DOI:
10.1039/C5QI00216H
(Research Article)
Inorg. Chem. Front., 2016,
3, 313-319
Assembled 3D electrocatalysts for efficient hydrogen evolution: WSe2 layers anchored on graphene sheets†
Received
26th October 2015
, Accepted 27th November 2015
First published on 3rd December 2015
Abstract
A new 3D composite electrocatalytic material is produced by growing WSe2 thin layers vertically on 2D reduced graphene oxide nanosheets. The material has improved electrode/electrolyte interactions and efficient catalytic properties due to the rich defects, a 3D hybrid configuration, and the direct integration of WSe2 onto the graphene sheets, which can significantly prevent the aggregation of WSe2 and promote the conductivity of the material. As demonstrated, the as-harvested WSe2/graphene hybrid exhibits excellent electrocatalytic activity in the hydrogen evolution reaction (HER), such as a low onset overpotential of −100 mV, a small Tafel slope of 64 mV per decade and outstanding stability.
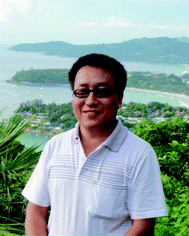 Yaping Du | Ya-Ping Du is currently a full professor and principle investigator at the Frontier Institute of Science and Technology of Xi'an Jiaotong University. His research interests include rare earth functional materials, colloidal inorganic nanocrystals, and energy storage and conversion applications. He has published more than 40 papers in peer-reviewed scientific journals and was a winner of the National Science Fund for Excellent Young Scholars in 2015. He obtained his BSc degree and PhD degree from Lanzhou University and Peking University in 2004 and 2009, respectively, and has been an assistant professor at the Suzhou Institute of Nano-tech and Nano-bionics, CAS, during 2009–2010. He did his postdoctoral research in the Nanyang Technological University of Singapore during 2010–2013. |
Introduction
Hydrogen is a promising candidate for the development of new, clean, and efficient energy carriers due to its high energy density.1,2 Currently, an attractive hydrogen production method through electrolysis stands out; running electricity through water to separate out the hydrogen and oxygen atoms. To make this process feasible, highly efficient and low-cost electrocatalysts need to be explored and developed. Although platinum (Pt) has been demonstrated to be an efficient electrocatalyst for the hydrogen evolution reaction (HER), the scarcity and high cost limit its practical application.3,4 Transition metal dichalcogenides (TMDs) are being envisaged for HER use due to their inexpensive cost and excellent electrochemical stability, and therefore great efforts have been dedicated to improving the HER performance of TMDs.5–8
As an important class of TMDs, tungsten selenide (WSe2) has a typical layered crystal structure, where each layer of W atoms is sandwiched between two layers of hexagonally close-packed Se atoms, and the atomic layers are stacked by weak van der Waals interactions.9 Additionally, WSe2 possesses a smaller band gap of ∼1.6 eV and exhibits excellent electrical transport performance in the category of TMD materials.10,11 Both theoretical calculations and experimental results have already highlighted that the HER activity of TMD materials arises from the active edge sites, indicating that the unsaturated dichalcogenide atoms on the edges play an important role in HER catalysis.12–15 However, the layered TMD materials can be easily re-stacked due to the intrinsic interlayer vdW attractions, as a result of reducing the active edge sites.16 Therefore, in order to further improve the catalytic performance, lots of efforts has been made to preserve the two-dimensional (2D) TMD nanostructures and introduce the desired defects, thus to design more active sites for promoting the HER activity. For instance, Cui's group developed layered vertically aligned 2D WSe2 nanofilms, exposing more active edge sites.17 He's group and Wang's group synthesized WS2(1−x)Se2x and WSe2 on carbon fibres, and assembled them as a working electrode, which exhibited promising HER performance owing to the abundant catalytic edge sites and enhanced charge transfer efficiency.18,19
In the present study, for the first time, we established a facile one-step colloidal synthesis method to fabricate a three-dimensional (3D) WSe2/rGO hybrid. This hybrid electrocatalyst was formed by the selective growth of WSe2 layers on 2D reduced graphene oxide (rGO) to obtain the assembled 3D configuration (Fig. S1, ESI†). Due to rGO as a unique support and the resulting 3D architecture, the aggregation of the WSe2 layers can be significantly prevented and thus facilitating electron transfer as well as exposing more catalytic edge sites. As a proof of concept application, the performance of the WSe2/rGO hybrid for the HER was evaluated (Scheme 1), which exhibited a superior HER activity with a low onset overpotential of −100 mV and a small Tafel slope of 64 mV per decade. Importantly, the HER activity of the WSe2/rGO hybrid can be maintained for at least 48 h without degradation under rigid acidic conditions.
