.
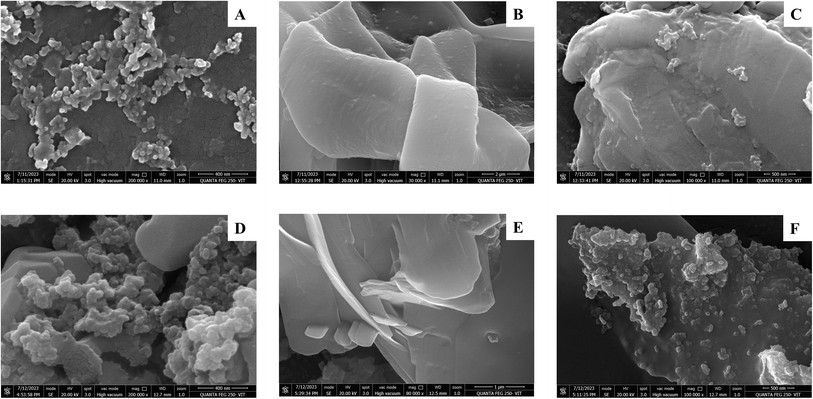 |
| Fig. 1 FESEM images of (A) nTiO2 1 mg L−1 in LW; (B) GO 1 mg L−1 in LW; (C) 1 mg L−1 nTiO2 + 1 mg L−1 GO in LW; (D) nTiO2 1 mg L−1 in BG-11; (E) GO 1 mg L−1 in BG-11; (F) 1 mg L−1 nTiO2 + 1 mg L−1 GO in BG-11. | |
The surface charge was measured for both the materials in their pristine and mixture forms in LW and BG-11 medium. The zeta potential values depicted that materials were more stable in LW media than BG-11. At lower concentrations (0.1 mg L−1), nTiO2 exhibited the zeta potential value of −14.08 ± 3.21 in LW and −10.67 ± 0.5 in BG-11. Meanwhile, GO at the same concentration exhibited a zeta potential of −13.8 ± 2.42 mV in LW and −8.81 ± 1.2 in BG-11. At higher concentrations (1 mg L−1), nTiO2 disclosed the value of −19.5 ± 1.7 mV in LW and −14.16 ± 2.28 mV in BG-11 medium. Meanwhile, at the same concentration, GO showed the zeta potential of −17.32 ± 2.2 mV in LW and −13.23 ± 2.73 mV in BG-11 media.
The combination of 0.1 mg L−1 nTiO2 + 0.1 mg L−1 GO resulted in a zeta potential value of −14.85 ± 1.2 mV for LW and −11.98 ± 1.7 mV for BG-11 media. The combination of 1 mg L−1 nTiO2 + 1 mg L−1 GO showed the zeta potential of −37.49 ± 4.7 mV for LW and −18.7 ± 3.7 mV for BG-11, respectively. In addition, 1 mg L−1 nTiO2 + 0.1 mg L−1 GO showed −28.53 ± 1.96 and −16.75 ± 6.4 mV in LW and BG-11, respectively. The mixture of 1 mg L−1 GO and 0.1 mg L−1 nTiO2 depicted the values of −25.4 ± 5.6 and −14.47 ± 2.93 mV for LW and BG-11, respectively.
FESEM images (Fig. 1) disclosed that the size of GO is higher than nTiO2, and this is due to the sheet-like structure of GO. When comparing the two-interaction media, the attachment of nTiO2 particles over the GO sheets was slightly higher in BG-11 media. Due to abundant oxygen functional groups on the surface of GO, nTiO2 can easily bond with the GO sheets.59 According to the zeta potential values in both the interaction media, it can be implied that all test groups are more stable in the LW than BG-11. This observed instability in the BG-11 test groups indicates a tendency for material aggregation in the BG-11 medium. This could lead to reduced availability of NMs to the algal cells compared to that in LW.60
3.2. Growth inhibition
Fig. 2 illustrates the growth inhibition observed in Chlorella sp. when exposed to pristine GO, pristine nTiO2, and their combination in BG-11 medium (Fig. 2A) and lake water (Fig. 2B). In BG-11, significant growth inhibition (p < 0.001) was observed in all experimental groups in comparison to the control group. A similar trend was observed in all the LW test groups. In this study, it was observed that there was no significant difference (p > 0.05) in the growth inhibition between pristine GO and pristine nTiO2, regardless of the concentration and the interaction medium. The primary cause of growth inhibition may be due to the cell membrane damage resulting from the physical interaction of NMs to the algal cells.61 In a previous study, it was reported that nTiO2 exhibited significant growth inhibition when exposed to freshwater algae Chlorella sp. at concentrations of 0.25, 0.5, and 1 mg L−1.34 Likewise, Hazeem et al., 2017 documented significant growth inhibition in microalgae Picochlorum sp. when exposed to concentrations of 0.5 and 1 mg L−1 of GO over 24 to 96 hours.62
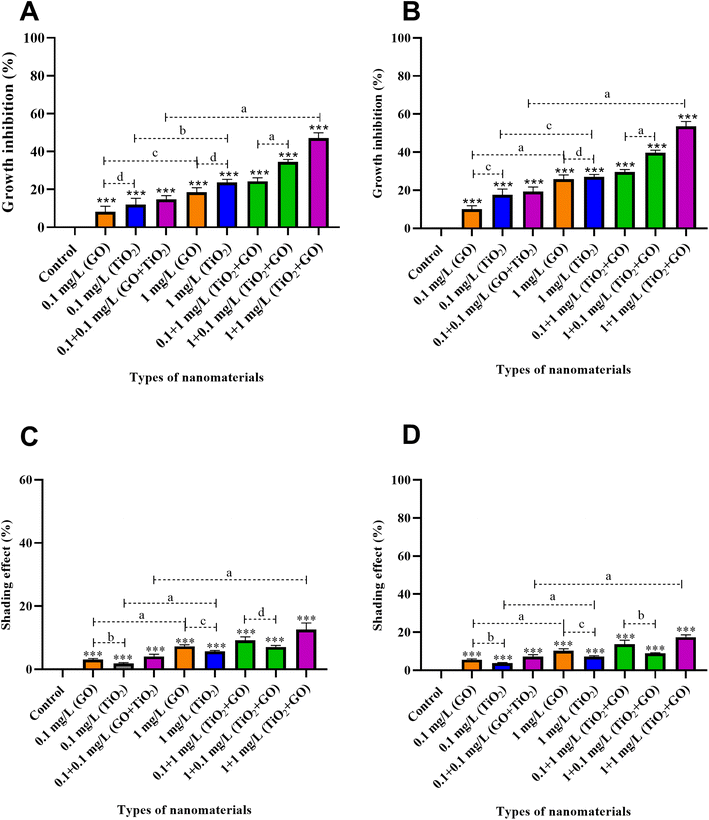 |
| Fig. 2 (A) Percentage growth inhibition of Chlorella sp. on exposure to pristine nTiO2 and pristine GO and their combination in BG-11 medium (B) under lake water (C) shading effect in BG-11 medium as interaction (D) shading effect in lake water. Note: The symbol ‘***’ denotes the significance between the test and control groups; a, b, c, and d indicate the significant difference between the groups (a = p < 0.001, b = p < 0.01, c = p < 0.05, and d = p > 0.05 (no significance)). | |
In the combination experiments, the trend in growth inhibition for BG-11 medium is as follows: 1 mg L−1 nTiO2 + 1 mg L−1 GO > 1 mg L−1 nTiO2 + 0.1 mg L−1 GO > 0.1 mg L−1 nTiO2 + 1 mg L−1 GO > 0.1 mg L−1 nTiO2 + 0.1 mg L−1 GO. A similar trend was observed in the combination experiments in the LW. The mixture of 0.1 mg L−1 nTiO2 + 0.1 mg L−1 GO exhibited significant growth inhibition when compared to their respective pristine forms in both LW and BG-11 media. A similar trend was observed for the mixtures of 1 mg L−1 nTiO2 + 1 mg L−1 GO when compared to their pristine forms. Furthermore, independent modeling assessment confirmed that the combination of nTiO2 and GO exhibited an additive mode of interactions with Chlorella sp., regardless of concentration and the interaction medium (Table 1). Notably, all the test groups in LW exhibited significant growth inhibition of Chlorella sp. compared to the corresponding test groups in BG-11.
