Efficient removal of per- and polyfluoroalkyl substances (PFASs) in drinking water treatment: nanofiltration combined with active carbon or anion exchange†
Received
6th April 2019
, Accepted 11th June 2019
First published on 26th June 2019
Abstract
Society's increasing use of chemicals poses a challenge for drinking water producers. Accepted concentrations for per- and polyfluoroalkyl substances (PFASs) in finished water are lower than ever before with new regulations often enacted based on findings made possible by improved analytical techniques and correspondingly justified health concerns. Nanomembrane filtration removes compounds, including PFASs, based primarily on size-exclusion, however, treatment and/or disposal of PFAS laden membrane concentrate remains a challenge. This study combined feedwater nanofiltration with granular activated carbon (GAC) and anion exchange (AIX) for concentrate treatment. Nanofiltration removed PFAS concentrations on average by 99% including some PFASs with molecular weights smaller than the membrane nominal cutoff of 270 Da, indicating membrane rejection mechanisms additional to size-exclusion. Treatment of raw water and concentrate was compared in column tests. AIX showed up to threefold greater half-time of saturation than GAC, however with a higher rate of decreasing efficiency, while GAC removed approximately 20% of incoming PFAS concentrations consistently after treatment of 15
000 bed volumes (BVs). Overall, GAC and AIX removed 2.6-fold and 4.1-fold more PFAS mass per adsorbent volume from the concentrated retentate than from raw water indicating that the combination of nanofiltration with GAC or AIX increases the efficiency of the adsorbent materials in comparison to only using GAC or AIX filters.
Water impact
Drinking water contaminated with per- and polyfluoroalkyl substances (PFASs) poses a health risk to affected consumers due to poor PFAS removal in conventional drinking water treatment plants. In our study, nanofiltration (NF) for drinking water treatment was combined with anion exchange (AIX) or granular activated carbon (GAC) for treatment of the NF waste stream. NF showed high removal efficiency for all PFASs (on average 99%). PFAS removal efficiencies were up to four-fold higher for the concentrated NF waste stream using AIX and GAC adsorption compared to the treatment of raw water. The combination of NF with AIX or GAC adsorbents offers drinking water providers reliable PFAS removal and the benefit of effective NF waste stream treatment using regenerable AIX and GAC materials.
|
1 Introduction
Per- and polyfluoroalkyl substances (PFASs) are a group of man-made chemicals with unique physico-chemical properties.1 Their ability to repel both oil and water in combination with their durability makes them chemicals of choice in a wide range of applications.1,2 PFASs are considered persistent and mobile chemicals as they show the ability to undergo long-range transport and several PFASs have been shown to be bioaccumulative and toxic, making them chemicals of very high concern.3–5 Contaminated ground and surface water has led to high PFAS concentrations in drinking water, with severe exposure of several communities.6–8 Conventional drinking water treatment often results in similar or even higher PFAS concentrations in finished drinking water compared to raw water, due to inefficient treatment techniques or PFAS precursor transformation by destructive methods.9,10 Removal of PFASs from raw drinking water therefore remains a major challenge and cost-efficient treatment methods have not been identified. Granular activated carbon (GAC) is a versatile adsorbent material that can be used to effectively remove PFASs from water if new or freshly regenerated.9,11,12 However, operation of GAC filters using typical regeneration operational cycles in drinking water production is unfavorable for PFAS removal which requires much shorter bed run times.11,13 Consequently, GAC filters have to be frequently replaced or regenerated if applied for effective PFAS removal. Other treatment techniques identified to remove PFASs include anion exchange (AIX), and membrane filtration techniques such as reverse osmosis and nanofiltration (NF).12 In contrast to adsorption materials like GAC or AIX, removal efficiencies of membrane processes generally are independent of concentrations of organic matter, salts or co-contaminants as the rejection mechanism of the membrane functions primarily by size-exclusion.14 Membranes can be operated for long periods of time as long as membrane fouling is controlled in the treatment train design.15,16 In contrast to direct application of adsorption materials like GAC and AIX, the application of a nanofiltration treatment step gives greater stability and reliability for the removal of PFASs especially in the case of fluctuating PFAS levels in raw water and given the risk that future PFAS regulatory levels may be lowered. Furthermore, nanofiltration can be used to remove natural organic matter, hardness, pesticides, and microbiological pathogens, thereby incorporating several treatment goals in a single treatment process. In order to make membrane techniques ready for large scale treatment of water sources contaminated with PFASs, suitable management of the membrane reject water, also referred to as concentrate, brine or retentate, is crucial. Reject water is commonly released into nearby watercourses or the ocean, reinjected into deep non-potable aquifers, or sent to wastewater treatment facilities.14 These concentrate management solutions are however hardly feasible for PFAS contaminated concentrates as one should prevent emissions into the environment and wastewater treatment is typically not able to remove PFASs sufficiently.17 Therefore, treatment of the concentrate laden with PFASs and other contaminants remains a challenge and is considered one of the main limitations of membrane filtration.12,18–20
Few studies exist evaluating differences in PFAS adsorption of both GAC and AIX sorbents in a direct comparison. Even fewer studies exist combining full-scale membrane techniques with pilot-scale GAC and AIX filters, respectively, even though this treatment set-up is being discussed as having the potential to be a new standard approach for the removal of PFASs in full-scale drinking water treatment.12,21 In this study, the treatment of PFAS contaminated raw water was investigated using a full-scale nanofiltration membrane pilot plant combined with column adsorption experiments applying a GAC and an AIX material. Objectives were to i) investigate the removal efficiency of PFASs using a nanofiltration membrane and ii) compare the sorbent materials GAC and AIX for the removal of PFAS contaminated raw water as well as membrane reject water and hence examine the effect of PFAS concentration factors on the adsorption performance of the adsorbents.