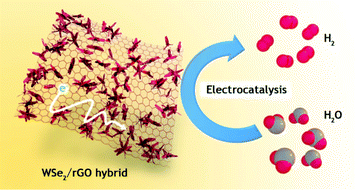 |
| Scheme 1 Schematic illustration of the 3D WSe2/rGO hybrid assembled from layered WSe2 anchored on graphene nanosheets and its application in the HER. | |
Experimental section
Chemicals
Ammonium tungstate, ((NH4)2WO4, 99.9%) and selenium powder (Se, 99.5%) were purchased from Alfa Aesar. Oleylamine, (OM, 70%), oleic acid (OA, 90%), 1-dodecanethiol (1-DDT, 98%) and 15% Pt on charcoal (Pt/C) were purchased from Sigma-Aldrich. Ethanol (AR) and cyclohexane (AR) were purchased from the Sinopharm Chemical Reagent Company. All chemicals were used as received without further purification. The nanocrystals were synthesized using standard air-free procedures.
Synthesis of WSe2 layers
A typical procedure is described as follows: first, a given amount of 0.2 mmol of (NH4)2WO4 and 16 mmol of oleylamine (OM) in a three-necked flask (100 mL) were heated to 120 °C to remove water and oxygen. This solution was marked as solution A. 4 mmol of OM, 10 μL of 1-dodecanethiol (1-DDT) and 0.6 mmol of Se powder were deposited in a separate flask, bubbled with nitrogen (N2) three times, and then treated with ultrasound under a N2 atmosphere to dissolve the Se powder. This solution was labeled as solution B. Once the Se powder was dissolved, solution B was injected into solution A under vigorous stirring. After injection, the resulting mixture was heated to 280 °C under N2 and kept at that temperature for 60 min. Upon cooling to room temperature, the products were precipitated by adding an excess of ethanol and separated from the solution by centrifugation (9500 rpm, 10 min), followed by drying in an oven at 60 °C. To remove the organic residue and excess selenium powder, the as-prepared products were annealed in Ar/H2 (95%
:
5%) at 500 °C for 1 h.
Growth of WSe2 layers on graphene
GO was made according to a modified Hummers method.20 Then, for the functionalization of GO: 5 ml of OM and 10 ml of toluene were added to 20 mg of oven-dried GO. Subsequently, the mixture was sonicated for 30 minutes until GO was homogeneously dispersed in the solution, and then the dispersion was centrifuged at 9000 rpm for 10 minutes to collect the sediment. The sediment, referred to as OM–GO, was re-dispersed in 5 mmol of OM and kept as a stock solution. The following steps were similar to the preparation of WSe2 except for adding 5 mmol of the OM–GO stock solution prepared above. For comparison, the other two WSe2/rGO hybrid nanostructures with different graphene and WSe2 ratios were prepared: one with 0.2 mmol of (NH4)2WO4, 0.6 mmol of Se and 10 mg of GO; and another with 0.2 mmol of (NH4)2WO4, 0.6 mmol of Se and 30 mg of GO.
Characterization
TEM images were acquired using a Hitachi HT-7700 transmission electron microscope (TEM, Japan) operating at 100 kV. High-resolution TEM (HRTEM) micrographs were obtained with a Philips Tecnai F20 FEG-TEM (the USA) operated at 200 kV. Samples for the TEM analysis were prepared by drying a drop of cyclohexane solution containing the nanomaterials on the surface of a carbon-coated copper grid. The Raman spectrum of the powder samples were recorded on a LabRAM HR Raman microscope with a laser excitation wavelength of 532 nm. The X-ray diffraction (XRD) patterns were obtained using a Rigaku D/MAX-RB with monochromatized Cu Kα radiation (λ = 1.5418 Å) in the 2θ range from 10° to 80°. X-ray photoelectron spectroscopy (XPS) was conducted using a PHI Quantera SXM instrument equipped with an Al X-ray excitation source (1486.6 eV). Binding energies (BEs) are referenced to the C 1s of the carbon contaminants at 284.6 eV. All samples were prepared by depositing a thin layer of products onto a cleaned Si wafer and drying at room temperature.
