Silver nanoparticle toxicity and association with the alga Euglena gracilis†
Received
30th April 2015
, Accepted 21st September 2015
First published on 21st September 2015
Abstract
The impact of silver nanoparticles (AgNPs) on aquatic algae has largely been studied with model species that possess a rigid cell wall. Here, we explored the interactions of AgNPs with Euglena gracilis, a green alga having no cell wall but a pellicle. The toxicity and silver uptake upon 1–2 h of exposure to various concentrations of AgNO3 and AgNPs, having a mean size of 47 nm measured in the exposure medium, were examined. The photosynthetic yield decreased in a concentration-dependent manner and AgNPs were less toxic than AgNO3 based on the total silver added. The cell morphology was significantly altered by AgNPs and AgNO3. The damaging effects of AgNPs on the photosynthesis and morphology were completely prevented by cysteine, suggesting that the toxicity of AgNPs was mediated by dissolved Ag. Indeed, the maximal quantity of cell-associated silver was higher upon exposure to AgNPs compared to that upon AgNO3 exposure, amounting to 5.1 × 10−4 mol Lcell−1 and 1.4 × 10−4 mol Lcell−1 for AgNPs and AgNO3, respectively. However, the difference was not caused by the cellular uptake of AgNPs, but by the strong sorption of AgNPs onto the pellicle.
Nano impact
One of the most controversial issues in algal nanotoxicology is whether nanoparticles can be internalized and if internalization is a prerequisite for toxicity. Here, we examined the interactions of AgNPs with the alga Euglena gracilis, possessing a proteinaceous pellicle instead of a typical cell wall, which we considered as a barrier against nanoparticle uptake. This study provides evidence that AgNPs were not internalized but strongly adsorbed on the pellicle. The toxicity of AgNPs to the alga merely resulted from the dissolved silver released from the nanoparticles.
|
Introduction
The growing production, use and disposal of silver nanoparticles (AgNPs) will eventually lead to their presence in aquatic environments,1,2 with ecological consequences that are difficult to predict.3–6 In the studies of AgNPs and algae, the inhibition of algal photosynthesis and growth has been reported.7–11 While it is accepted that the dissolved Ag released from AgNPs is highly toxic and contributes to the observed AgNP effects, the extent of the contribution of AgNPs to the overall toxicity remains unclear. For instance, AgNPs were shown to inhibit photosynthesis in the freshwater alga Chlamydomonas reinhardtii,7 but their toxicity was completely prevented in the presence of cysteine, a strong silver ligand, thereby indicating that the inhibitory effects of AgNPs were caused solely by the dissolved Ag. Likewise, the toxic effects of AgNPs on the growth, photosynthesis, and chlorophyll production in the marine diatom Thalassiosira weissflogii were prevented following the addition of the thiols glutathione and cysteine to the exposure medium.8 In another study, the inhibitory effects of AgNPs on the growth of the freshwater alga Ochromonas danica were reduced but not fully prevented in the presence of high concentrations of glutathione, suggesting that, besides the dissolved Ag, AgNPs also directly contributed to toxicity.10
Whether nanoparticle internalization in algal cells is a prerequisite for toxicity is not yet understood.6 The algal cell is typically surrounded by a cell wall that could represent a barrier against nanoparticle uptake. The algal cell wall is composed of multiple layers that are chemically heterogeneous among different algal species. The major cell wall components of algae include cellulose, glycoproteins and polysaccharides, which are linked to other structural or functional components to form a rigid complex network.12–14 Diatom algae possess a special type of cell wall which is composed of hydrated silicon dioxide. In general, the algal cell wall is semi-permeable, and the pore size has been estimated to be a few nanometers (5–20 nm).15 Comparing the size of nanoparticles to the pore size of the algal cell wall, it has been hypothesized that only nanoparticles with sizes that are smaller than the size of the pores may cross the cell wall and be internalized in cells via endocytosis.3,16 Internalization of AgNPs was reported in the alga O. danica.10 This algal species lacks a cell wall, and has been previously proven to be capable of undergoing endocytosis.17 On the other hand, a systematic study of the alga C. reinhardtii did not evidence particle internalization either in the wild-type or in the cell wall-free mutant, suggesting that both the cell wall and the cell membrane constitute a barrier for particle internalization.18
In order to address the questions (1) does nanoparticle uptake occur in algae and (2) to what extent do the nanoparticles contribute to toxicity, we examine here the interactions of citrate-coated AgNPs with the freshwater alga Euglena gracilis. This algae species was selected because it does not possess a cell wall but a glycoprotein-containing pellicle, with longitudinal articulated stripes aligned on the surface.19,20 Together with the assessment of toxicity upon short-term exposure, silver uptake and accumulation in E. gracilis was studied.
Materials and methods
Materials
Citrate-coated AgNPs were provided as an aqueous solution with a concentration of 1 g L−1 (9.27 mM, pH 6.5) by NanoSys (Wolfhalden, Switzerland). The AgNP stock solution was kept in the dark to prevent redox reactions, and experimental solutions were freshly prepared in the exposure medium. A stock solution of 50 mM AgNO3 (Sigma) was prepared in deionized water (Barnstead Nanopure, Switzerland) and stored in the dark.
All materials for algal growth were autoclaved to prevent biological contamination. To avoid metal contamination in the silver uptake experiments, polycarbonate and Teflon materials were washed in acid (0.03 M HNO3), and cellulose filters (0.45 μm, Sartorius) were boiled in acid for 1 h and then dried.