2 Experimental
2.1 Nanofiltration pilot plant
Nanofiltration membranes used were spiral wound membranes (NF270-400; Dow Filmtech™ Membranes) with a nominal molecular weight cut-off (MWCO) of 270 Da. The treatment train consisted of a 30 μm polypropylene patron filter (GE Infrastructure Water and Process Technology Purtrex filter), a feedwater pump with antiscalant injection and two NF270 membranes in series. A feedwater flow rate of 2.3 m3 h−1 was targeted with 78% permeate recovery. Partial clogging of the prefilter due to manganese particles from the delivery pipeline led to the filter being needed to be replaced on seven occasions which resulted in slightly lower feed pressures and slightly higher concentrations of PFAS in the permeate than observed on average. Raw water was groundwater from Stadsträdgården, Uppsala, Sweden, a groundwater well field contaminated with PFASs (Table 2). Samples of raw water, permeate and reject water were taken once a week for a period of 35 weeks (17 March to 17 December 2015). For the general water quality data, see Table 1. Both raw and reject water were further treated using GAC and AIX adsorption columns (Fig. 1).
Table 1 Average water quality data in raw water, water permeating the NF270 membrane and membrane reject water measured at two different occasions throughout the 35 week membrane experiment
Parameter |
Unit |
Raw water |
Permeate |
Reject water |
Temperature |
°C |
8.5 |
8.5 |
8.5 |
Turbidity |
FNU |
0.12 |
0.13 |
0.14 |
Colour |
mg L−1 Pt |
5.0 |
3.8 |
6.3 |
Conductivity |
mS m−1 (25 °C) |
69 |
54 |
117 |
pH |
— |
7.8 |
7.7 |
7.9 |
Hardness |
°dH |
17.4 |
12.6 |
34.9 |
TOC |
mg L−1 |
2.8 |
<1.0 |
14.4 |
HCO3 |
mg L−1 |
333 |
273 |
556 |
NO32− |
mg L−1 |
4.4 |
4.4 |
3.9 |
F− |
mg L−1 |
1.1 |
0.8 |
2.2 |
Cl− |
mg L−1 |
39 |
39 |
40 |
SO42− |
mg L−1 |
39 |
<3.0 |
180 |
COD-Mn |
mg L−1 |
1.3 |
<1.0 |
6.8 |
Fe2+/3+ |
μg L−1 |
<20 |
<20 |
<20 |
Mn2+ |
μg L−1 |
<5 |
<5 |
5.3 |
Cu2+ |
μg L−1 |
<20 |
<20 |
38 |
Na+ |
mg L−1 |
25.9 |
24.4 |
33.8 |
Ca2+ |
mg L−1 |
98.1 |
74.1 |
186.5 |
Mg2+ |
mg L−1 |
15.6 |
9.3 |
38.1 |
U6+ |
μg L−1 |
31 |
<0.5 |
150 |
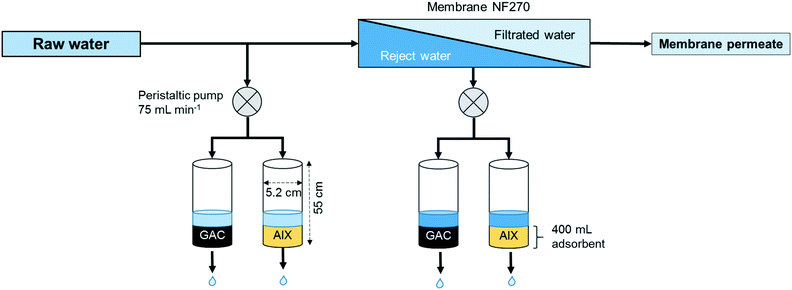 |
| Fig. 1 Schematic of the treatment of PFAS contaminated raw water using two nanofiltration membranes connected in series resulting in filtrated water and membrane reject water. In addition, the raw water and the membrane reject water were treated using granulated activated carbon (GAC) or anion exchange (AIX) adsorption material. | |
2.2 Column experiments
Adsorbents in the column experiments were GAC of the type Filtrasorb® 400 (Calgon Carbon Corporation, Feluy, Belgium) and the AIX resin A600 (Purolite®, Llantrisant, Wales, for specifications, see the ESI†). Column experiments were run at the Bäcklösa municipal drinking water treatment plant (Uppsala, Sweden) according to a procedure described earlier22 (Fig. 1). Briefly, 400 mL adsorbent was filled into a 55 cm high and 5.2 cm wide glass column each. A sintered glass filter was integrated at the bottom of each column to hold the adsorbent in place. Two different water types were run through the columns: untreated raw water and membrane reject water. Flow rates through the columns were kept constant at 70–80 mL min−1 for each column with the help of peristaltic pumps.