Electrocatalytic measurements for the HER
Typically, 4 mg of the sample and 30 μL of Nafion solution (5 wt%) were dispersed in 1 mL of water–ethanol solution with a volume ratio of 1
:
1 by sonicating for 1 h to form a homogeneous ink. Then, 5 μL of the dispersion (containing 20 μg of catalyst) was loaded onto a rotating disk electrode (RDE) with a 3 mm diameter (loading ca. 0.285 mg cm−2). Linear sweep voltammetry with a scan rate of 2 mV s−1 was conducted in 0.5 M H2SO4 (purged with pure N2) using an electrochemical cell setup, with a saturated calomel electrode (Hg/HgCl2 in saturated KCl) as the reference electrode, a Pt plate as the counter electrode, and a RDE as the working electrode with a rotating speed of 1600 rpm. All the potentials were converted to values with reference to a reversible hydrogen electrode (RHE). Cyclic voltammetry was conducted at room temperature using the same standard three-electrode setup ranging from 0.3 to 0.4 V (vs. RHE) at a scan rate of 20, 40, 60, etc. mV s−1. The time-dependent current density curve of WSe2 and the WSe2/rGO hybrid was conducted under a static overpotential of −700 mV (vs. SCE) for the continuous operation of 48 h. Nyquist plots of the WSe2 layers and WSe2/rGO hybrid were measured on the AUTOLAB PGSTAT204 electrochemistry workstation in the frequency range from 0.1 Hz to 100 kHz at an open circuit potential with 10 mV as the amplitude potential. The WSe2/rGO hybrid and pure WSe2-coated CFPs (1 cm2, catalyst loading 1 mg) were used as working electrodes to collect chronoamperometry data at the applied potential of −0.7 V vs. SCE.
Results and discussion
Fig. 1a shows the X-ray diffraction (XRD) patterns of the WSe2 layers and WSe2/rGO hybrid. All of the diffraction peaks corresponding to the typical hexagonal WSe2 (space group: P63/mmc, a = b = 0.329 nm, c = 1.298 nm, JCPDS: 38-1388) are detected in the WSe2/rGO hybrid, and no diffraction peaks from any other chemical species are detectable in both the WSe2 layers and WSe2/rGO hybrid samples. The nearly similar XRD patterns for the WSe2 layers and WSe2/rGO hybrid shown in Fig. 1a may be due to the high crystallization of WSe2, and as a result the XRD signals of rGO are too weak to observe. The transmission electron microscopy (TEM) and scanning electron microscopy (SEM) images shown in Fig. 1b and S2a† demonstrate the formation of WSe2 with 2D layered structures. As seen from the SEM and TEM images shown in Fig. 1c and d, the rGO nanosheets are decorated with uniform WSe2 layers and no free WSe2 was left in the solution (Fig. S2b, ESI†), indicating that the graphene nanosheets afford a good platform for the nucleation and subsequent growth of WSe2 layers. The selective growth of WSe2 layers on rGO is possible due to the precursors that are anchored to GO through functional groups (e.g. carboxyl, hydroxyl, and epoxy), and the defects on the graphene act as the nucleation sites.12,21–23 Notably, the vertical WSe2 layers could be clearly visible in the enlarged SEM image (inset of Fig. 1c) of the WSe2/rGO hybrid. Such a nanostructured material can offer a much rougher surface, reduce the solid–gas interaction and thereby enhance the HER performance.24,25 The energy dispersive X-ray spectroscopy (EDS) data (Fig. S2c, ESI†) verifies the stoichiometric atomic ratio for W
:
Se in the as-formed WSe2 (W
:
Se = 1
:
2).