To avoid silver complexation, 10 mM 3-morpholinopropanesulfonic acid (MOPS) at pH 7.5 was selected as the exposure medium. The ionic strength of this medium was calculated to be 3 mM using the software Visual MINTEQ v3.0 Beta (http://vminteq.lwr.kth.se/visual-minteq-ver-3-0).
Nanoparticle characterization
The diameter and ζ-potential of 10–100 μM AgNPs in MOPS (10 mM, pH 7.5) were measured between 15 min to 4 h by dynamic light scattering (DLS) using a Zetasizer (Nano ZS, Malvern Instruments). The UV-vis absorbance of AgNPs was recorded after 1 h of exposure to MOPS using a spectrophotometer (UVIKON 930). The dissolution of AgNPs was determined after 2 h of exposure to MOPS. The fraction of dissolved Ag was separated from the nanoparticles via ultrafiltration through a 3 kDa filter unit (Millipore centrifugal concentrator) and ultracentrifugation (145
000 × g, 3 h, CENTRIKON T-2000). The filtrate obtained from ultrafiltration and the supernatant (0.5 mL of aliquot from the upper volume) obtained from ultracentrifugation were acidified for analysis of Ag.
Algal culture and exposure
The alga E. gracilis strain Z (Culture Collection of Algae, Göttingen, Germany) was cultured in the synthetic medium Talaquil (pH 7.5),21 supplemented with vitamins B1 and B12.22,23 The algae were maintained at 20 °C on a shaker (90 rpm, Infors, Switzerland) under light–dark cycles of 12 h each. The cell number and volume were measured using an electronic particle counter (Beckman Coulter Z2, USA).
All exposures were performed within 2 h in MOPS (10 mM, pH 7.5). In this medium, the algae maintained their maximal photosynthetic activity up to 4 h (ESI† Fig. S2). Before exposure to AgNO3 and AgNPs, exponentially grown algae were first centrifuged (2000 × g, 10 min) and then resuspended in MOPS. For the toxicity experiment, the final cell density was 1.5 × 104 cell mL−1, while a higher cell density of 1 × 105 cell mL−1 was used in the uptake experiments to ensure sufficient amounts of silver for quantification. Additionally, the effects of AgNO3 and AgNPs on photosynthesis were also measured at a cell density of 1 × 105 cell mL−1.
Photosynthesis
The toxic effects on photosynthesis were assessed under increasing concentrations of AgNO3 (0–400 nM) and AgNPs (0–40 μM). After 1 and 2 h, the photosynthetic yield was measured by fluorometry using a PHYTO-PAM (Heinz Walz GmbH, Germany). The maximum fluorescence (Fm′) was measured under a short saturating pulse of light, and compared to the fluorescence in the steady state (F). The photosynthetic yield was determined according to the equation: photosynthetic yield = (Fm′ − F)/Fm′. The values were presented as percentage of controls, and were plotted as a function of measured total Ag, dissolved Ag, and also cell-associated Ag.
To determine the contribution of dissolved Ag to AgNP toxicity, cysteine was used as the silver ligand. AgNPs (5 μM) and AgNO3 (100 nM) were first pre-equilibrated with cysteine (1 μM) for 15 min. Then, the algae were exposed to the AgNP–cysteine or AgNO3–cysteine mixture for 1 h and the photosynthetic yield was measured.
Cell morphology
The morphology of the algal cells after 1 h of exposure to AgNO3 (100 nM) and AgNPs (5 μM) in the presence and absence of cysteine (1 μM) was examined after fixation in 4% paraformaldehyde (10 min) using a confocal laser scanning microscope (CLSM, Leica SP5 DMI 6000). Both the chlorophyll fluorescence and the transmitted light image were obtained at different depths along the z-axis. The output image was generated by average intensity projection of the z-axis image stack (ImageJ, version 1.44).
Uptake experiments
The algae were exposed to 0–10 μM AgNPs and 0–500 nM AgNO3 at a cell density of 1 × 105 cell mL−1. After 1 h of exposure, the algae were washed to remove loosely bound AgNPs or adsorbed silver ions following a protocol established in the preliminary experiments (ESI† Fig. S3). The algae exposed to AgNPs were first centrifuged (2000 × g, 10 min) and resuspended in MOPS. After 2 wash cycles, the algae were resuspended in cysteine–MOPS and gently stirred for 5 min. The algae exposed to AgNO3 were centrifuged and resuspended in cysteine–MOPS, followed by stirring for 5 min. After washing, the algae were filtered (SM 16510, Sartorius) and digested for metal analysis. The silver which was measured after the wash steps was operationally defined as the cell-associated silver ({Ag}cell). The measured {Ag}cell was either related to the cell number and expressed as mol per cell, or related to the measured cell volume and expressed as mol Lcell−1. The experiments were performed in technical triplicate and repeated at least twice.
Metal analysis
For metal determination, filters with algae were transferred into Teflon flasks and digested in 3 mL of 65% HNO3 and 0.5 mL of 30% H2O2 in a microwave oven (195 °C, MLS 1200 Mega, Microwave Laboratory System, Switzerland). Each sample was then filled to 25 mL with deionized water in a volumetric flask. The total silver mass (1
:
10 dilution) was measured by inductively coupled plasma mass spectrometry (ICP-MS, Thermo Finnigan, Germany) using the isotope 109Ag. To control the reliability of quantification, water references (M105A, IFA-Tulln, Austria) with a known silver content were measured.