2.3 Sampling and analysis
Column experiments were run over a period of 12 weeks and samples were taken every two weeks. This resulted in an experiment runtime of 23
100 bed volumes (BVs, see also eqn (2)) for all GAC (FS400) columns and 18
600 and 21
600 BVs for the AIX (A600) columns treating raw and membrane reject water, respectively. All columns were backwashed every week after each sampling occasion in order to simulate typical full-scale operation. Samples were taken in 250 mL plastic bottles according to the instructions provided by ALS Scandinavia and stored at 4 °C in the dark before being shipped to ALS Scandinavia located in Stockholm, Sweden, for analysis.23 In total, 15 PFASs were analyzed including C4–C12 perfluorocarboxylates (PFCAs; i.e. PFBA, PFPeA, PFHxA, PFHpA, PFOA, PFNA, PFDA, PFUnDA, PFDoDA), C4, C6, C8 and C10 perfluorosulfonates (PFSAs; i.e. PFBS, PFHxS, PFOS, PFDS), the C8 perfluoroalkyl sulfonamide FOSA and the fluorotelomer sulfonate 6
:
2 FTSA. Standard method reporting limits varied between 2 and 25 ng L−1. For the purpose of illustrating the adsorption treatment's removal efficiency R [%] in respective graphs, PFAS concentrations lower than the limit of quantification (LOQ) in outgoing column water were treated as completely removed (R = 100%). For calculating average removal efficiencies for the membrane process, concentrations <LOQ were set to 0.5 LOQ. The performance of the adsorption materials with respect to PFAS removal was compared with that of the column adsorption model proposed by Lin and Huang:24 | 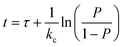 | (1) |
where t describes the elapsed experimental time [h], τ stands for the half-time of saturation [h or BV], kc is the column constant [h−1 or BV−1] and P is the fraction of PFAS breakthrough through the material (C/C0). In order to normalize eqn (1) for the applied flow rate, the time variable in eqn (1) can be expressed as treated BV as expressed by eqn (2): |  | (2) |
where t is the experimental time [h], r describes the flow rate of water through the column [mL h−1] and Vad [mL] is the volume of the adsorbent material. From the linear regression of ln(P/(1 − P)) versus treated BV (eqn (1)) one can thus determine the model parameters τ as the y-intercept and kc from the regressions slope. τ [BV] is a measure for the adsorption capacity of the respective material, as it corresponds to the 50% breakthrough, while the column constant kc [BV−1] describes the mass transfer rate for the column. The mass transfer rate includes not only the effects of the adsorbent but also the column hydraulics such as short circuiting and wall effects. Calculations and graphical visualizations were carried out with the R software and the RStudio interface.25,26
3 Results and discussion
3.1 Removal efficiency of the nanofiltration process
Average concentration factors of reject water vs. feedwater, CREJ/CRAW, reached values of up to 5 and were higher for longer chain PFASs (Tables 2 and S1 in the ESI†). It is difficult to draw conclusions about the removal efficiency of short chain vs. long chain PFASs as feedwater concentrations were higher for longer chain PFASs naturally leading to higher concentration factors. The average removal efficiency of >99% in this study is higher than what was reported for other studies applying NF270 membranes to treat PFAS containing water.15,27,28 Appleman and coauthors27 observed concentrations of up to 40 ng L−1 of PFBS and PFHxS in the permeate of a NF270 membrane treating spiked deionized water (initial concentrations ca. 1000 ng L−1). In their study, other short chain PFASs (i.e. PFBA, PFPeA and PFHxA) also showed lower rejection rates than what was found in the present study. Yu et al.28 observed a rejection rate of PFOS (initial concentration 100 μg L−1) between 94 and 97% for both deionized water and deionized water fortified with a humic acid concentration of 20 mg L−1 with decreasing removal efficiency and with increasing transmembrane pressure on the NF270 membrane. They saw a slight increase in the removal efficiency for the humic acid containing PFOS solution as compared to the pure deionized water solution. The higher removal efficiencies found in the present study compared to their study might have arisen because the feedwater PFAS concentrations were lower or a lower membrane flux was used. Other factors that influence the removal efficiency include constituents present in natural groundwater, possible removal in the prefilter, or the degree of membrane fouling which has shown both positive and negative effects for the rejection of organic micropollutants.15,27,29 Generally, comparisons to other studies should be made with care as many experimental parameters as well as water quality are often substantially different between studies. Other studies have not used natural raw water as was done in this study, which hampers direct comparisons.