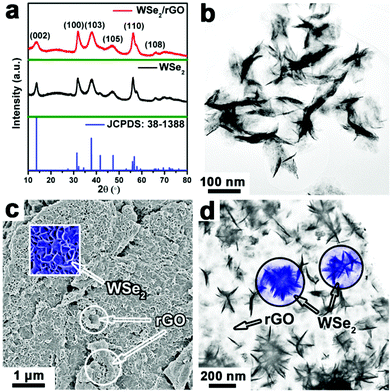 |
| Fig. 1 (a) XRD patterns, (b) TEM image of the WSe2 layers, (c) SEM and (d) TEM images of the WSe2/rGO hybrid. The square inset of (c) is the enlarged SEM image and the circular insets in (d) show the TEM image of WSe2 grown on rGO sheets. | |
The high-resolution TEM (HRTEM) images taken from different positions on the WSe2 layers grown on rGO show clear lattice fringes, demonstrating the as-obtained WSe2 layers are of high crystallinity (Fig. 2a and b). As seen from the planar orientation (Fig. 2a), WSe2 shows lattice fringes with interplane spacings of ∼0.28 nm and the fast Fourier transformation (FFT) pattern (inset of Fig. 2a) exhibits six independent diffraction arcs, which can all be indexed to the (100) plane of hexagonal (2H-type) WSe2. Moreover, the present diffraction arcs of the FFT pattern can be attributed to the quasiperiodic structure with rich defects.26 Meanwhile, the layered structure with interlayer spacings of ∼0.64 nm and ∼0.28 nm can be observed from the vertically stacked WSe2 layers (Fig. 2b), corresponding to the (002) and (100) plane of WSe2, respectively, while the FFT pattern (inset of Fig. 2b) reveals the orientation of the layers along the <001> direction. Importantly, the HRTEM images also demonstrate that there are lots of defects (highlighted in the circular areas of Fig. 2a and b) in the present WSe2 layers, which are expected to serve as active sites to improve the HER catalytic performance.26,27–29 The corresponding crystal structure model shown in Fig. 2c–e can foster the understanding of the as-harvested WSe2 structure illustrated in the HRTEM images. Viewed from the <001> direction (Fig. 2d), WSe2 holds the graphene-like structure and the (100) plane is labeled, which is consistent with the HRTEM image shown in Fig. 2a. Moreover, viewed from the <010> direction (Fig. 2e), just like the vertically standing WSe2 layers, the (100) and (002) planes are marked and also agree well with the HRTEM image shown in Fig. 2b.
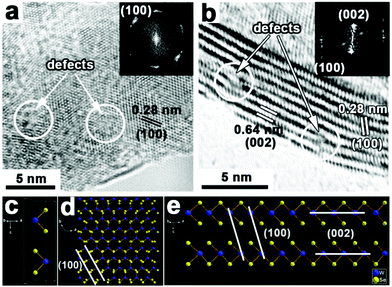 |
| Fig. 2 HRTEM images of (a) planar and (b) vertically stacked WSe2 layers grown on rGO. Insets of (a) and (b) are the corresponding FFT patterns. (c) The unit cell of hexagonal WSe2 with its atom layer from the <001> direction Se–[Se–W–Se–Se–W–Se]–Se. The modeled structure of WSe2 viewed from the (d) <001> and (e) <010> direction. | |
The detailed structure and chemical state for the WSe2/rGO hybrid were investigated using Raman spectroscopy and X-ray photoelectron spectroscopy (XPS). Fig. 3a shows the Raman spectrum of the WSe2/rGO hybrid; due to the small energy difference between the A1g (out-of-plane) (253 cm−1) and E12g (inplane) mode (250 cm−1), there is only one broad peak situated from 200 to 400 cm−1.30 Meanwhile, the characteristics of the graphene Raman shift for D, G, and 2D bands can be observed in the WSe2/rGO hybrid (Fig. 3a).31 The XPS spectrum of W is shown in Fig. 3b, and the binding energies of W 4f7/2 and W 4f5/2 located at 32.3 and 34.5 eV indicated that the elemental chemical state of W is mainly quadrivalent for the WSe2/rGO hybrid.32 Meanwhile, the small peak located at 37.7 eV is ascribed to the core levels of W 5p3/2 from WO3 due to the partial oxidation of the WSe2 layers.17 Two intense peaks at 54.1 and 54.9 eV (Fig. 3c) attributable to the core levels of Se 3d5/2 and Se 3d3/2, respectively, are characteristic of Se2− of the WSe2/rGO hybrid.33,34 The C 1s XPS signal shown in Fig. 3d demonstrated that there was a trace amount of oxygen content in the WSe2/rGO hybrid, confirming the reduction of GO to rGO.35
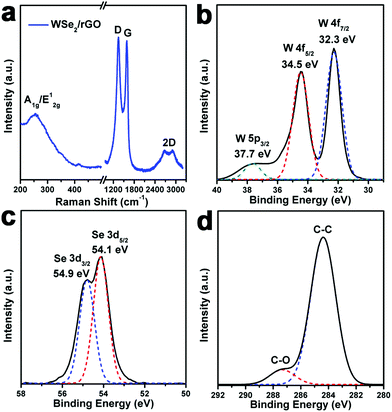 |