Table 1 Independent action model for the combinations of nTiO2 and GO
Media |
Contaminants (mg L−1) |
Observed toxicity (%) |
Expected toxicity (%) |
Ratio of inhibition (RI) |
Significance |
Nature of action |
LW |
GO 0.1 + nTiO2 0.1 |
19.2 |
25.9 |
0.7 |
p > 0.05 |
Additive |
GO 0.1 + nTiO2 1 |
39.6 |
34.3 |
1.1 |
p > 0.05 |
Additive |
GO 1 + nTiO2 0.1 |
29.5 |
38.9 |
0.7 |
p > 0.05 |
Additive |
GO 1 + nTiO2 1 |
53.6 |
45.8 |
1.1 |
p > 0.05 |
Additive |
BG-11 |
GO 0.1 + nTiO2 0.1 |
14.7 |
19.1 |
0.7 |
p > 0.05 |
Additive |
GO 0.1 + nTiO2 1 |
34.5 |
29.8 |
1.1 |
p > 0.05 |
Additive |
GO 1 + nTiO2 0.1 |
24.3 |
28.2 |
0.8 |
p > 0.05 |
Additive |
GO 1 + nTiO2 1 |
47.1 |
37.7 |
1.2 |
p > 0.05 |
Additive |
These findings suggest that the mode of action for nTiO2 and GO may vary from one another. The combination with a high concentration of nTiO2 (1 mg L−1) and a low concentration (0.1 mg L−1) of GO exhibited higher growth inhibition than the mixture with a low concentration of nTiO2 (0.1 mg L−1) and a high concentration (1 mg L−1) of GO. In scenarios where the concentration of nTiO2 is high, there is an increased chance of physical damage to algal cells, compounded by light inhibition from GO.63,64 Conversely, higher concentrations of GO are associated with intensified light inhibition, along with direct damage by nTiO2, leading to subsequent growth inhibition.65 Notably, in the combination of high concentrations of both nTiO2 (1 mg L−1) and GO (1 mg L−1), both physical damage and light inhibition may have jointly contributed to the highest level of growth inhibition. This increase in combined toxicity could be ascribed to the additive impact exerted by nTiO2 and GO on Chlorella sp. in both BG-11 and LW. In a previous study, the combinations of ZnO NPs and GO at concentrations corresponding to their EC10 and EC50 values demonstrated more growth inhibition than their pristine forms on exposure to freshwater algae Scenedesmus obliquus.22 Furthermore, they reported an additive mode of interaction between ZnO NPs and GO when exposed to Scenedesmus obliquus.
As expected, test groups in the BG-11 medium exhibited less growth inhibition when compared to the corresponding test groups in the LW medium. This difference could be attributed to the “cushioning effect” of BG-11 media, as it possesses crucial nutritional components required for algal growth. Sharma et al., 2016 reported that BG-11 medium enhanced the growth and biomass of Chlorella sp. when compared to other defined culture media like M4N, BBM, and Fog's medium.66
3.2.1. Shading effect.
The shading effect of pristine GO, pristine nTiO2, and their combination on Chlorella sp. under BG-11 and LW conditions are depicted in Fig. 2(C and D). All the test groups exhibited (p < 0.001) significant shading effect when compared to the control group in both LW and BG-11 media. At the concentration of 0.1 mg L−1, pristine GO showed a significant (p < 0.001) increase in shading effect compared to pristine nTiO2 in both LW and BG-11 media. A similar pattern was observed between 1 mg L−1 pristine GO and 1 mg L−1 pristine nTiO2 in both LW and BG-11 media. In the combination experiments, the trend in the shading effect was similar for both BG-11 and LW. The trend is as follows: 1 mg L−1 nTiO2 + 1 mg L−1 GO > 0.1 mg L−1 nTiO2 + 1 mg L−1 GO > 1 mg L−1 nTiO2 + 0.1 mg L−1 GO > 0.1 mg L−1 nTiO2 + 0.1 mg L−1 GO. The mixture 0.1 mg L−1 nTiO2 + 0.1 mg L−1 GO exhibited a significant (P < 0.05) shading effect when compared to their pristine forms in both BG-11 and LW. Similarly, the mixture of 1 mg L−1 nTiO2 + 1 mg L−1 GO exhibited a highly significant (p < 0.001) shading effect when compared to their pristine forms in both BG-11 and LW. Notably, all the test groups in LW exhibited a significant shading effect in Chlorella sp. compared to the corresponding test groups in BG-11.
The aggregation of nTiO2 could impede light availability, thereby eliciting a shading effect on algal cells.35,38 Similarly, Yin et al., 2020 reported that the shading effect exhibited by GO is a primary factor in inducing growth inhibition in freshwater algae Scenedesmus obliquus.65 Interestingly, the pronounced shading effect exhibited by GO in comparison to nTiO2 may be ascribed to the sheet form of GO, being hydrophilic and displaying high opacity in its aggregated state.45,67 Moreover, introducing GO into the medium could exacerbate the algal settlement and hinder the light source.65 All the test groups in the BG-11 medium showed less shading effect when compared to the test sets in the LW medium. This could be attributed to the experimental conditions in shading effect studies, where contaminants are not directly introduced to algal cells. This situation might create scenarios where algal cells interacting in the BG-11 medium have improved nutritional availability compared to those in the LW medium.
3.3. Oxidative stress (ROS and MDA)
Fig. 3A and B illustrate the production of ROS in Chlorella sp. when exposed to pristine GO, pristine nTiO2, and their combination BG-11 and LW media, respectively. Fig. 3C and D show the levels of MDA in Chlorella sp. upon exposure to pristine GO, pristine nTiO2, and their combination in BG-11 and LW media, respectively. In contrast to the control group, a significant (p < 0.001) increase in the ROS generation and MDA levels was observed in all experimental groups, except for 0.1 mg L−1 of pristine GO (p > 0.05) and pristine nTiO2 (p > 0.05) in both LW and BG-11. It was observed that no significant difference (p > 0.05) in the ROS production was observed between pristine GO and pristine nTiO2 in both LW and BG-11 medium. A similar trend was observed in the MDA content estimation.