Table 2 Average concentrations [ng L−1] of frequently detected PFASs in raw water, membrane permeate and reject water throughout the full 35 week membrane experiment. Molecular weights (MW [g mol−1]) are given for comparison with the molecular weight cut-off of the NF270 membrane (270 Da). CREJ/CRAW describe compound-specific average concentration factors reached in reject water compared to raw water. Values for CREJ/CRAW relate to the average reporting limits (LOQ), where values <LOQ were replaced with 0.5 LOQ
|
PFBA |
PFPeA |
PFHxA |
PFHpA |
PFOA |
PFBS |
PFHxS |
PFOS |
MW [g mol−1] |
213.03 |
263.04 |
313.04 |
363.05 |
413.06 |
299.09 |
399.10 |
499.12 |
Raw water |
<6.0 |
<7.0 |
14 |
<8.0 |
<10 |
11 |
110 |
39 |
Permeate |
<6.0 |
<5.0 |
<5.0 |
<5.0 |
<5.0 |
<5.0 |
<10 |
<7.0 |
Reject water |
12 |
12 |
65 |
15 |
35 |
47 |
470 |
190 |
C
REJ/CRAW |
>2.0 |
>1.7 |
4.6 |
>1.9 |
>3.5 |
4.3 |
4.4 |
5.0 |
Interestingly, PFASs with molecular weights (MWs) lower than the nominal MWCO of 270 Da were found to be concentrated by the membrane process by a factor of approximately two. This is in line with the findings of Appleman et al., who reported that PFBA (MW = 213 g mol−1) was rejected to 93% by filtration through NF270.27 This indicates that other processes than size exclusion were involved for the rejection of PFASs, such as electrostatic repulsion and diffusion into the polymeric phase of the membrane.16,19,30,31 Average PFAS concentrations in the membrane permeate were <LOQ. In individual occasions, due to prefilter clogging, PFHxS and PFOS were detected at concentrations >LOQ in the permeate. The respective removal efficiencies reached by the membrane treatment were, however, >95% in all occasions (Table S1 in the ESI†).
3.2 Removal efficiency of the adsorbents
Longer chain PFASs (i.e. PFHxA, PFOA, PFHxS and PFOS) were removed more efficiently than short chain homologues (i.e. PFBA, PFPeA and PFBS) by both materials (Fig. 2). This was expected, as the adsorption of longer chain PFASs is reportedly promoted by hydrophobic effects for both GAC and AIX.13,32,33 In line with results obtained in earlier research, PFSAs were retained better than PFCAs, which was especially apparent for the AIX resin.11,22 Removal efficiencies of below zero percent were observed for both materials for PFHxA and PFHpA, indicating desorption processes over time. This has been observed in previous studies and can be explained by displacement of the shorter chain PFCAs by DOC, other contaminants and longer chain PFASs.13,22,34,35 Interestingly, desorption was observed for the GAC column treating the less concentrated raw water but not for GAC fed by the membrane reject water. Slopes for change in PFAS removal efficiency for the GAC material over time generally were less steep for the GAC column treating reject water as compared to the GAC column treating raw water. After 23
100 BVs, removal efficiencies for PFHxA were even larger for the GAC column treating reject water (16%) than those for GAC treating raw water (−8%). One possible explanation could be that the larger amount of organic matter in the reject water compared to raw water (14 mg L−1vs. 2.8 mg L−1, see Table 1) created additional sorption sites in the column treating reject water.36,37 In addition, enhanced biofilm growth in the column treating reject water could increase the sorption capacity of GAC for PFASs as observed for other micropollutants.38–40 The AIX material performed better for the treatment of both raw and membrane reject water than GAC in the beginning of the experiment. However, as time continued, the GAC material was observed to consistently remove around 20% of the frequently detected PFCAs (viz. PFHxA, PFHpA and PFOA). In contrast to that the AIX column showed a steeper decrease in the removal efficiency over time, and a lower removal efficiency was observed than that for the GAC column for these compounds towards the end of the experiment.