| Fig. 3 Raman and XPS spectra of the WSe2/rGO hybrid. (a) A1g and E12g Raman modes of the WSe2/rGO hybrid, and D, G, and 2D bands for rGO nanosheets. (b–d) XPS spectra of W 4f, Se 3d and C 1s signals recorded for the WSe2/rGO hybrid. | |
In the present synthesis, the combination of capping ligands (OA and OM) and the amount of the Se powder precursor were found to play crucial roles in the formation of high-quality WSe2 products. For example, fixing the amount of solvent (20 mmol) and the mole ratio of (NH4)2WO4 and the Se powder ((NH4)2WO4
:
Se = 1
:
3), when using 0.2 mmol of (NH4)2WO4, 0.6 mmol of Se and pure OM as a solvent at 280 °C for 60 min, the pure phase WSe2 products were obtained (Fig. 1a). Under the same reaction conditions, except using OA as the solvent (e.g. OM
:
OA = 1
:
1 and pure OA), the harvested products possessed a layered structure (Fig. S3a and b, ESI†), but coexisted with a small amount of tungsten oxide (WO3) (Fig. S3c, ESI†). In addition, when the mole ratio of (NH4)2WO4 and the Se powder was larger than 1
:
3 (e.g. 1
:
2, 0.2 mmol of (NH4)2WO4, 0.4 mmol of Se) in pure OM at 280 °C for 60 min, the as-prepared samples also contained considerable impurities with nanorod morphologies (Fig. S4a and b, ESI†). To conclude, after a series of condition based experiments, we found that high-quality WSe2 layers can be obtained in 20 mmol of OM solvent at a temperature of 280 °C for 60 min under the precursor concentration of 0.2 mmol of (NH4)2WO4 and 0.6 mmol of Se powder, which can elegantly maintain the balance between nucleation and growth stages (Fig. 1b and S2a, ESI†).
The HER electrocatalytic performance of the as-prepared WSe2 layers and WSe2/rGO hybrid were investigated using a standard three-electrode setup with the same loading value of 0.285 mg cm−2 on a rotating disk electrode (RDE) in 0.5 M H2SO4 electrolyte degassed with N2 (experimental section). As shown in Fig. 4a, the polarization curves (iR corrected) show the current density versus voltage (J versus V) for the comparison of the WSe2 layers and WSe2/rGO hybrid along with the Pt/C (15%) samples. As depicted in Fig. 4a, only rGO had no HER activity, hinting that the core catalysts were the WSe2 layers. Compared to the pure WSe2 layers, the onset potential of the WSe2/rGO hybrid shifted from −160 mV to −100 mV and significant H2 evolution (J = 10 mA cm−2) was observed at −180 mV, which was much smaller than WSe2 (−307 mV) vs. RHE, suggesting the excellent catalytic activity of the WSe2/rGO hybrid. In order to further study the HER electrocatalytic performance of the WSe2/rGO hybrid, WSe2 layers physically mixed with rGO (WSe2 + rGO) having the same ratio as the WSe2/rGO hybrid were also investigated, which exhibited a much lower HER catalytic activity (Fig. S5a, ESI†). The superior HER catalytic performance of the WSe2/rGO hybrid compared to WSe2 + rGO may be due to the improved charge transfer efficiency between the WSe2 layers and rGO.12,36 In addition, the HER electrocatalytic activities of the WSe2/rGO hybrids with different WSe2 to rGO content ratios were measured (Fig. S5b, ESI†). When the loading density of WSe2 on rGO was higher (0.2 mmol of WSe2/10 mg of rGO), a relatively lower catalytic performance was obtained, probably due to the lower efficiency of charge transfer.26,37 On the contrary, when the loading density of WSe2 on rGO was lower (0.2 mmol of WSe2/30 mg of rGO), an inferior catalytic performance resulted from the lower loading density of the core catalysts of WSe2. Thus, 0.2 mmol of WSe2 loaded on 20 mg of graphene (0.2 mmol of WSe2/20 mg of rGO) is the optimized content ratio for fulfilling the best HER performance.