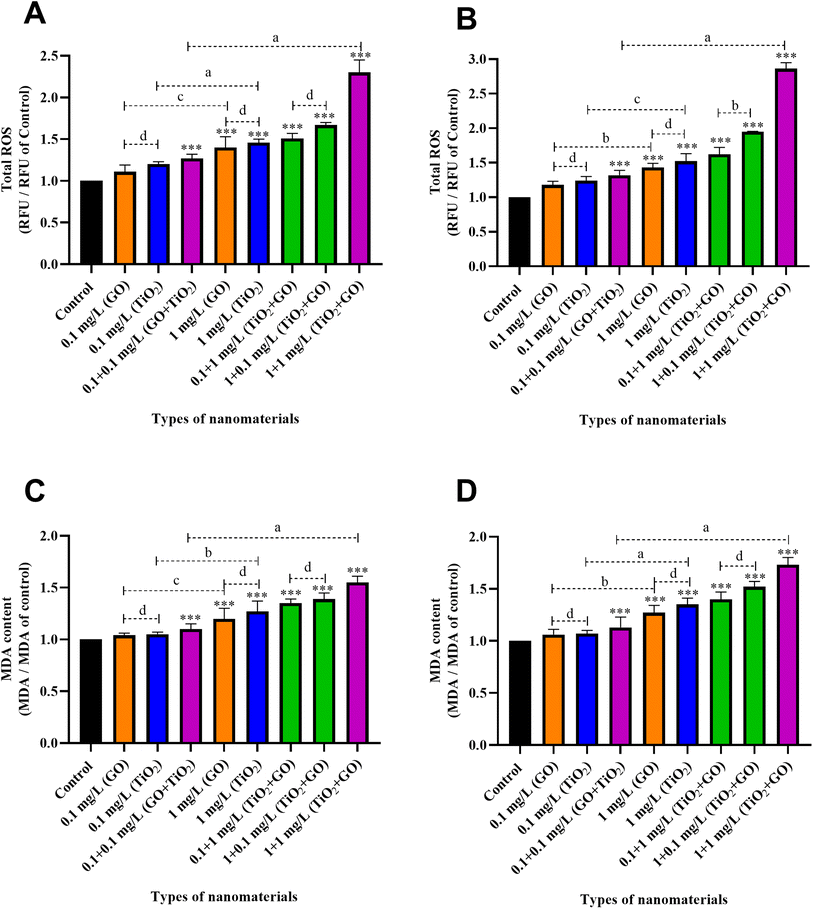 |
| Fig. 3 (A) ROS generation in Chlorella sp on exposure to pristine nTiO2 and pristine GO and their combination in BG-11 medium (B) under lake water (C) MDA levels in BG-11 medium as interaction (D) MDA levels in lake water. Note: The symbol ‘***’ denotes significance between test and control groups; a, b, c, and d indicate the significant difference between the groups (a = p < 0.001, b = p < 0.01, c = p < 0.05, and d = p > 0.05 (no significance)). | |
In the combination experiments, the trend observed for ROS generation and MDA levels was similar in both BG-11 and LW medium. The trend followed: 1 mg L−1 nTiO2 + 1 mg L−1 GO > 1 mg L−1 nTiO2 + 0.1 mg L−1 GO > 0.1 mg L−1 nTiO2 + 1 mg L−1 GO > 0.1 mg L−1 nTiO2 + 0.1 mg L−1 GO. In both the interaction media, the mixture of 0.1 mg L−1 nTiO2 + 0.1 mg L−1 GO did not exhibit a significant (p > 0.05) increase in ROS generation when compared to their pristine forms. However, 1 mg L−1 nTiO2 + 1 mg L−1 GO showed significant (p < 0.001) ROS production compared to their pristine forms in BG-11 and LW. A comparable pattern was observed in the MDA estimation. All the test groups in LW exhibited a significant ROS generation and MDA production in Chlorella sp. compared to the corresponding test groups in BG-11.
Lipids, playing a crucial role as fundamental components of the cellular membrane, represent the primary focal points of ROS. These ROS initiate lipid peroxidation by abstracting hydrogen from the unsaturated chains of fatty acids.68 Malondialdehyde (MDA) serves as an indicative marker, disclosing the degree of oxidative damage occurring in algal cells.69 The production of ROS and the subsequent increase in MDA content in Chlorella sp. upon interaction with nTiO2 can primarily be ascribed to the photocatalytic properties of nTiO2.70 Similarly, GO tends to attach on algal cell surfaces, creating a hypoxic environment that could directly enhance ROS and MDA production.9,14 However, the negligible ROS production and MDA levels upon interaction with 0.1 mg L−1 of both nTiO2 and GO could be ascribed to the inherent tolerance capacity of Chlorella sp. towards NMs at low concentrations. In a previous study, Roy et al., 2016 documented the negligible toxicity exhibited by 0.1 mg L−1 of nTiO2 in freshwater microalgae Chlorella sp.34 Similarly, Yan et al., 2022 reported that exposure of Microcystis aeruginosa to 0.1 mg L−1 of GO did not exhibit significant production ROS and MDA content.14
The mixture of 1 mg L−1 nTiO2 + 0.1 mg L−1 GO generated more ROS and MDA compared to 0.1 mg L−1 nTiO2 + 1 mg L−1 GO. This could be due to the higher availability of nTiO2 to the algal cells in the presence of 0.1 mg L−1 GO. The combination of GO and nTiO2 exhibited enhanced ROS and MDA production. This could be attributed to the propensity of GO to adsorb nTiO2, which could have exhibited additive damage to the algal cells. Additionally, the FE-SEM image also confirms the tendency of GO to adsorb nTiO2. In a previous study, Li et al., 2015 reported that the cell wall damage by nTiO2 could be a possible reason for the generation of ROS in Pichia pastoris.71 The highest ROS production in the mixture of 1 mg L−1 nTiO2 + 1 mg L−1 GO could be due to the independent ROS-inducing mechanism exhibited by nTiO2 and GO. The potential of nTiO2 to induce oxidative stress may serve as a primary mechanism for toxicity in algae.72 Similarly, reports suggested that the principal mechanism posited to explicate the toxicity of GO is oxidative stress.73 As the analysis of MDA content aligns with the pattern observed in ROS, it distinctly indicates ROS-induced lipid peroxidation.45,74 The test groups in LW media exhibited more oxidative stress than those in the BG-11 medium. In addition to the lack of cushioning effect, the heightened stability of the materials (nTiO2, GO, and their mixtures) in LW could be attributed to more oxidative stress than BG-11. Nanomaterials that exhibit enhanced stability are more likely to directly interact with algal cells, leading to more stress and subsequent oxidative stress.75 Conversely, in the case of less stable nanomaterials, the higher aggregation rate diminishes the chance of direct interaction with algal cells, resulting in reduced oxidative stress.74,76
3.4. PS(II) maximum quantum efficiency (Y(II)) and electron transport rate (ETR)
Fig. 4A and B reveals the changes in the PS(II) maximum quantum efficiency (Y(II)) of Chlorella sp. on exposure to pristine GO, pristine nTiO2, and their mixtures in BG-11 media (Fig. 4A) as well as LW (Fig. 4B). When compared to the control cells, all the treated cells in both the media showed the significant decline (p < 0.001). In this study, a significant reduction (p < 0.001) in the Y(II) and ETR (Fig. 4C (BG-11) and 4D (LW)) was observed in the cells treated with pristine GO than with pristine nTiO2, regardless of the concentration and the interaction medium.