ToF-SIMS analysis
E. gracilis cells were exposed to 250 nM AgNO3 as well as 1 and 5 μM AgNPs at a cell density of 1 × 105 cell mL−1. After 1 h, the algae were washed as described above. Then, the algae were fixed in 2.5% glutaraldehyde on ice for 10 min, and washed twice with deionized water. After centrifugation (2000 × g, 10 min), the algae pellet was soaked in 0.6% ammonium acetate for 10 s, deposited on a silicon substrate and dried using nitrogen gas flow.
ToF-SIMS analysis (ToF.SIMS 5 instrument, ION-TOF GmbH) was performed in both spectral and imaging modes. Using the spectral mode, 107Ag+ and 109Ag+ were detected with high mass resolution at masses of 106.91 and 108.90, respectively. 25 keV Bi+ primary ions were used to ensure high sensitivity to silver, together with electron flooding to compensate for charge accumulation at the sample surface. Based on in-depth sputtering with 2 keV O2− over 23.4 s (20 scans), 5.2 s of sputtering was selected as the optimal sputtering time for silver detection (ESI† Fig. S6). Secondary ions of positive polarity were analysed from surface areas of 150 × 150 μm2 before and after sputtering for 5.2 s (4 scans). To ensure a reasonable signal over noise ratio, each measurement accounted for a total of 200 scans, which represents a surface ion dose of 5.5 × 1013 ions per cm2. Additionally, each analysed cell was characterized in imaging mode with a high lateral resolution (~200 nm) to gain insights into its spatial conformation. To ensure the reliability and reproducibility of the measurements, a minimum of five cells for each sample were randomly selected and analysed.
Data analysis
All data analyses were performed in the software GraphPad Prism version 4.0 (USA). Concentrations leading to 50% inhibition (EC50s) of the photosynthetic yield were determined by nonlinear regression sigmoidal dose–response curve fitting using the Hill slope equation, and were presented as the mean of three independent experiments, with a 95% confidence interval. Differences in the concentration–response curves were compared based on the Hill slope and the EC50 values using an F-test. The photosynthetic values and cell volume in the cysteine experiment were analysed by ANOVA followed by Dunnett's post-test. The uptake data were fitted by either a linear regression or a non-linear regression model using a sigmoidal dose–response equation (ESI† Fig. S4).
Results
Nanoparticle characterization
The AgNPs in the original stock solution (9.27 mM) displayed an average size of 20 ± 0.2 nm and an average ζ-potential of −34 ± 2 mV. Upon dilution in 10 mM MOPS at pH 7.5, the AgNPs remained stable up to 4 h with an average size between 38 and 73 nm (Fig. 1A), and a ζ-potential between −23 and −28 mV for AgNP concentrations between 10 and 100 μM (Fig. 1B). The AgNP suspension displayed a maximal UV-vis absorbance at 410 nm (Fig. S1†). The amount of dissolved silver in AgNP suspensions (10–100 μM) was 0.5–3.5% as determined by ultracentrifugation and 1.3–2.6% by ultrafiltration, with a combined mean value of 1.7% (Table 1).
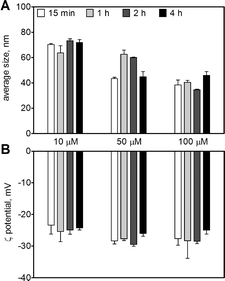 |
| Fig. 1
ζ-average size (A) and ζ-potential (B) of 10, 50, 100 μM AgNPs in 10 mM MOPS, pH 7.5. | |
Table 1 Percentage of dissolved silver in AgNP suspensions
AgNP, μM |
Ultracentrifugation |
Ultrafiltration |
Mean |
SD |
Mean |
SD |
10 |
3.5% |
0.7% |
1.3% |
0.7% |
25 |
0.8% |
0.2% |
2.6% |
0.1% |
100 |
0.5% |
0.1% |
1.6% |
0.1% |
Combined mean 1.7% |
Effects on algal photosynthesis
Inhibition of the photosynthetic yield increased with increasing concentration of AgNO3 and AgNPs. After 1 h of exposure, AgNO3 displayed higher toxicity than AgNPs based on the total silver added (Fig. 2A). At the highest applied concentration of AgNO3 (400 nM) and AgNPs (40 μM), the photosynthetic yield decreased to 6% and 18%, respectively, compared to that of the control cells. The EC50 values were 85 nM for AgNO3 and 1858 nM for AgNPs (Table 2). Extending the exposure time to 2 h yielded similar concentration–response curves compared to 1 h for both AgNO3 and AgNPs, with EC50 values of 89 nM and 1487 nM, respectively.