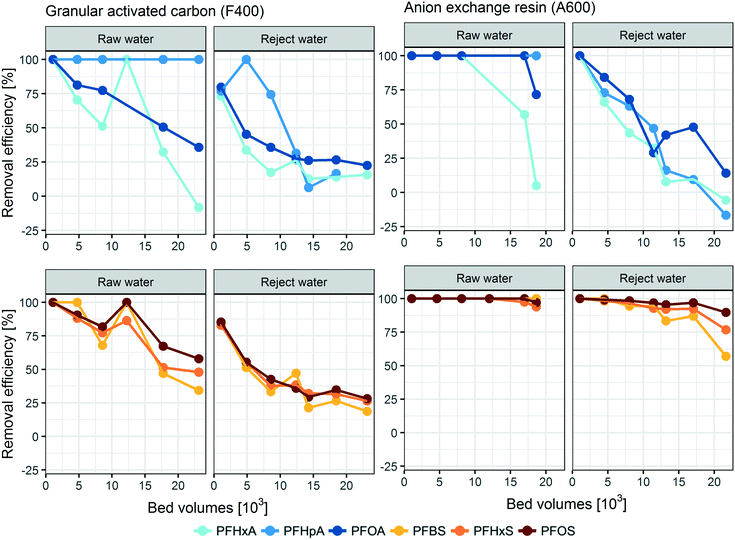 |
| Fig. 2 Removal efficiency [%] of frequently detected PFCAs (top) and PFSAs (bottom) for the evaluated granular activated carbon material (F400, left) and anion exchange resin (A600, right) depending on bed volumes treated. Treatment of both raw water and water rejected by a NF270 membrane was evaluated. Please note that lines are solely added to guide the eye, and do not represent a model. | |
3.3 Column half-time of saturation and total loading
Saturation half-time τ derived with the Lin–Huang model was lower for the GAC material than that for the AIX material, i.e. predicted breakthrough of respective compounds is faster for GAC than for AIX. Furthermore, half-time of saturation derived for both materials generally showed a trend towards longer column life-times for PFASs with greater perfluorocarbon chain length (Fig. S2 in the ESI†), which is a trend previously found for both GAC and AIX materials.22,35 Significant values for a linear regression could be derived for PFOA, PFBS, PFHxS and PFOS for the GAC column treating raw water, PFHxA, PFOA, PFBS, PFHxS and PFOS for the GAC column treating reject water, and PFHxA, PFHpA, PFOA, PFBS, PFHxS, and PFOS for AIX treating reject water (Table S3 in the ESI†), indicating that observed breakthrough curves were complete and can be expected to follow the pseudo steady state squared driving force mass transfer model.24 Average membrane reject water concentrations of PFOA, PFBS, PFHxS and PFOS were by factors of >4, 5, 4, and 5 greater, respectively, than those in raw water during the duration of the column experiments (Table S2 in the ESI†). Interestingly, the half-time of saturation for the GAC column treating raw water was only around a factor of two greater for these compounds than for the GAC column treating reject water (Table S3 in the ESI†). For example, for PFHxS, the most prevalent PFAS in the raw feedwater with 140 ng L−1, the GAC column's half-time of saturation was 76 days while for the reject water column with 590 ng L−1 PFHxS it was 38 days. Similarly for PFOS, the second most prevalent PFAS with 49 ng L−1 in the feedwater, the GAC column half-time of saturation was 95 days while for the reject column with 250 ng L−1 it was 40 days. The initial concentrations therefore have a complex influence on the GAC adsorption performance in this study, and breakthrough curves do not superimpose each other when expressed on a concentration normalized basis in contrast to what was previously found for GAC adsorption of other micropollutants.41,42 This could be explained by the impact of organic matter and biofilms which can enhance the sorption capacity for PFASs in the GAC column treating reject water in comparison to the GAC column treating raw water.
When considering the total loading, the Lin–Huang model predicts that in the present study GAC was more efficient at removing PFASs from membrane concentrate than from raw water. This observation is further highlighted when summarizing total PFAS adsorption for the adsorption materials on a volume of adsorbent basis. Fig. 3 shows the PFAS mass removed per liter adsorption material. Both GAC and AIX were more efficient in removing PFASs from membrane reject water than from raw water. Overall, GAC removed 2.6-fold as much total PFAS mass per material volume from reject water than from raw water (10 vs. 4.1 mg total PFAS per L material), while AIX removed 4.1 times more PFAS mass from reject than from raw water (19 vs. 4.5 mg total PFAS per L material), respectively. The performance differences between the two materials appear more clearly for the columns treating reject water where AIX showed 1.8-fold greater total PFAS removal per liter material than GAC as opposed to merely 1.1-fold greater removal for more dilute raw water (Fig. 3). The increased uptake of total PFASs for the columns treating membrane reject water is in agreement with a previous study on micropollutant removal using several engineered materials.43 Earlier studies have suggested that the presence of organic matter reduces PFAS removal by GAC, indicating that organic matter competes for sorption sites with PFASs and other micropollutants.9,13,27 The performance difference between GAC and AIX could therefore be explained by the lower capacity of AIX resin for organic matter.44 Thus, higher organic matter concentrations in the membrane reject water possibly influenced the overall PFAS removal performance of AIX to a lesser extent than the GAC performance. It was expected that the AIX adsorption capacity would be adversely affected by the greater concentrations of sulphate, bicarbonate, and organic matter in the membrane reject water, however AIX seemed to be influenced by the water quality only to a small extent.