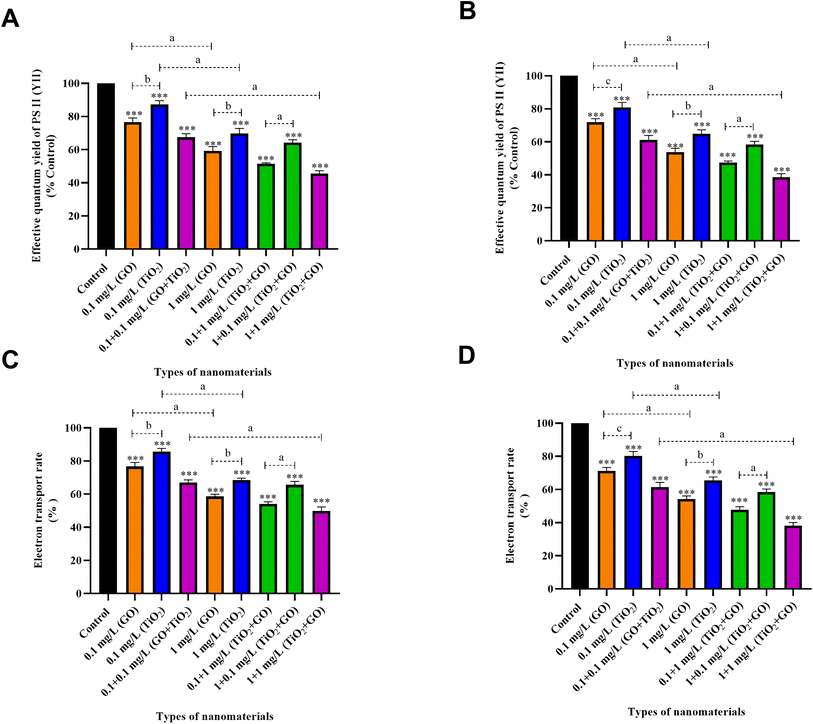 |
| Fig. 4 Photosynthetic parameters in treated algal cells were evaluated. (A and C) The comparison of effective quantum yield of PS II (Y II) and electron transport rate (ETR) of control and treated cells in LW media; (B and D) The comparison of effective quantum yield of PS II (Y II), and electron transport rate (ETR) of control and treated cells in BG-11 media. Note: The level of significance compared to control is presented by ‘***’ (p < 0.001); a, b, c, and d indicate the significant difference between the groups (a = p < 0.001, b = p < 0.01, c = p < 0.05, and d = p > 0.05 (no significance)). | |
The mixture 0.1 mg L−1 nTiO2 + 0.1 mg L−1 GO exhibited a significant (P < 0.05) reduction in Y(II) and ETR when compared to their pristine forms in both BG-11 and LW. Similarly, the mixture of 1 mg L−1 nTiO2 + 1 mg L−1 GO exhibited a significant (p < 0.001) reduction in Y(II) and ETR when compared to their pristine forms in both BG-11 and LW. Notably, all the test groups in LW exhibited reduced Y(II) and ETR values in Chlorella sp. compared to the corresponding test groups in BG-11.
Photosynthesis is a fundamental biological process in algae, where light energy is converted into chemical energy.77 The decrease in Y(II) and ETR values indicates an impairment in electron transport and photosynthesis, resulting from damage to the reaction center of photosystem II (PS II).78 Das et al., 2022 reported that exposure of Scnedesmus obliquus with nTiO2 at concentrations of 0.025, 0.25, and 2.5 mg L−1 substantially reduced the ETR and maximum quantum yield of PS II.79 Similarly, exposure of freshwater microalgae Euglena gracilis to GO at 0.1 and 1 mg L−1 concentrations reduced the effective photochemical quantum yield of PS II (Fv/Fm).80 The substantial reduction of Y(II) and ETR in combined toxicity experiments could be due to the excessive ROS production by nTiO2 coupled with the light shading effects of GO.81,82 Excessive ROS production in algal cells possesses the ability to damage photosynthetic apparatus.83 The carbon nanomaterials such as GO, upon aggregation, become opaque, which can hinder the passage of light to the algal cells.62 Moreover, direct attachment GO to the algal cell surface resulted in reduced photosynthetic activity.84
The BG-11 medium, as described by Pandey et al. in 2023, is instrumental in promoting the growth of photoautotrophic blue-green algae.85 This medium contains carbon sources, synthetic nitrogen, and other inorganic salts, which help in energy production and cellular development of the microalgae, as highlighted in the study by Dolganyuk et al. in 2020.86 Nitrogen, in particular, is a crucial component that plays a key role in improving algal biochemical activities and physiology. Its absence directly impedes photosynthetic activity, as noted in the studies by Perera et al. in 2022 and Fattore et al. in 2021.87,88 In the present study, it was observed that the freshwater Chlorella sp. had better survival and there was less reduction in PS II efficiency and ETR. This may be attributed to the availability of synthetic nitrogen and other elements in the BG-11 medium, which potentially facilitated and enhanced their growth as compared to lake water.
3.5. Mechanism of toxicity and Co-relation analysis
The potential toxicity mechanisms of pristine nTiO2 and pristine graphene oxide (GO) on Chlorella sp. may arise from direct cell damage and increased ROS production, attributed to their inherent photocatalytic properties. Consequently, exposure to pristine nTiO2 and pristine GO led to a significant increase in MDA levels. Notably, pristine GO exhibited a more substantial shading effect compared to pristine nTiO2, which was accompanied by a decrease in maximum quantum yield and electron transport rate. This difference could be attributed to the physical structure of GO, composed of sheets, which potentially impedes light transmission to the algal cells in contrast to nTiO2 NPs.
In combination, the toxicity mechanism towards algal cells varies as the concentration of the pollutant changes. The combinations of 1 mg L−1 nTiO2 and 0.1 mg L−1 of GO exhibited enhanced toxic effects compared to those with 0.1 mg L−1 of nTiO2 and 1 mg L−1 of GO. This discrepancy could be due to the distinct modes of action of the pollutants, wherein 1 mg L−1 of nTiO2 may result in heightened physical damage to algal cells alongside light inhibition caused by GO (0.1 mg L−1). Similarly, 1 mg L−1 of GO may induce intensified light inhibition on algal cells in addition to direct damage caused by nTiO2 (0.1 mg L−1). Remarkably, in the mixture of nTiO2 (1 mg L−1) and GO (1 mg L−1), both physical damage and light inhibition may jointly contribute to exhibiting substantial toxic effects on Chlorella sp.
The graphical representation of the biological parameters in the form of Heatmap in Fig. 5A-i (for LW) and 5B-i (for BG-11) reveals that the relative growth inhibition increased in the binary mixture groups when compared to pristine. A similar observation was noted for the shading effect, total ROS, MDA content, the maximum effective quantum yield of Y II (PS II), and ETR. The Pearson correlation mapping was utilized to analyze the correlation between all the biological parameters in LW (Fig. 5A-ii) and BG-11 (Fig. 5B-ii). In both media, a notable positive correlation exists among growth inhibition, shading effect, total ROS, and MDA content, which is statistically significant. Meanwhile, a negative correlation was observed for growth inhibition, shading effect, total ROS, and MDA content when compared to Y II (PS II) and ETR. Furthermore, a positive correlation was also noted between Y II (PS II) and ETR parameters. The level of significant correlation among the different parameters was represented by ‘*’ (*** (highly significant) = p < 0.001; ** (moderately significant) = p < 0.01; and * (less significant) = p < 0.05). In this study, it was observed that as the growth inhibition increased, the levels of ROS and MDA also increased. This trend was also reflected in correlation analysis. The correlation analysis by Liu et al. 2023 showed a similar pattern where the oxide nanoparticle-induced greater growth inhibition by generating ROS and MDA content.89 A decline in the total chlorophyll content was also observed simultaneously with the increase in ROS production.89 The current study followed a similar Y II (PS II) and ETR reduction trend.