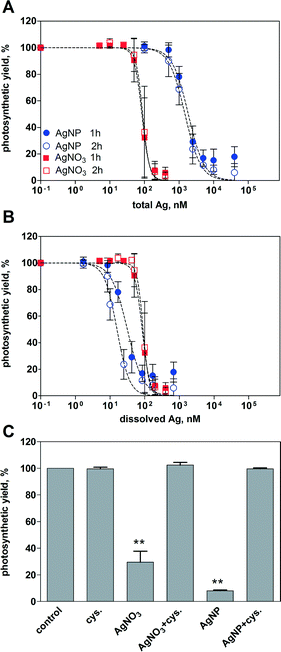 |
| Fig. 2 Inhibition of photosynthesis upon 1 and 2 h of exposure to AgNO3 and AgNPs. Concentration–response curves were plotted as a function of total Ag (A) and dissolved Ag measured in the exposure medium (B). Data were analysed using F-test. Based on the total Ag, AgNO3 was found to be more toxic than AgNPs (p < 0.0001). There was no significant difference between 1 and 2 h of exposure for both AgNO3 (p = 0.8331) and AgNPs (p = 0.1947). Based on the dissolved Ag, AgNP toxicity was significantly higher than that of AgNO3 (p < 0.0001). The photosynthetic yield was measured upon 1 h of exposure to 100 nM AgNO3 and 5 μM AgNPs in the presence of cysteine (C). ** = significantly different from control, p < 0.01, ANOVA, Dunnett's multiple comparison test. | |
Table 2 AgNO3 and AgNP EC50 values for the photosynthetic yield in E. gracilis, expressed on the basis of measured total and dissolved Ag concentrations
Treatment |
Time |
EC50, nM |
95% CI, nM |
As a function of total Ag |
AgNO3 |
1 h |
85 |
74–97 |
AgNPs |
1 h |
1858 |
1511–2284 |
AgNO3 |
2 h |
89 |
78–102 |
AgNPs |
2 h |
1487 |
1286–1719 |
As a function of dissolved Ag (assuming 1.7% dissolution) |
AgNPs |
1 h |
32 |
26–39 |
AgNPs |
2 h |
16 |
14–19 |
By plotting the photosynthetic yield as a function of the mean value of dissolved silver (1.7% of total Ag), AgNPs appeared to be more toxic than AgNO3 (Fig. 2B). The calculated EC50 values were 32 nM after 1 h, and 16 nM after 2 h (Table 2). Based on the whole range of measured dissolved silver (0.5–3.5%), the resulting EC50 values of AgNPs were all significantly lower compared to those of AgNO3 (ESI† Table S1).
The role of dissolved Ag in AgNP toxicity was examined using the silver ligand cysteine. The photosynthetic yield of the control cells was reduced to 30% after 1 h of exposure to 100 nM AgNO3, and 8% after exposure to 5 μM AgNPs (Fig. 2C). In the presence of 5 μM cysteine, no decrease of photosynthetic yield was detectable, suggesting that the AgNP toxicity was mediated by dissolved Ag.
Effects on cell morphology
Microscopic examination of algae exposed to 100 nM AgNO3 and 5 μM AgNPs revealed morphological changes. The control cells displayed a spindle-like morphology with an average cell volume of 1532 ± 81 fL (Fig. 3A). The cells exposed to AgNO3 were less elongated compared to the control cells and the cell volume increased up to 2654 ± 132 fL (Fig. 3B). In the case of AgNPs, the cells were completely round, and the cell volume increased to 2774 ± 172 fL (Fig. 3C). In the presence of cysteine, the cell morphology and cell volume of the algae exposed to AgNO3 and AgNPs were similar to those of the control cells (Fig. 3E and F), while cysteine had no effect on the morphology or cell volume (Fig. 3D).
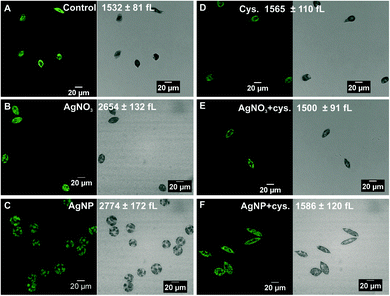 |
| Fig. 3 CLSM images and measured cell volume of the algae exposed to AgNO3 and AgNPs in the absence and presence of cysteine. Control cells (A), and cells after 1 h of exposure to 100 nM AgNO3 (B), 5 μM AgNPs (C), cysteine (D), 100 nM AgNO3 + cysteine (E), and 5 μM AgNPs + cysteine (F). | |
Uptake experiments
Cell-associated silver ({Ag}cell) in E. gracilis after 1 h of exposure increased with increasing concentration of AgNO3. The increase in {Ag}cell per algal cell was linear (R2 = 0.99) over the AgNO3 concentration range between 25 and 500 nM (Fig. 4A). Detailed values of {Ag}cell are listed in Table S2 in the ESI.† At the highest AgNO3 concentration, the {Ag}cell was 6.2 × 10−16 mol per cell. When {Ag}cell was related to the measured cell volume, ranging from 1317 to 4260 fL in the case of AgNO3 and from 1797 to 3262 fL in the case of AgNPs, the silver uptake was non-linear. A maximal {Ag}cell value of 1.4 × 10−4 mol Lcell−1 was measured at 500 nM AgNO3. In the case of AgNPs, {Ag}cell increased with increasing AgNP concentration up to 2.5 μM AgNPs and remained constant upon further increase of the AgNP concentration up to 10 μM (Fig. 4B). Above 2.5 μM AgNPs, maximal values of 1.5 × 10−15 mol per cell and 5.1 × 10−4 mol Lcell−1 were measured. A comparison of {Ag}cell in the AgNO3 and AgNP treatments was carried out as a function of dissolved silver (Fig. 4C and S4). In the concentration range of 25–200 nM dissolved Ag, {Ag}cell was 5–15 times higher in the AgNP-exposed cells.