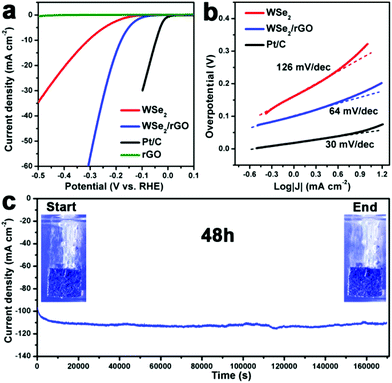 |
| Fig. 4 (a) Polarization curves of the WSe2 layers, the WSe2/rGO hybrid, rGO and Pt/C (15%). (b) The corresponding Tafel curves and (c) durability test by continuous HER recorded on the WSe2/rGO hybrid-modified CFP electrode at a static overpotential of −0.7 V vs. SCE. The catalysts were deposited on CFP with a loading concentration of 1 mg cm−2. Inset of (c): digital photos showing the H2 bubbles formed on the WSe2/rGO hybrid-modified CFP at the start and end time points (48 h). All the measurements were performed in N2 saturated 0.5 M H2SO4 electrolyte. | |
The promising enhancement in HER activity (Fig. 4b) was apparent by comparing the Tafel slopes of the WSe2/rGO hybrid (64 mV per decade, after iR correction) with those of pure WSe2 layers (126 mV per decade, after iR correction), demonstrating that the kinetic speed of electrochemical hydrogen evolution for the WSe2/rGO hybrid was much faster than for the pure WSe2 layers. This Tafel slope was also comparable to or even lower than the previously reported WSe2-based HER catalysts (Table S1†). Generally, a Tafel slope includes three principal steps in converting H+ to H2 in the HER:12,38–40
| H3O+ + e–M ↔ H–M + H2O (Volmer reaction) | (1) |
| 2H–M ↔ H2–M + M (Tafel reaction) | (2) |
| H3O+ + e–M + H–M ↔ H2–M + M + H2O (Heyrovsky reaction) | (3) |
where, e–M represents the catalyst-bound electrons and H–M and H
2–M stand for the hydrogen atom and molecule adsorbed onto the catalyst’s surface, respectively. The first step (1) is a discharge step (Volmer reaction, 120 mV dec
−1), in which protons (H
3O
+) are adsorbed onto the active sites on the catalyst’s surface and combined with electrons (e–M) to form adsorbed hydrogen atoms (H–M). The following process is the combination step (2) (Tafel reaction, 30 mV dec
−1) or desorption step (3) (Heyrovsky reaction, 40 mV dec
−1). In the present work, the Tafel slope is 64 mV per decade for the WSe
2/rGO hybrid (
Fig. 4b), indicating the Heyrovsky reaction plays a crucial role in determining the HER rate of the WSe
2/rGO hybrid.
Apart from the current density and Tafel slope, the stability of the catalyst is another important criterion for the HER. The long-term stability of the WSe2 layers and WSe2/rGO hybrid catalysts is conducted by means of continuous HER at the static overpotential of −0.7 V vs. SCE in 0.5 M H2SO4 for 48 h. The WSe2 layers and WSe2/rGO hybrid catalysts are loaded on carbon fibre paper (CFP) with a high catalyst loading concentration of 1 mg cm−2 (Experimental section). As shown in Fig. 4c, the current density of the WSe2/rGO hybrid modified CFP electrode increases gradually at the initial ∼2 h and then remains stable even after a long period of 48 h. Furthermore, digital photos (inset of Fig. 4c) taken of the WSe2/rGO hybrid modified CFP electrode at the start and end of the durability test, show the vigorous effervescence of H2 bubbles (Movie 1, ESI†). Remarkably, the XPS spectra of the WSe2/rGO hybrid after the HER for 48 h show no obvious change in the chemical state of both the W and Se elements (Fig. S6a and b, ESI†), indicating that the WSe2/rGO hybrid catalysts are rather stable in a rigid acidic environment for a long time. In comparison, under the same HER conditions, the pure WSe2 exhibited a relative fluctuation in HER activity after 11 h (Fig. S7, ESI†).
The smaller (64 mV per decade) Tafel slope, lower onset potential (−100 mV) and outstanding stability verify that the WSe2/rGO hybrid can be an effective electrocatalyst for the HER. We also performed electrochemical impedance spectroscopy (EIS) of the WSe2 layers and WSe2/rGO hybrid to investigate the electrode kinetics for the HER. The Nyquist plots of the WSe2 layers and WSe2/rGO hybrid samples are compared (Fig. 5a) and fitted to an equivalent circuit (Fig. S8, ESI†), where a constant phase element (CPE) is employed. As displayed in Fig. 5a, the Nyquist plots reveal a decrease in the charge-transfer resistance (Rct) for the WSe2/rGO hybrid (40.8 Ω) compared to the pure WSe2 layers (81.7 Ω), indicating a smaller reaction resistance and higher catalytic activity for the HER in acid solution for the WSe2/rGO hybrid electrode. In addition, as shown in the inset of Fig. 5a, the WSe2/rGO hybrid also exhibited a smaller Rs (0.4 Ω) than the WSe2 layers (0.8 Ω), demonstrating that our one-pot synthesis strategy for the growth of the WSe2 layers on graphene could also improve the electrical contact at the interface of the electrode.