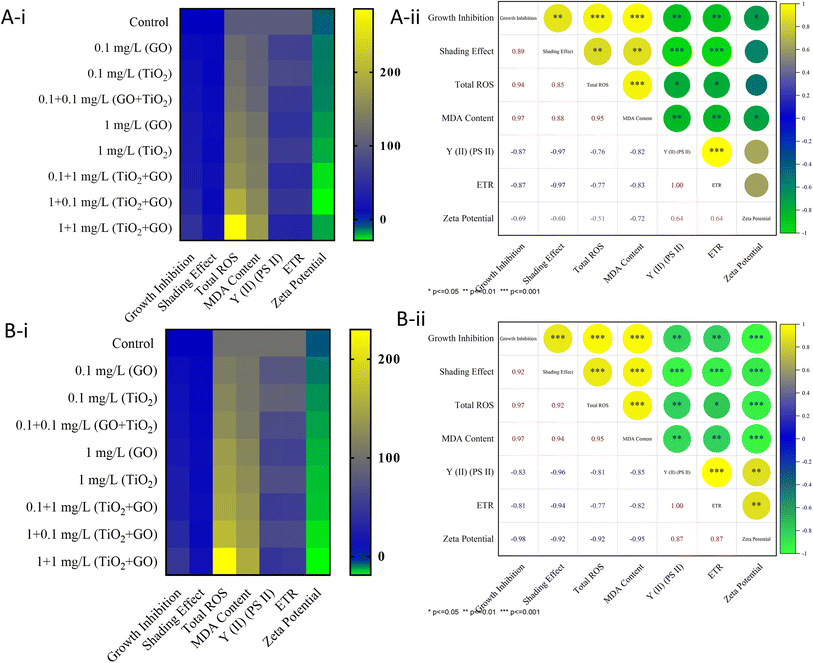 |
| Fig. 5 Heatmap (5 A-i (LW); B-i (BG-11)) and the correlation analysis plots (5 A-ii (LW); B-ii (BG-11)) between the parameters in all treatment groups (* = p < 0.05; ** = p < 0.01; *** = p < 0.001). | |
4 Conclusion
This investigation shows that the combination of nTiO2 and GO elicits more pronounced toxic effects than their pristine forms. Furthermore, it was observed that the nature of the interaction medium significantly influences the modulation of nanomaterial toxicity on Chlorella sp. Most toxicity assessments involved freshwater algae as the model organism, utilizing the defined culture medium as the medium of interaction. Employing environmentally relevant conditions and mediums is recommended to evaluate and understand the ecotoxicological effects of nanomaterials. Future research endeavors could delve into the potential risks posed by carbon materials, in combination with other aquatic pollutants in higher organisms, and their implications in trophic transfer.
Conflicts of interest
There are no conflicts to declare.
References
- F. Albalawi, M. Z. Hussein, S. Fakurazi and M. J. Masarudin, Engineered nanomaterials: The challenges and opportunities for nanomedicines, Int. J. Nanomed., 2021, 161–184 CrossRef PubMed.
- C. J. Dedman, A. M. King, J. A. Christie-Oleza and G.-L. Davies, Environmentally relevant concentrations of titanium dioxide nanoparticles pose negligible risk to marine microbes, Environ. Sci.: Nano, 2021, 8, 1236–1255 RSC.
- X. Li, Q. Ma, T. Liu, Z. Dong and W. Fan, Effect of TiO2-nanoparticles on copper toxicity to bacteria: Role of bacterial surface, RSC Adv., 2020, 10, 5058–5065 RSC.
- Z. Luo, Z. Li, Z. Xie, I. M. Sokolova, L. Song, W. J. G. M. Peijnenburg, M. Hu and Y. Wang, Rethinking nano-TiO2 safety: overview of toxic effects in humans and aquatic animals, Small, 2020, 16, 2002019 CrossRef CAS PubMed.
- M. M. Rashid, P. Forte Tavčer and B. Tomšič, Influence of titanium dioxide nanoparticles on human health and the environment, Nanomaterials, 2021, 11, 2354 CrossRef CAS PubMed.
- B. Xia, L. Zhu, Q. Han, X. Sun, B. Chen and K. Qu, Effects of TiO2 nanoparticles at predicted environmental relevant concentration on the marine scallop Chlamys farreri: An integrated biomarker approach, Environ. Toxicol. Pharmacol., 2017, 50, 128–135 CrossRef CAS PubMed.
- A. Georgantzopoulou, P. Almeida Carvalho, C. Vogelsang, M. Tilahun, K. Ndungu, A. M. Booth, K. V Thomas and A. Macken, Ecotoxicological effects of transformed silver and titanium dioxide nanoparticles in the effluent from a lab-scale wastewater treatment system, Environ. Sci. Technol., 2018, 52, 9431–9441 CrossRef CAS PubMed.
- V. N. Haynes, J. E. Ward, B. J. Russell and A. G. Agrios, Photocatalytic effects of titanium dioxide nanoparticles on aquatic organisms—Current knowledge and suggestions for future research, Aquat. Toxicol., 2017, 185, 138–148 CrossRef CAS PubMed.
- J. Yin, W. Fan, J. Du, W. Feng, Z. Dong, Y. Liu and T. Zhou, The toxicity of graphene oxide affected by algal physiological characteristics: A comparative study in cyanobacterial, green algae, diatom, Environ. Pollut., 2020, 260, 113847 CrossRef CAS PubMed.
- D. P. Singh, C. E. Herrera, B. Singh, S. Singh, R. K. Singh and R. Kumar, Graphene oxide: An efficient material and recent approach for biotechnological and biomedical applications, Mater. Sci. Eng., C, 2018, 86, 173–197 CrossRef CAS PubMed.
- A. Jiříčková, O. Jankovský, Z. Sofer and D. Sedmidubský, Synthesis and applications of graphene oxide, Materials, 2022, 15, 920 CrossRef PubMed.
- J. Ali, Y. Li, E. Shang, X. Wang, J. Zhao, M. Mohiuddin and X. Xia, Aggregation of graphene oxide and its environmental implications in the aquatic environment, Chin. Chem. Lett., 2023, 34, 107327 CrossRef CAS.
- A. Pires, E. Figueira, M. S. S. Silva, C. Sá and P. A. A. P. Marques, Effects of graphene oxide nanosheets in the polychaete Hediste diversicolor: Behavioural, physiological and biochemical responses, Environ. Pollut., 2022, 299, 118869 CrossRef CAS PubMed.
- Z. Yan, X. Yang, I. Lynch and F. Cui, Comparative evaluation of the mechanisms of toxicity of graphene oxide and graphene oxide quantum dots to blue-green algae Microcystis aeruginosa in the aquatic environment, J. Hazard. Mater., 2022, 425, 127898 CrossRef CAS PubMed.
- J. Wang, X. Zhu, L. Tan, T. Zhao, Z. Ni, N. Zhang and J. Wang, Single and combined nanotoxicity of ZnO nanoparticles and graphene quantum dots against the microalga Heterosigma akashiwo, Environ. Sci.: Nano, 2022, 9, 3094–3109 RSC.
- R. F. Lehutso, Y. Tancu, A. Maity and M. Thwala, Aquatic toxicity of transformed
and product-released engineered nanomaterials: An overview of the current state of knowledge, Process Saf. Environ., 2020, 138, 39–56 CrossRef CAS.