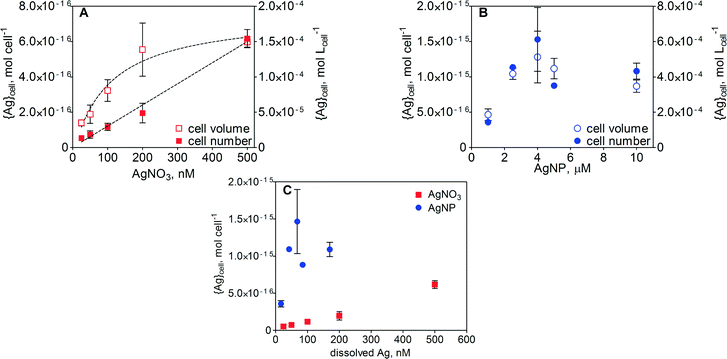 |
| Fig. 4 Cell-associated silver ({Ag}cell) measured in E. gracilis after 1 h of exposure to various concentrations of AgNO3 (A) and AgNPs (B) expressed as mol Lcell−1 (left y-axis) and mol per cell (right y-axis), and comparison of {Ag}cell between AgNO3 and AgNP exposure based on dissolved silver (C). | |
ToF-SIMS analysis
After 1 h of exposure to AgNO3 and AgNPs, the surface of E. gracilis was analyzed using ToF-SIMS. The chemical map of three positive ions, Si+, CxHy+ and Ag+, was resolved in the spectral mode (Fig. 5). The signal of the Si+ ion refers to the substrate where the algae were deposited. The fragment ion type CxHy+ is typically representative of organic matter, and was therefore used to locate the algal cells. The Ag+ ions in the chemical map were resolved from both 107 and 109 mass types to enhance the overall signals. The silver spectrum of mass 107, being the most representative silver mass detected, is shown in Fig. 5 (right column). Silver mass 109 behaved similarly yet with lower intensity (data not shown). The overall algae structure was additionally characterized in the imaging mode with the sum of the total ion counts (Fig. 5, left column), showing that the cells remained intact after sample preparation.
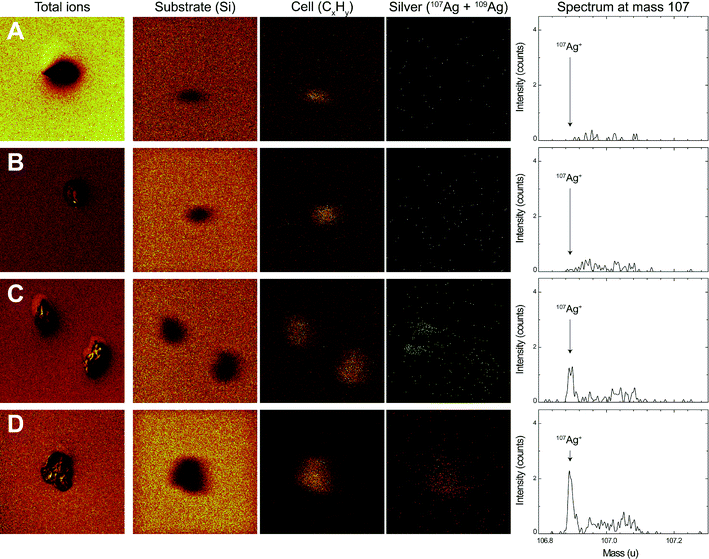 |
| Fig. 5 ToF-SIMS chemical analysis after sputtering of the control cells (A), cells exposed to 250 nM AgNO3 (B), 1 μM AgNPs (C) and 5 μM AgNPs (D). From left to right: total counts (imaging mode), chemical map of the substrate (Si+), cell (CxHy+) and silver (107Ag+ + 109Ag+), and mass 107 spectrum of silver from the cell area. Surface area: 150 × 150 μm2. | |
The ToF-SIMS analysis revealed the presence and the distribution of silver at the surface of E. gracilis cells. A comparison of chemical analyses before and after O2 sputtering is shown in Fig. S7 in the ESI.† Before sputtering, silver was detected over the whole sample surface, including both the cell area and the surrounding substrate. Quantitative analysis of silver intensity showed that, before sputtering, the overall silver intensity, Isubstrate + Icell, increased from control (193) to AgNO3 (382), then to 1 μM AgNP (491), and to 5 μM AgNP (1045) treatments (Table 3). After the short sputtering, most of the silver from the substrate was removed, while the silver from the cell area remained unaffected (ESI† Fig. S7). This was further confirmed by the quantitative analysis showing that the sputtering significantly decreased the total silver intensity (Isubstrate + Icell) in all samples, whereas the silver intensity from the cell area (Icell) was not affected by the sputtering procedure (Table 3). A comparison of Icell after sputtering can be visualized directly from the silver spectrum (Fig. 5, right column). In the control (Fig. 5A) and the AgNO3-exposed cells (Fig. 5B), the silver intensity was found to be very low. For 1 μM (note that two cells were analyzed, Fig. 5C) and 5 μM AgNPs (Fig. 5D), an increased silver intensity was detected with a distinct silver peak representing the silver counts from the cell area. Silver was found to be distributed homogeneously on the cell surface. The Icell of 5 μM AgNP-exposed cells was more than 2 times higher compared to that of 1 μM AgNP-exposed cells (Table 3). The intensity ratio, Icell′/Isubstrate′, was calculated with respect to the corresponding cell or substrate area (Table 3). The ratio increased from AgNO3 (3.6) to 1 μM AgNPs (5.5), and then to 5 μM AgNPs (18.0).