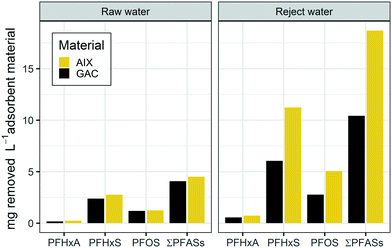 |
| Fig. 3 Total PFAS mass removed per volume adsorbent [mg PFASs removed per L adsorbent material] for the experiment duration of 12 weeks. Total amounts of treated bed volumes of raw water were 23 100 BVs for the granular activated carbon (GAC; Filtrasorb® 400) and 18 600 BVs for the anion exchange (AIX; Purolite® A600) material and for the reject water 23 100 BVs (GAC) and 21 600 BVs (AIX), respectively. | |
3.4 Future perspectives
This study evaluated the use of membrane filtration to improve the removal of PFASs in raw water for drinking water production in comparison to using only GAC or AIX treatment and the use of GAC and AIX to free membrane reject water from PFASs. The nanofiltration process successfully removed PFASs frequently detected from groundwater in the City of Uppsala, Sweden, on average to >99%. Even PFASs with molecular weights smaller than the membrane nominal MWCO of 270 Da were found to be concentrated in the membrane reject water, suggesting other reject mechanisms additional to size-exclusion. In contrast to the removal of PFASs using GAC and AIX, the nanofiltration process was stable and provided the additional benefit of reducing concentrations of organic matter (TOC), hardness, and uranium as shown in the difference between raw water and permeate values in Table 1. The feasibility of applying either GAC or AIX for the treatment of membrane reject water depends on water quality and on target water quality levels for the treated reject water. Generally, AIX showed up to threefold greater half-time of saturation than GAC, whereas the GAC material removed around 20% of the incoming PFAS concentration consistently after treatment of 15
000 BVs. PFAS adsorption onto AIX appears to be more dependent on the PFAS concentrations in the water to be treated and less affected by the water quality, while for GAC organic matter may be a primary factor for lower overall PFAS adsorption.
Ultimately, this study showed that stable PFAS removal from drinking water can be achieved by NF filtration and that PFAS adsorption using AIX and GAC can be up to four-fold more efficient for a concentrated waste stream than for more dilute raw feedwater. The combination of NF with AIX or GAC adsorbents offers drinking water providers reliable PFAS removal and the benefit of effective NF waste stream treatment using regenerable AIX and GAC materials. Future work should verify the greater adsorption capacity offered by both AIX and GAC when treating NF concentrate as opposed to more dilute feedwater and address cost–benefit calculations in order to provide a cost competitive solution both for PFAS removal from drinking water and for prevention of PFAS release into the environment. Future work should also examine the effect of co-removal of organic matter on PFAS removal for both AIX and GAC. Greater organic matter concentrations are often associated with a negative influence on PFAS removal for both AIX and GAC in particular.
Generally, future applications need to be able to remove PFASs from membrane concentrate for proper disposal. Results presented in this study indicate that adsorption onto either GAC or AIX with subsequent incineration of the materials can be considered a realistic solution, ready for current full scale application. Other possibilities include membrane arrays which could increase recovery up to 90–95% and thus increase the PFAS concentrations in the membrane reject water by a factor of >10, which may make GAC or AIX even more efficient. The presented treatment combination could further be compared to treating a more concentrated waste stream with destructive processes, such as coagulation/filtration combined with incineration of the filter residuals or novel techniques like electrochemical or sonochemical treatment processes.
Conflicts of interest
There are no conflicts to declare.
Acknowledgements
This work was partly funded by the Swedish Research Council Formas (PFAS-FREE, contract nr. 942-2015-1554). The authors would also like to thank Uppsala Water and Waste AB for the provided support.
References
-
E. Kissa, Fluorinated Surfactants and Repellents, Taylor & Francis Inc, 2001 Search PubMed.
- K. Prevedouros, I. T. Cousins, R. C. Buck and S. H. Korzeniowski, Sources, Fate and Transport of Perfluorocarboxylates, Environ. Sci. Technol., 2006, 40, 32–44 CrossRef CAS PubMed.
- M. Haukås, U. Berger, H. Hop, B. Gulliksen and G. W. Gabrielsen, Bioaccumulation of per- and polyfluorinated alkyl substances (PFAS) in selected species from the Barents Sea food web, Environ. Pollut., 2007, 148, 360–371 CrossRef PubMed.
- Z. Zhao, Z. Xie, A. Möller, R. Sturm, J. Tang, G. Zhang and R. Ebinghaus, Distribution and long-range transport of polyfluoroalkyl substances in the Arctic, Atlantic Ocean and Antarctic coast, Environ. Pollut., 2012, 170, 71–77 CrossRef CAS PubMed.
-
ECHA, European Chemical Agency, Candidate list of substances of very high concern for authorisation, 2017, Accessed 29 October 2018.
-
C8 Science Panel, The Science Panel Website, 2017, Accessed 29 October 2018.
- I. Gyllenhammar, U. Berger, M. Sundström, P. McCleaf, K. Eurén, S. Eriksson, S. Ahlgren, S. Lignell, M. Aune, N. Kotova and A. Glynn, Influence of contaminated drinking water on perfluoroalkyl acid levels in human serum – A case study from Uppsala, Sweden, Environ. Res., 2015, 140, 673–683 CrossRef CAS PubMed.
-
Toxicological Effects of Perfluoroalkyl and Polyfluoroalkyl Substances, ed. J. C. DeWitt, Springer International Publishing, Switzerland, 2015 Search PubMed.