- Z. Guo, J. Zuo, J. Feng, J. Li, S. Zhang and K. Ma, Impact of Titanium Dioxide-Graphene Oxide (TiO2-GO) Composite Nanoparticle on the Juveniles of the Giant River Prawn, Macrobrachium rosenbergii: Physio-Biochemistry and Transcriptional Response, Mar. Biotechnol., 2023, 25, 45–56 CrossRef CAS PubMed.
- O. Suárez-Iglesias, S. Collado, P. Oulego and M. Díaz, Graphene-family nanomaterials in wastewater treatment plants, J. Chem. Eng., 2017, 313, 121–135 CrossRef.
- S. Simelane and L. N. Dlamini, An investigation of the fate and behaviour of a mixture of WO3 and TiO2 nanoparticles in a wastewater treatment plant, J. Environ. Sci., 2019, 76, 37–47 CrossRef PubMed.
- Y. Deng, J. Li, M. Qiu, F. Yang, J. Zhang and C. Yuan, Deriving characterization factors on freshwater ecotoxicity of graphene oxide nanomaterial for life cycle impact assessment, Int. J. Life Cycle Assess., 2017, 22, 222–236 CrossRef CAS.
- X. Shi, Z. Li, W. Chen, L. Qiang, J. Xia, M. Chen, L. Zhu and P. J. J. Alvarez, Fate of TiO2 nanoparticles entering sewage treatment plants and bioaccumulation in fish in the receiving streams, NanoImpact, 2016, 3–4, 96–103 CrossRef.
- N. Ye, Z. Wang, S. Wang and W. J. G. M. Peijnenburg, Toxicity of mixtures of zinc oxide and graphene oxide nanoparticles to aquatic organisms of different trophic level: particles outperform dissolved ions, Nanotoxicology, 2018, 12, 423–438 CrossRef CAS PubMed.
- M. A. Irshad, R. Nawaz, M. Z. Ur Rehman, M. Adrees, M. Rizwan, S. Ali, S. Ahmad and S. Tasleem, Synthesis, characterization and advanced sustainable applications of titanium dioxide nanoparticles: A review, Ecotoxicol. Environ. Saf., 2021, 212, 111978 CrossRef CAS PubMed.
- X. Liu, P. Song, R. Lan, R. Zhao, R. Xue, J. Zhao and B. Xing, Heteroaggregation between graphene oxide and titanium dioxide particles of different shapes in aqueous phase, J. Hazard. Mater., 2022, 428, 128146 CrossRef CAS PubMed.
- S. Das, V. Thiagarajan, N. Chandrasekaran, B. Ravindran and A. Mukherjee, Nanoplastics enhance the toxic effects of titanium dioxide nanoparticle in freshwater algae Scenedesmus obliquus, Comp. Biochem. Physiol., Part C: Toxicol. Pharmacol., 2022, 256, 109305 CAS.
- A. E. Marchello, D. M. Barreto and A. T. Lombardi, Effects of titanium dioxide nanoparticles in different metabolic pathways in the freshwater microalga Chlorella sorokiniana (Trebouxiophyceae), Water, Air, Soil Pollut., 2018, 229, 1–11 CrossRef CAS.
- J. Ding, S. Zhang, R. M. Razanajatovo, H. Zou and W. Zhu, Accumulation, tissue distribution, and biochemical effects of polystyrene microplastics in the freshwater fish red tilapia (Oreochromis niloticus), Environ. Pollut., 2018, 238, 1–9 CrossRef CAS PubMed.
- V. Nava and B. Leoni, A critical review of interactions between microplastics, microalgae and aquatic ecosystem function, Water Res., 2021, 188, 116476 CrossRef CAS PubMed.
- C. Adochite and L. Andronic, Aquatic toxicity of photocatalyst nanoparticles to green microalgae Chlorella vulgaris, Water, 2020, 13, 77 CrossRef.
- L. A. Millington, K. H. Goulding and N. Adams, The influence of growth medium composition on the toxicity of chemicals to algae, Water Res., 1988, 22, 1593–1597 CrossRef CAS.
- I. V. N. Rathnayake, M. Megharaj, M. Beer and R. Naidu, Medium composition affects the heavy metal tolerance of microalgae: a comparison, J. Appl. Phycol., 2021, 33, 3683–3695 CrossRef CAS.
- A. F. Aravantinou, V. Tsarpali, S. Dailianis and I. D. Manariotis, Effect of cultivation media on the toxicity of ZnO nanoparticles to freshwater and marine microalgae, Ecotoxicol. Environ. Saf., 2015, 114, 109–116 CrossRef CAS PubMed.
- A. AL-Ammari, L. Zhang, J. Yang, F. Wei, C. Chen and D. Sun, Toxicity assessment of synthesized titanium dioxide nanoparticles in fresh water algae Chlorella pyrenoidosa and a zebrafish liver cell line, Ecotoxicol. Environ. Saf., 2021, 211, 111948 CrossRef CAS PubMed.
- R. Roy, A. Parashar, M. Bhuvaneshwari, N. Chandrasekaran and A. Mukherjee, Differential effects of P25 TiO2 nanoparticles on freshwater green microalgae: Chlorella and Scenedesmus species, Aquat. Toxicol., 2016, 176, 161–171 CrossRef CAS PubMed.
- M. Sendra, I. Moreno-Garrido, M. P. Yeste, J. M. Gatica and J. Blasco, Toxicity of TiO2 in nanoparticle or bulk form to freshwater and marine microalgae under visible light and UV-A radiation, Environ. Pollut., 2017, 227, 39–48 CrossRef CAS PubMed.
- Y. Liu, S. Wang, Z. Wang, N. Ye, H. Fang and D. Wang, TiO2, SiO2 and ZrO2 nanoparticles synergistically provoke cellular oxidative damage in freshwater microalgae, Nanomaterials, 2018, 8, 95 CrossRef PubMed.
- Z. Li, P. Juneau, Y. Lian, W. Zhang, S. Wang, C. Wang, L. Shu, Q. Yan, Z. He and K. Xu, Effects of titanium dioxide nanoparticles on photosynthetic and antioxidative processes of Scenedesmus obliquus, Plants, 2020, 9, 1748 CrossRef CAS PubMed.
- A. E. Marchello, D. M. Barreto and A. T. Lombardi, Effects of titanium dioxide nanoparticles in different metabolic pathways in the freshwater microalga Chlorella sorokiniana (Trebouxiophyceae), Water, Air, Soil Pollut., 2018, 229, 1–11 CrossRef CAS.
- A. Middepogu, J. Hou, X. Gao and D. Lin, Effect and mechanism of TiO2 nanoparticles on the photosynthesis of Chlorella pyrenoidosa, Ecotoxicol. Environ. Saf., 2018, 161, 497–506 CrossRef CAS PubMed.
- J. Zhang, X. Xie, Q. Li, J. Wang and S. Zhang, Combined toxic effects of TiO2 nanoparticles and organochlorines on Chlorella pyrenoidosa in karst area natural waters, Aquat. Toxicol., 2023, 257, 106442 CrossRef CAS PubMed.
- C. Rex M and A. Mukherjee, The comparative effects of visible light and UV-A radiation on the combined toxicity of P25 TiO2 nanoparticles and polystyrene microplastics on Chlorella sp, Environ. Sci. Pollut. Res. Int., 2023, 30, 122700–122716 CrossRef CAS PubMed.