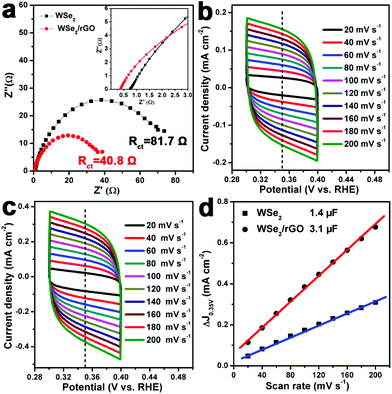 |
| Fig. 5 (a) EIS Nyquist plots. Voltammograms of the (b) WSe2 layers and (c) WSe2/rGO hybrid electrocatalysts at various scan rates (20–200 mV s−1). (d) Estimated Cdl and relative electrochemically active surface area for the WSe2 layers and WSe2/rGO hybrid electrocatalysts. The inset of (a) shows the high frequency Nyquist plots of the two catalysts. | |
We further compared the electrochemical surface area (ECSA) of the WSe2 layers and WSe2/rGO hybrid samples by measuring the double-layer capacitance (Cdl). Fig. 5b and c show the typical cyclic voltammograms (CVs) of the WSe2 layers and WSe2/rGO hybrid, respectively, taken with various scan rates (20–200 mV s−1) in the region of 0.3–0.4 V (vs. RHE) in 0.5 M H2SO4. Current density differences (ΔJ = Ja − Jc) at 0.35 V (vs. RHE) are then plotted against the scan rate. The electrochemical double-layer capacitance values, which are equivalent to half of the linear slopes in Fig. 5d, are used to represent and compare the ECSA of the WSe2 layers and WSe2/rGO hybrid. It is noteworthy that the Cdl of the WSe2/rGO hybrid (3.1 μF) is much higher than that of the WSe2 layers (1.4 μF), suggesting that the WSe2/rGO hybrid possesses a much larger active surface area and more active sites for hydrogen production.8,41
Three possible reasons could be responsible for the excellent HER performance of the 3D WSe2/rGO hybrid nanostructures. Firstly, the WSe2 layers are vertically grown on rGO, which can effectively reduce the agglomeration of the WSe2 layers, maximally exposing the active edges and reducing the solid–gas interaction. Secondly, the charge transfer efficiency at the electrode interface is greatly promoted through combining with the highly electrically conductive rGO. The last one is the defect-rich structure of the WSe2/rGO hybrid, which contributes to the additional catalytically active sites.
Conclusions
In summary, WSe2/rGO hybrid nanostructures have been constructed through a facile colloidal chemistry method and developed as HER catalyst electrodes, which exhibit excellent HER activity with a low onset potential of −100 mV, a small Tafel slope of 64 mV per decade and excellent stability. The Tafel slope of 64 mV dec−1 suggests a Volmer–Heyrovsky mechanism for the catalyzed HER. These findings can be attributed to the uniform distribution of the WSe2 layers on rGO, the high electrical conductivity of rGO and the rich defects in the hybrid structures. The work presented in this paper will spur research to improve the activity of TMD-based electrocatalysts for the HER.
Acknowledgements
We gratefully acknowledge financial aid from the start-up funding from Xi'an Jiaotong University, the Fundamental Research Funds for the Central Universities (2015qngz12), the NSFC (grant no. 21371140), and the China National Funds for Excellent Young Scientists (grant no. 21522106).
Notes and references
- J. A. Turner, Science, 2004, 305, 972 CrossRef CAS PubMed
.
- B. Winther-Jensen, K. Fraser, C. Ong, M. Forsyth and D. R. MacFarlane, Adv. Mater., 2010, 22, 1727 CrossRef CAS PubMed
.
- E. Antolini, Energy Environ. Sci., 2009, 2, 915 CAS
.
- C. L. Tan and H. Zhang, Chem. Soc. Rev., 2015, 44, 2713 RSC
.
- D. Voiry, H. Yamaguchi, J. W. Li, R. Silva, D. C. B. Alves, T. Fujita, M. W. Chen, T. Asefa, V. B. Shenoy, G. Eda and M. Chhowalla, Nat. Mater., 2013, 12, 850 CrossRef CAS PubMed
.
- J. Kibsgaard, Z. B. Chen, B. N. Reinecke and T. F. Jaramillo, Nat. Mater., 2012, 11, 963 CrossRef CAS PubMed
.
- L. Cheng, W. J. Huang, Q. F. Gong, C. H. Liu, Z. Liu, Y. G. Li and H. J. Dai, Angew. Chem., Int. Ed., 2014, 53, 7860 CrossRef CAS PubMed
.