Table 3 Quantitative analysis of silver intensity (107Ag+ + 109Ag+) in the control cells, cells exposed to 250 nM AgNO3, 1 μM and 5 μM AgNPs, before and after sputtering
Treatment |
Ag from total areaa(Isubstrate + Icell) |
Ag from cell area(Icell) |
Intensity ratiobIcell′/Isubstrate′ |
Before |
After |
Before |
After |
Before |
After |
A total area of 150 × 150 μm2 was analyzed.
The intensity ratios were normalized by the respective areas. Icell′ = Icell/cell area. Isubstrate′ = Isubstrate/substrate area.
|
Control |
193 |
50 |
3 |
4 |
0.4 |
1.7 |
250 nM AgNO3 |
382 |
55 |
7 |
8 |
0.4 |
3.6 |
1 μM AgNPs |
491 |
219 |
70 |
69 |
2.0 |
5.5 |
5 μM AgNPs |
1045 |
289 |
201 |
154 |
3.8 |
18.0 |
Discussion
Since nanoparticles may display different agglomeration behaviours in aqueous solutions,24,25 and the size of nanoparticles is expected to influence the biological effects, we first characterized the AgNP suspensions in the exposure medium used in the experiments. Assessing the size of the AgNPs showed that the nanoparticles remained stable over 4 h of exposure with a mean size of 47 nm. The UV-vis spectra, which showed maximal absorbance at around 410 nm (Fig. S1†), confirmed that the AgNPs did not agglomerate during that time. Also, the fraction of dissolved silver after 2 h of exposure in the medium varied only slightly for the different AgNP concentrations, between 0.5 and 3.5% as determined by ultracentrifugation and between 1.3 and 2.6% as determined by membrane filtration. Similar values of dissolved silver were previously determined with the AgNPs dispersed in MOPS as well as other media containing inorganic salts and organic substances,26–28 indicating that there was little particle dissolution.
Both AgNPs and AgNO3 were proven to decrease the photosynthetic yield. The inhibition of photosynthesis was concentration-dependent, with EC50 values of 85 nM for AgNO3 and 1858 nM for AgNPs after 1 h of exposure. The EC50 values of AgNPs determined in our study were within the range of the EC50s (43–4800 nM) reported for other algal species.4,5,29 Based on the total silver concentrations in the exposure medium, AgNO3 was found to be more toxic than AgNPs, with a 22 times lower EC50 value compared to that of AgNPs. With reference to previous studies showing that the toxicity of AgNPs to algae was dependent on the dissolved Ag in the exposure medium,7–9,29 we hypothesized that also in E. gracilis, the dissolved Ag was a major contributor to AgNP toxicity. Experiments were carried out in the presence of cysteine, in order to complex the dissolved Ag and decrease Ag bioavailability. Accordingly, the effects on photosynthesis were completely prevented, thus supporting our hypothesis that the toxicity of AgNPs was due to dissolved Ag. Recalculation of the photosynthetic yield based on the measured values of dissolved Ag shifted the AgNP concentration–response curve to lower effective concentrations than for AgNO3, although the extent of this difference depended on the chosen percentage of dissolution (ESI† Table S1). The lower EC50s of AgNPs might be explained by increased AgNP dissolution upon interaction with algal exudates, such as H2O2.30,31
AgNPs and AgNO3 also affected the morphology of E. gracilis cells. The cells exhibited an irregular round morphology which coincided with the doubling of cell volume, reflecting an algal stress response induced by silver. The alteration of algal cell morphology and increase in cell size upon exposure to silver were reported with the marine alga Chattonella marina.31 The addition of cysteine abolished the morphological effects in E. gracilis, further confirming that the dissolved Ag was the determinant factor of AgNP toxicity. The enlargement of cells might result from unspecific interactions of silver ions with the thiol groups of glycoproteins, which are the major components of the E. gracilis pellicle.19 Interactions with glycoproteins of the cell wall were also suggested from a study evidencing the regulation of cell wall proteins in C. reinhardtii exposed to similar concentrations of AgNO3.32
Cell-associated silver ({Ag}cell) was measured after 1 h of exposure to AgNO3 and AgNPs. Upon exposure to nanomolar concentrations of AgNO3 (25–500 nM), silver accumulated up to 35–150 μmol Lcell−1, resulting in high bioconcentration factors (BCFs, ESI† Table S2) up to 1713 L Lcell−1. The estimated BCFs in our study were comparable to the values reported for the alga C. reinhardtii (803–2246 L Lcell−1).18 In the case of AgNPs (1–10 μM), an increase in {Ag}cell was measured up to 2.5 μM AgNP exposure, and then the {Ag}cell remained almost constant with a maximal value of 513 μmol Lcell−1. The {Ag}cell measured in the AgNP-exposed algae may have derived from the AgNPs and dissolved Ag present in the AgNP suspensions. Thus, the {Ag}cell of AgNP and AgNO3 exposed algae was plotted as a function of dissolved Ag (Fig. 4C and S4†), and the fraction derived from dissolved Ag in the AgNP suspensions was subtracted based on the {Ag}cell measured upon AgNO3 exposure at the same dissolved Ag concentrations (ESI† Table S2). Since ICP-MS measurements do not inform whether the measured silver is derived from the ionic or particulate form, the {Ag}cell after subtraction was calculated to correspond to 68–289 AgNPs per cell, based on the mean nanoparticle size of 47 nm. On the other hand, assuming that the {Ag}cell corresponded to the uptake of dissolved Ag only, up to 44% of AgNPs should have dissolved in the exposure medium, which by far exceeded the experimentally determined values (0.5–3.5%). In the study of C. reinhardtii, the measured {Ag}cell after AgNP exposure was calculated to correspond to a maximal number of 2–10 nanoparticles per cell, or to 0.4–2.1% increased AgNP dissolution,18 indicating that the {Ag}cell was mostly derived from dissolved Ag. We therefore assumed that the {Ag}cell measured in E. gracilis exposed to AgNPs reflected the AgNPs tightly adsorbed to the cells.