- S. Ouyang, X. Hu and Q. Zhou, Envelopment–Internalization Synergistic Effects and Metabolic Mechanisms of Graphene Oxide on Single-Cell Chlorella vulgaris Are Dependent on the Nanomaterial Particle Size, ACS Appl. Mater. Interfaces, 2015, 7, 18104–18112 CrossRef CAS PubMed.
- X. Ji, X. Li, S. Wu, M. Hou and Y. Zhao, Effects of graphene oxide on algal cellular stress response: Evaluating metabolic characters of carbon fixation and nutrient removal, Chemosphere, 2020, 252, 126566 CrossRef CAS PubMed.
- K. Y. Kim, S. M. Kim, J. Y. Kim and Y.-E. Choi, Elucidating the mechanisms underlying the cytotoxic effects of nano-/micro-sized graphene oxide on the microalgae by comparing the physiological and morphological changes in different trophic modes, Chemosphere, 2022, 309, 136539 CrossRef CAS PubMed.
- J. Zhao, X. Cao, Z. Wang, Y. Dai and B. Xing, Mechanistic understanding toward the toxicity of graphene-family materials to freshwater algae, Water Res., 2017, 111, 18–27 CrossRef CAS PubMed.
- P. F. M. Nogueira, D. Nakabayashi and V. Zucolotto, The effects of graphene oxide on green algae Raphidocelis subcapitata, Aquat. Toxicol., 2015, 166, 29–35 CrossRef CAS PubMed.
- S. Das, N. Chandrasekaran and A. Mukherjee, The effects of graphene oxide on green algae Raphidocelis subcapitata, Comp. Biochem. Physiol., Part C: Toxicol. Pharmacol., 2023, 267, 109587 CAS.
- B. Roy, P. K. Suresh, N. Chandrasekaran and A. Mukherjee, Antibiotic tetracycline enhanced the toxic potential of photo catalytically active P25 titanium dioxide nanoparticles towards freshwater algae Scenedesmus obliquus, Chemosphere, 2021, 267, 128923 CrossRef CAS PubMed.
- I. M. Sadiq, S. Dalai, N. Chandrasekaran and A. Mukherjee, Ecotoxicity study of titania (TiO2) NPs on two microalgae species: Scenedesmus sp. and Chlorella sp, Ecotoxicol. Environ. Saf., 2011, 74, 1180–1187 CrossRef CAS PubMed.
- S. Das, S. Giri, S. A. Jose, M. Pulimi, S. Anand, N. Chandrasekaran, P. K. Rai and A. Mukherjee, Comparative toxicity assessment of individual, binary and ternary mixtures of SiO2, Fe3O4, and ZnO nanoparticles in freshwater microalgae, Scenedesmus obliquus: Exploring the role of dissolved ions, Comp. Biochem. Physiol., Part C: Toxicol. Pharmacol., 2023, 273, 109718 CAS.
-
OECD, Proposal for Updating Guideline 201 Freshwater Alga and Cyanobacteria, Growth Inhibition Test, OECD Guideline for Testing of Chemicals, 2002, 1–21 Search PubMed.
- S. Giri and A. Mukherjee, Ageing with algal EPS reduces the toxic effects of polystyrene nanoplastics in freshwater microalgae Scenedesmus obliquus, J. Environ. Chem. Eng., 2021, 9, 105978 CrossRef CAS.
- L. Natarajan, M. Annie Jenifer, W. J. G. M. Peijnenburg and A. Mukherjee, Algal extracellular polymeric substances (algal-EPS) for mitigating the combined toxic effects of polystyrene nanoplastics and nano-TiO2 in Chlorella sp, Nanotoxicology, 2023, 17, 143–156 CrossRef CAS PubMed.
- J. Zhao, X. Cao, Z. Wang, Y. Dai and B. Xing, Mechanistic understanding toward the toxicity of graphene-family materials to freshwater algae, Water Res., 2017, 111, 18–27 CrossRef CAS PubMed.
- V. Thiagarajan, S. Ramasubbu, C. Natarajan and A. Mukherjee, Differential sensitivity of marine algae Dunaliella salina and Chlorella sp. to P25 TiO2 NPs, Environ. Sci. Pollut. Res. Int., 2019, 26, 21394–21403 CrossRef CAS PubMed.
- J. W. Lee, S. H. Lee, J. W. Han and G. H. Kim, Early Light-Inducible Protein (ELIP) Can Enhance Resistance to Cold-Induced Photooxidative Stress in Chlamydomonas reinhardtii, Front. Physiol., 2020, 11, 545235 Search PubMed.
- A. Debroy, N. Roy, S. Giri, M. Pulimi, N. Chandrasekaran, W. J. G. M. Peijnenburg and A. Mukherjee, EPS-corona formation on graphene family nanomaterials (GO, rGO and graphene) and its role in mitigating their toxic effects in the marine alga, Environ. Pollut., 2024, 341, 123015 CrossRef CAS PubMed.
- V. Thiagarajan, L. Natarajan, R. Seenivasan, N. Chandrasekaran and A. Mukherjee, Tetracycline affects the toxicity of P25 n-TiO2 towards marine microalgae Chlorella sp, Environ. Res., 2019, 179, 108808 CrossRef CAS PubMed.
- Z. Zhu, F. Zhou, S. Zhan, Y. Tian and Q. He, Study on the bactericidal performance of graphene/TiO2 composite photocatalyst in the coating of PEVE, Appl. Surf. Sci., 2018, 430, 116–124 CrossRef CAS.
- N. Qi, P. Wang, C. Wang and Y. Ao, Effect of a typical antibiotic (tetracycline) on the aggregation of TiO2 nanoparticles in an aquatic environment, J. Hazard. Mater., 2018, 341, 187–197 CrossRef PubMed.
- F. Chen, Z. Xiao, L. Yue, J. Wang, Y. Feng, X. Zhu, Z. Wang and B. Xing, Algae response to engineered nanoparticles: current understanding, mechanisms and implications, Environ. Sci.: Nano, 2019, 6, 1026–1042 RSC.
- L. J. Hazeem, M. Bououdina, E. Dewailly, C. Slomianny, A. Barras, Y. Coffinier, S. Szunerits and R. Boukherroub, Toxicity effect of graphene oxide on growth and photosynthetic pigment of the marine alga Picochlorum sp. during different growth stages, Environ. Sci. Pollut. Res. Int., 2017, 24, 4144–4152 CrossRef CAS PubMed.
- Y. Wang, X. Zhu, Y. Lao, X. Lv, Y. Tao, B. Huang, J. Wang, J. Zhou and Z. Cai, TiO2 nanoparticles in the marine environment: physical effects responsible for the toxicity on algae Phaeodactylum tricornutum, Sci. Total Environ., 2016, 565, 818–826 CrossRef CAS PubMed.
- J. Zhang, L. Jiang, D. Wu, Y. Yin and H. Guo, Effects of environmental factors on the growth and microcystin production of Microcystis aeruginosa under TiO2 nanoparticles stress, Sci. Total Environ., 2020, 734, 139443 CrossRef CAS PubMed.
- J. Yin, W. Fan, J. Du, W. Feng, Z. Dong, Y. Liu and T. Zhou, The toxicity of graphene oxide affected by algal physiological characteristics: A comparative study in cyanobacterial, green algae, diatom, Environ. Pollut., 2020, 260, 113847 CrossRef CAS PubMed.
- A. K. Sharma, P. K. Sahoo, S. Singhal and A. Patel, Impact of various media and organic carbon sources on biofuel production potential from Chlorella sp, 3 Biotech, 2016, 6, 1–12 Search PubMed.