- M. F. Rahman, S. Peldszus and W. B. Anderson, Behaviour and fate of perfluoroalkyl and polyfluoroalkyl substances (PFASs) in drinking water treatment: A review, Water Res., 2014, 50, 318–340 CrossRef CAS PubMed.
- B. R. Shivakoti, S. Fujii, M. Nozoe, S. Tanaka and C. Kunacheva, Perfluorinated chemicals (PFCs) in water purification plants (WPPs) with advanced treatment processes, Water Sci. Technol.: Water Supply, 2010, 10, 87–95 CAS.
- T. D. Appleman, C. P. Higgins, O. Quiñones, B. J. Vanderford, C. Kolstad, J. C. Zeigler-Holady and E. R. Dickenson, Treatment of poly- and perfluoroalkyl substances in U.S. full-scale water treatment systems, Water Res., 2014, 51, 246–255 CrossRef CAS PubMed.
- N. Merino, Y. Qu, R. A. Deeb, E. L. Hawley, M. R. Hoffmann and S. Mahendra, Degradation and Removal Methods for Perfluoroalkyl and Polyfluoroalkyl Substances in Water, Environ. Eng. Sci., 2016, 33, 615–649 CrossRef CAS.
- C. Eschauzier, E. Beerendonk, P. Scholte-Veenendaal and P. D. Voogt, Impact of Treatment Processes on the Removal of Perfluoroalkyl Acids from the Drinking Water Production Chain, Environ. Sci. Technol., 2012, 46, 1708–1715 CrossRef CAS PubMed.
- B. van der Bruggen, L. Lejon and C. Vandecasteele, Reuse, Treatment, and Discharge of the Concentrate of Pressure-Driven Membrane Processes, Environ. Sci. Technol., 2003, 37, 3733–3738 CrossRef CAS PubMed.
- C. Y. Tang, Q. S. Fu, C. S. Criddle and J. O. Leckie, Effect of Flux (Transmembrane Pressure) and Membrane Properties on Fouling and Rejection of Reverse Osmosis and Nanofiltration Membranes Treating Perfluorooctane Sulfonate Containing Wastewater, Environ. Sci. Technol., 2007, 41, 2008–2014 CrossRef CAS PubMed.
- E. Steinle-Darling and M. Reinhard, Nanofiltration for Trace Organic Contaminant Removal: Structure, Solution, and Membrane Fouling Effects on the Rejection of Perfluorochemicals, Environ. Sci. Technol., 2008, 42, 5292–5297 CrossRef CAS PubMed.
- M. M. Schultz, C. Higgins, C. A. Huset, R. Luthy, D. F. Barofsky and J. A. Field, Fluorochemical Mass Flows in a Municipal Wastewater Treatment Facility, Environ. Sci. Technol., 2006, 40, 7350–7357 CrossRef CAS PubMed.
- J. Thompson, G. Eaglesham, J. Reungoat, Y. Poussade, M. Bartkow, M. Lawrence and J. F. Mueller, Removal of PFOS, PFOA and other perfluoroalkyl acids at water reclamation plants in South East Queensland, Australia, Chemosphere, 2011, 82, 9–17 CrossRef CAS PubMed.
- Á. Soriano, D. Gorri and A. Urtiaga, Efficient treatment of perfluorohexanoic acid by nanofiltration followed by electrochemical degradation of the NF concentrate, Water Res., 2017, 112, 147–156 CrossRef PubMed.
- C. Boo, Y. Wang, I. Zucker, Y. Choo, C. O. Osuji and M. Elimelech, High Performance Nanofiltration Membrane for Effective Removal of Perfluoroalkyl Substances at High Water Recovery, Environ. Sci. Technol., 2018, 52, 7279–7288 CrossRef CAS PubMed.
- I. Ross, J. McDonough, J. Miles, P. Storch, P. Thelakkat Kochunarayanan, E. Kalve, J. Hurst, S. S. Dasgupta and J. Burdick, A review of emerging technologies for remediation of PFASs, Remediation, 2018, 28, 101–126 CrossRef.
- P. McCleaf, S. Englund, A. Östlund, K. Lindegrena, K. Wiberg and L. Ahrens, Removal efficiency of multiple poly- and perfluoroalkyl substances (PFASs) in drinking water using granular activated carbon (GAC) and anion exchange (AE) column tests, Water Res., 2017, 77–87 CrossRef CAS PubMed.
-
ALS Scandinavia, OV-34a Perfluorinated compounds in water.
- S. H. Lin and C. Y. Huang, Adsorption of BTEX from aqueous solution by macroreticular resins, J. Hazard. Mater., 1999, 70, 21–37 CrossRef CAS PubMed.
-
RDevelopmentCoreTeam, R: A language and environment for statistical computing, R Foundation for Statistical Computing, Vienna, Austria, 2016 Search PubMed.
-
RStudioTeam, RStudio: Integrated Development for R. RStudio Inc., Boston, MA, USA, 2016.