Despite the apparently strong association of AgNPs with E. gracilis cells, several lines of evidence support the notion that the AgNPs were not internalized, but were instead strongly attached to the pellicle. First, the cell-associated AgNPs were not toxic to the algae. For instance, at the same concentration of {Ag}cell, photosynthesis was significantly inhibited upon exposure to AgNO3, whereas no inhibition was observed with AgNP exposure (ESI† Fig. S5). Moreover, at the same inhibition level, the {Ag}cell was 3.6 times higher upon exposure to AgNPs compared to that obtained upon AgNO3 exposure. Secondly, qualitative and quantitative surface analysis by ToF-SIMS revealed the sorption of AgNPs onto the pellicle. This technique has been recently used to explore the interactions of nanoparticles with mammalian cells.33–36 The short sputtering (5.2 s) applied during the measurement led to the ablation of a few nanometers from the topmost layer of the cell, while the thickness of the E. gracilis pellicle was estimated to be 30–40 nm,37 thus the ToF-SIMS analysis only focused on the surface of the cells. The removal of silver from the substrate but not from the cell area suggests that there were two distinct sources, silver ions and AgNPs, contributing to the detected silver signals before sputtering (Table 3, ESI† Fig. S7). The silver ions were easily removed by the sputtering procedure, which is typical for loosely adsorbed atoms or small molecules,38 while AgNPs as large assemblies were much harder to sputter away. The analysis of the intensity ratio demonstrated a 2.8–4.8 times increase in silver intensity per cell area to substrate area after sputtering, indicating strong adsorption of AgNPs on the cells (Table 3). The silver intensity from the cell area after sputtering was more than doubled upon exposure to 5 μM AgNPs compared to that upon exposure to 1 μM AgNPs (Fig. 5 and Table 3), supporting the fact that the amount of AgNPs adsorbed onto the pellicle was related to the exposure concentration. Whether the sorption was due to specific interactions with E. gracilis pellicle proteins or physical restraint within the pellicle stripes cannot be sorted out. The surface of the algae may play an important role in governing their interaction with the nanoparticles. In the case of O. danica which has no cell wall or pellicle, AgNPs were visualized to be inside the cells.10 In C. reinhardtii, which has a rigid cell wall mainly composed of glycoproteins, no evidence for uptake of AgNPs was seen.18 Our results therefore highlight the importance of taking the large diversity in cell wall composition and surface architecture among different algal species into account.
Acknowledgements
This work was supported by the Swiss National Science Foundation in the framework of the Swiss National Research Program 64 on the Opportunities and Risks of Nanomaterials. We thank David Kistler (Department of Environmental Toxicology, Swiss Federal Institute of Aquatic Science and Technology, Eawag, Dübendorf, Switzerland) for ICP-MS measurements.
References
- R. Kaegi, B. Sinnet, S. Zuleeg, H. Hagendorfer, E. Mueller, R. Vonbank, M. Boller and M. Burkhardt, Environ. Pollut., 2010, 158, 2900–2905 CrossRef CAS PubMed.
- T. M. Benn and P. Westerhoff, Environ. Sci. Technol., 2008, 42, 4133–4139 CrossRef CAS.
- E. Navarro, A. Baun, R. Behra, N. B. Hartmann, J. Filser, A. J. Miao, A. Quigg, P. H. Santschi and L. Sigg, Ecotoxicology, 2008, 17, 372–386 CrossRef CAS PubMed.
- J. Fabrega, S. N. Luoma, C. R. Tyler, T. S. Galloway and J. R. Lead, Environ. Int., 2011, 37, 517–531 CrossRef CAS PubMed.
- O. Bondarenko, K. Juganson, A. Ivask, K. Kasemets, M. Mortimer and A. Kahru, Arch. Toxicol., 2013, 87, 1181–1200 CrossRef CAS PubMed.
- R. Behra, L. Sigg, M. J. Clift, F. Herzog, M. Minghetti, B. Johnston, A. Petri-Fink and B. Rothen-Rutishauser, J. R. Soc., Interface, 2013, 10, 20130396 CrossRef PubMed.
- E. Navarro, F. Piccapietra, B. Wagner, F. Marconi, R. Kaegi, N. Odzak, L. Sigg and R. Behra, Environ. Sci. Technol., 2008, 42, 8959–8964 CrossRef CAS.
- A. J. Miao, K. A. Schwehr, C. Xu, S. J. Zhang, Z. Luo, A. Quigg and P. H. Santschi, Environ. Pollut., 2009, 157, 3034–3041 CrossRef CAS PubMed.
- F. Ribeiro, J. A. Gallego-Urrea, K. Jurkschat, A. Crossley, M. Hassellov, C. Taylor, A. M. Soares and S. Loureiro, Sci. Total Environ., 2014, 466–467, 232–241 CrossRef CAS PubMed.