- L. J. Hazeem, M. Bououdina, E. Dewailly, C. Slomianny, A. Barras, Y. Coffinier, S. Szunerits and R. Boukherroub, Toxicity effect of graphene oxide on growth and photosynthetic pigment of the marine alga Picochlorum sp. during different growth stages, Environ. Sci. Pollut. Res. Int., 2017, 24, 4144–4152 CrossRef CAS PubMed.
- M. Rezayian, V. Niknam and H. Ebrahimzadeh, Oxidative damage and antioxidative system in alga, Toxicol Rep, 2019, 6, 1309–1313 CrossRef CAS PubMed.
- A. B. Sikiru, A. Arangasamy, I. C. Alemede, S. S. A. Egena and R. Bhatta, Dietary supplementation effects of Chlorella vulgaris on performances, oxidative stress status and antioxidant enzymes activities of prepubertal New Zealand White rabbits, Bull. Natl. Res. Cent., 2019, 43, 1–7 CrossRef.
- M. M. Matouke, D. T. Elewa and K. Abdullahi, Binary effect of titanium dioxide nanoparticles (nTiO2) and phosphorus on microalgae (Chlorella ‘Ellipsoides Gerneck, 1907), Aquat. Toxicol., 2018, 198, 40–48 CrossRef CAS PubMed.
- F. Li, Z. Liang, X. Zheng, W. Zhao, M. Wu and Z. Wang, Toxicity of nano-TiO2 on algae and the site of reactive oxygen species production, Aquat. Toxicol., 2015, 158, 1–13 CrossRef CAS PubMed.
- B. Xia, B. Chen, X. Sun, K. Qu, F. Ma and M. Du, Interaction of TiO2 nanoparticles with the marine microalga Nitzschia closterium: growth inhibition, oxidative stress and internalization, Sci. Total Environ., 2015, 508, 525–533 CrossRef CAS PubMed.
- I. Martín-de-Lucía, M. C. Campos-Mañas, A. Agüera, F. Leganés, F. Fernández-Piñas and R. Rosal, Combined toxicity of graphene oxide and wastewater to the green alga Chlamydomonas reinhardtii, Environ. Sci.: Nano, 2018, 5, 1729–1744 RSC.
- D. Wu, S. Yang, W. Du, Y. Yin, J. Zhang and H. Guo, Effects of titanium dioxide nanoparticles on Microcystis aeruginosa and microcystins production and release, J. Hazard. Mater., 2019, 377, 1–7 CrossRef CAS PubMed.
- N. B. Turan, H. S. Erkan, G. O. Engin and M. S. Bilgili, Nanoparticles in the aquatic environment: Usage, properties, transformation and toxicity—A review, Process Saf. Environ. Prot., 2019, 130, 238–249 CrossRef CAS.
- L. Natarajan, M. A. Jenifer, N. Chandrasekaran, G. K. Suraishkumar and A. Mukherjee, Polystyrene nanoplastics diminish the toxic effects of Nano-TiO2 in marine algae Chlorella sp, Environ. Res., 2022, 204, 112400 CrossRef CAS PubMed.
- K. Suresh Kumar, H. U. Dahms, J. S. Lee, H. C. Kim, W. C. Lee and K. H. Shin, Algal photosynthetic responses to toxic metals and herbicides assessed by chlorophyll a fluorescence, Ecotoxicol. Environ. Saf., 2014, 104, 51–71 CrossRef CAS PubMed.
- A. Middepogu, J. Hou, X. Gao and D. Lin, Effect and mechanism of TiO2 nanoparticles on the photosynthesis of Chlorella pyrenoidosa, Ecotoxicol. Environ. Saf., 2018, 161, 497–506 CrossRef CAS PubMed.
- S. Das, V. Thiagarajan, N. Chandrasekaran, B. Ravindran and A. Mukherjee, Nanoplastics enhance the toxic effects of titanium dioxide nanoparticle in freshwater algae Scenedesmus obliquus, Comp. Biochem. Physiol., Part C: Toxicol. Pharmacol., 2022, 256, 109305 CAS.
- K. Y. Kim, S. M. Kim, J. Y. Kim and Y. E. Choi, Elucidating the mechanisms underlying the cytotoxic effects of nano-/micro-sized graphene oxide on the microalgae by comparing the physiological and morphological changes in different trophic modes, Chemosphere, 2022, 309, 136539 CrossRef CAS PubMed.
- Z. Li, P. Juneau, Y. Lian, W. Zhang, S. Wang, C. Wang, L. Shu, Q. Yan, Z. He and K. Xu, Effects of Titanium Dioxide Nanoparticles on Photosynthetic and Antioxidative Processes of Scenedesmus obliquus, Plants, 2020, 9, 1748 CrossRef CAS PubMed.
- Z. Yan, X. Yang, I. Lynch and F. Cui, Comparative evaluation of the mechanisms of toxicity of graphene oxide and graphene oxide quantum dots to blue-green algae Microcystis aeruginosa in the aquatic environment, J. Hazard. Mater., 2022, 425, 127898 CrossRef CAS PubMed.
- Z. Chen, S. Song, Y. Wen, Y. Zou and H. Liu, Toxicity of Cu(II) to the green alga Chlorella vulgaris: a perspective of photosynthesis and oxidant stress, Environ. Sci. Pollut. Res. Int., 2016, 23, 17910–17918 CrossRef CAS PubMed.
- L. J. Hazeem, M. Bououdina, E. Dewailly, C. Slomianny, A. Barras, Y. Coffinier, S. Szunerits and R. Boukherroub, Toxicity effect of graphene oxide on growth and photosynthetic pigment of the marine alga Picochlorum sp. during different growth stages, Environ. Sci. Pollut. Res. Int., 2017, 24, 4144–4152 CrossRef CAS PubMed.
- S. Pandey, I. Narayanan, R. Vinayagam and R. Selvaraj, Journal of Environmental Chemical Engineering A review on the effect of blue green 11 medium and its constituents on microalgal growth and lipid production, J. Environ. Chem. Eng., 2023, 11, 109984 CrossRef CAS.
- V. Dolganyuk, D. Belova, O. Babich, A. Prosekov, S. Ivanova, D. Katserov, N. Patyukov and S. Sukhikh, Microalgae: A promising source of valuable bioproducts, Biomolecules, 2020, 10, 1153 CrossRef CAS PubMed.
- I. A. Perera, S. Abinandan, S. R. Subashchandrabose, K. Venkateswarlu, R. Naidu and M. Megharaj, Combined inorganic nitrogen sources influence the release of extracellular compounds that drive mutualistic interactions in microalgal–bacterial co-cultures, J. Appl. Phycol., 2022, 34, 1311–1322 CrossRef CAS.
- N. Fattore, A. Bellan, L. Pedroletti, N. Vitulo and T. Morosinotto, Acclimation of photosynthesis and lipids biosynthesis to prolonged nitrogen and phosphorus limitation in Nannochloropsis gaditana, Algal Res., 2021, 58, 102368 CrossRef.
- Y. Liu, M. Kang, Y. Weng, Y. Ding and X. Bai, Toxicity and tolerance mechanism of binary zinc oxide nanoparticles and tetrabromobisphenol A regulated by humic acid in Chlorella vulgaris, Environ. Sci.: Processes Impacts, 2023, 25, 1615–1625 RSC.
|
This journal is © The Royal Society of Chemistry 2024 |