- T. D. Appleman, E. R. Dickenson, C. Bellona and C. P. Higgins, Nanofiltration and granular activated carbon treatment of perfluoroalkyl acids, J. Hazard. Mater., 2013, 260, 740–746 CrossRef CAS PubMed.
- Y. Yu, C. Zhao, L. Yu, P. Li, T. Wang and Y. Xu, Removal of perfluorooctane sulfonates from water by a hybrid coagulation–nanofiltration process, Chem. Eng. J., 2016, 289, 7–16 CrossRef CAS.
- H. Y. Ng and M. Elimelech, Influence of colloidal fouling on rejection of trace organic contaminants by reverse osmosis, J. Membr. Sci., 2004, 244, 215–226 CrossRef CAS.
- C. Bellona and J. E. Drewes, Viability of a low-pressure nanofilter in treating recycled water for water reuse applications: A pilot-scale study, Water Res., 2007, 3948–3958 CrossRef CAS PubMed.
- L. D. Nghiem, A. I. Schäfer and M. Elimelech, Removal of Natural Hormones by Nanofiltration Membranes: Measurement, Modeling, and Mechanisms, Environ. Sci. Technol., 2004, 38, 1888–1896 CrossRef CAS PubMed.
- C. P. Higgins and R. G. Luthy, Sorption of Perfluorinated Surfactants on Sediments, Environ. Sci. Technol., 2006, 40, 7251–7256 CrossRef CAS PubMed.
- K. E. Carter and J. Farrell, Removal of Perfluorooctane and Perfluorobutane Sulfonate from Water via Carbon Adsorption and Ion Exchange, Sep. Sci. Technol., 2010, 45, 762–767 CrossRef CAS.
-
V. Gellrich and V. P. Knepper, The Handbook of Environmental Chemistry: Fluorinated Surfactants and Transformation Products, Springer-Verlag, 2011, pp. 63–72 Search PubMed.
- A. Zaggia, L. Conte, L. Falletti, M. Fant and A. Chiorboli, Use of strong anion exchange resins for the removal of perfluoroalkylated substances from contaminated drinking water in batch and continuous pilot plants, Water Res., 2016, 91, 137–146 CrossRef CAS PubMed.
- J. Gustavsson, L. Ahrens, M. A. Nguyen, S. Josefsson, D. B. Kleja and K. Wiberg, Influence of natural organic matter on the extraction efficiency of flame retardants from surface waters, J. Chromatogr. A, 2017, 1524, 74–86 CrossRef CAS PubMed.
- D. N. Kothawala, S. J. Köhler, A. Östlund, K. Wiberg and L. Ahrens, Influence of dissolved organic matter concentration and composition on the removal efficiency of perfluoroalkyl substances (PFASs) during drinking water treatment, Water Res., 2017, 121, 320–328 CrossRef CAS PubMed.
- J. Scott, A. Karanjkar and D. Rowe, Biofilm covered granular activated carbon for decontamination of streams containing heavy metals and organic chemicals, Miner. Eng., 1995, 8, 221–230 CrossRef CAS.
- C. Quintelas, E. Sousa, F. Silva, S. Neto and T. Tavares, Competitive biosorption of ortho-cresol, phenol, chlorophenol and chromium (VI) from aqueous solution by a bacterial biofilm supported on granular activated carbon, Process Biochem., 2006, 41, 2087–2091 CrossRef CAS.
- M. L. Frankel, T. I. Bhuiyan, A. Veksha, M. A. Demeter, D. B. Layzell, R. J. Helleur, J. M. Hill and R. J. Turner, Removal and biodegradation of naphthenic acids by biochar and attached environmental biofilms in the presence of co-contaminating metals, Bioresour. Technol., 2016, 216, 352–361 CrossRef CAS PubMed.
- C. J. Corwin and R. S. Summers, Controlling trace organic contaminants with GAC adsorption, J. - Am. Water Works Assoc., 2012, E36–E47 CrossRef.
- R. S. Summers, S. M. Kim, K. Shimabuku, S.-H. Chae and C. J. Corwin, Granular activated carbon adsorption of MIB in the presence of dissolved organic matter, Water Res., 2013, 47, 3507–3513 CrossRef CAS PubMed.
- M. Jiang, W. Yang, Z. Zhang, Z. Yang and Y. Wang, Adsorption of three pharmaceuticals on two magnetic ion-exchange resins, J. Environ. Sci., 2015, 31, 226–234 CrossRef CAS PubMed.
- J. Hu, A. Martin, R. Shang, W. Siegers, E. Cornelissen, B. Heijman and L. Rietveld, Anionic exchange for NOM removal and the effects on micropollutant adsorption competition on activated carbon, Sep. Purif. Technol., 2014, 129, 25–31 CrossRef CAS.
Footnotes |
† Electronic supplementary information (ESI) available. See DOI: 10.1039/c9ew00286c |
‡ Now at: Gislaved Government Administration, Gislaved, Sweden. |
|
This journal is © The Royal Society of Chemistry 2019 |