- A. J. Miao, Z. Luo, C. S. Chen, W. C. Chin, P. H. Santschi and A. Quigg, PLoS One, 2010, 5, e15196 CAS.
- R. J. Griffitt, J. Luo, J. Gao, J. C. Bonzongo and D. S. Barber, Environ. Toxicol. Chem., 2008, 27, 1972–1978 CrossRef CAS PubMed.
- A. Heredia, R. Guillen, A. Jimenez and J. Fernandezbolanos, Rev. Esp. Cienc. Tecnol. Aliment., 1993, 33, 113–131 CAS.
- J. P. Knox, FASEB J., 1995, 9, 1004–1012 CAS.
- D. S. Domozych, M. Ciancia, J. U. Fangel, M. D. Mikkelsen, P. Ulvskov and W. G. T. Willats, Front. Plant Sci., 2012, 3 Search PubMed.
- A. Fleischer, M. A. O'Neill and R. Ehwald, Plant Physiol., 1999, 121, 829–838 CrossRef CAS PubMed.
- M. N. Moore, Environ. Int., 2006, 32, 967–976 CrossRef CAS PubMed.
- G. T. Cole and M. J. Wynne, J. Phycol., 1974, 10, 397–410 Search PubMed.
- F. Piccapietra, C. G. Allue, L. Sigg and R. Behra, Environ. Sci. Technol., 2012, 46, 7390–7397 CrossRef CAS PubMed.
- Y. Nakano, Y. Urade, R. Urade and S. Kitaoka, J. Biochem., 1987, 102, 1053–1063 CAS.
- J. R. Sommer, J. Cell Biol., 1965, 24, 253–257 CrossRef CAS.
- S. Le Faucheur, R. Behra and L. Sigg, Environ. Toxicol. Chem., 2005, 24, 1731–1737 CrossRef CAS PubMed.
- S. Shigeoka, T. Onishi, Y. Nakano and S. Kitaoka, J. Gen. Microbiol., 1987, 133, 25–30 CAS.
- T. E. Shehata and E. S. Kempner, J. Bacteriol., 1978, 133, 396–398 CAS.
- R. Behra and H. Krug, Nat. Nanotechnol., 2008, 3, 253–254 CrossRef CAS PubMed.
- M. Farre, J. Sanchis and D. Barcelo, TrAC, Trends Anal. Chem., 2011, 30, 517–527 CrossRef CAS PubMed.
- Y. Yue, R. Behra, L. Sigg, P. Fernandez Freire, S. Pillai and K. Schirmer, Nanotoxicology, 2015, 9, 54–63 CrossRef CAS PubMed.
- C. Gil-Allue, K. Schirmer, A. Tlili, M. O. Gessner and R. Behra, Environ. Sci. Technol., 2015, 49, 1165–1172 CrossRef CAS PubMed.
- F. Piccapietra, L. Sigg and R. Behra, Environ. Sci. Technol., 2012, 46, 818–825 CrossRef CAS PubMed.
-
F. Piccapietra, Doctor of Sciences Ph.D. Thesis, ETH Zurich, 2012 Search PubMed.
- G. Suarez, C. Santschi, O. J. F. Martin and V. I. Slaveykova, Biosens. Bioelectron., 2013, 42, 385–390 CrossRef CAS PubMed.
- D. He, J. J. Dorantes-Aranda and T. D. Waite, Environ. Sci. Technol., 2012, 46, 8731–8738 CrossRef CAS PubMed.
- S. Pillai, R. Behra, H. Nestler, M. J. Suter, L. Sigg and K. Schirmer, Proc. Natl. Acad. Sci. U. S. A., 2014, 111, 3490–3495 CrossRef CAS PubMed.
- F. Draude, S. Galla, A. Pelster, J. Tentschert, H. Jungnickel, A. Haase, A. Mantion, A. F. Thunemann, A. Taubert, A. Luch and H. F. Arlinghaus, Surf. Interface Anal., 2013, 45, 286–289 CrossRef CAS PubMed.
- A. Haase, H. F. Arlinghaus, J. Tentschert, H. Jungnickel, P. Graf, A. Mantion, F. Draude, S. Galla, J. Plendl, M. E. Goetz, A. Masic, W. Meier, A. F. Thunemann, A. Taubert and A. Luch, ACS Nano, 2011, 5, 3059–3068 CrossRef CAS PubMed.
- B. Hagenhoff, D. Breitenstein, E. Tallarek, R. Mollers, E. Niehuis, M. Sperber, B. Goricnik and J. Wegener, Surf. Interface Anal., 2013, 45, 315–319 CrossRef CAS PubMed.
- P. L. Lee, B. C. Chen, G. Gollavelli, S. Y. Shen, Y. S. Yin, S. L. Lei, C. L. Jhang, W. R. Lee and Y. C. Ling, J. Hazard. Mater., 2014, 277, 3–12 CrossRef CAS PubMed.
- R. Vismara, L. Barsanti, P. Lupetti, V. Passarelli, D. Mercati, R. Dallai and P. Gualtieri, Tissue Cell, 2000, 32, 451–456 CrossRef CAS.
-
J. C. Vickerman and D. Briggs, ToF-SIMS: Materials Analysis by Mass Spectrometry–2nd Edn SurfaceSpectra, Manchester and IM Publications, Chichester, 2013 Search PubMed.
Footnote |
† Electronic supplementary information (ESI) available. See DOI: 10.1039/c5en00093a |
|
This journal is © The Royal Society of Chemistry 2015 |