- M. A. Lukowski, A. S. Daniel, F. Meng, A. Forticaux, L. S. Li and S. Jin, J. Am. Chem. Soc., 2013, 135, 10274 CrossRef CAS PubMed
.
- W. Zhao, Z. Ghorannevis, L. Chu, M. Toh, C. Kloc, P. H. Tan and G. Eda, ACS Nano, 2012, 7, 791 CrossRef PubMed
.
- Q. H. Wang, K. Kalantar-Zadeh, A. Kis, J. N. Coleman and M. S. Strano, Nat. Nanotechnol., 2012, 7, 699 CrossRef CAS PubMed
.
- H. J. Chuang, X. B. Tan, N. J. Ghimire, M. M. Perera, B. Chamlagain, M. M. C. Cheng, J. Q. Yan, D. Mandrus, D. Tomanek and Z. X. Zhou, Nano Lett., 2014, 14, 3594 CrossRef CAS PubMed
.
- Y. Li, H. Wang, L. Xie, Y. Liang, G. Hong and H. Dai, J. Am. Chem. Soc., 2011, 133, 7296 CrossRef CAS PubMed
.
- T. Li and G. Galli, J. Phys. Chem. C, 2007, 11, 16192 Search PubMed
.
- D. Merki, S. Fierro, H. Vrubel and X. Hu, Chem. Sci., 2011, 2, 1262 RSC
.
- T. F. Jaramillo, K. P. Jørgensen, J. Bonde, J. H. Nielsen, S. Horch and I. Chorkendorff, Science, 2007, 317, 100 CrossRef CAS PubMed
.
- K. Chang, W. Chen, L. Ma, H. Li, H. Li, F. Huang, Z. Xu, Q. Zhang and J. Lee, J. Mater. Chem., 2011, 21, 6251 RSC
.
- H. Wang, D. Kong, P. Johanes, J. J. Cha, G. Zheng, K. Yan, N. Liu and Y. Cui, Nano Lett., 2013, 13, 3426 CrossRef CAS PubMed
.
- K. Xu, F. Wang, Z. Wang, X. Zhan, Q. Wang, Z. Cheng, M. Safdar and J. He, ACS Nano, 2014, 8, 8468 CrossRef CAS PubMed
.
- M. Zou, J. Zhang, H. Zhu, M. Du, Q. Wang, M. Zhang and X. Zhang, J. Mater. Chem. A, 2015, 3, 12149 CAS
.
- D. C. Marcano, D. V. Kosynkin, J. M. Berlin, A. Sinitskii, Z. Z. Sun, A. Slesarev, L. B. Alemany, W. Lu and J. M. Tour, ACS Nano, 2010, 4, 4806 CrossRef CAS PubMed
.
- Y. Shi, W. Zhou, A. Y. Lu, W. Fang, Y. H. Lee, A. L. Hsu, S. M. Kim, K. K. Kim, H. Y. Yang, L. J. Li, J. C. Idrobo and J. Kong, Nano Lett., 2012, 12, 2784 CrossRef CAS PubMed
.
- H. Wang, J. T. Robinson, G. Diankov and H. Dai, J. Am. Chem. Soc., 2010, 132, 3270 CrossRef CAS PubMed
.
- H. Wang, L. F. Cui, Y. Yang, H. S. Casalongue, J. T. Robinson, Y. Liang, Y. Cui and H. Dai, J. Am. Chem. Soc., 2010, 132, 13978 CrossRef CAS PubMed
.
- Z. Y. Lu, Y. J. Li, X. D. Lei, J. F. Liu and X. M. Sun, Mater. Horiz., 2015, 2, 294 RSC
.
- Z. Y. Lu, W. Zhu, X. Y. Yu, H. C. Zhang, Y. J. Li, X. M. Sun, X. W. Wang, H. Wang, J. M. Wang, J. Luo, X. D. Lei and L. Jiang, Adv. Mater., 2014, 26, 2683 CrossRef CAS PubMed
.
- J. Xie, H. Zhang, S. Li, R. Wang, X. Sun, M. Zhou, J. F. Zhou, X. W. Lou and Y. Xie, Adv. Mater., 2013, 25, 5807 CrossRef CAS PubMed
.
- C. B. Roxlo, H. W. Deckman, J. Gland, S. D. Cameron and R. R. Chianelli, Science, 1987, 235, 1629 CAS
.
- W. Kautek and H. Gerischer, Surf. Sci., 1982, 119, 46 CrossRef CAS
.
- R. R. Chianelli, A. F. Ruppert, S. K. Behal, B. H. Kear, A. Wold and R. Kershaw, J. Catal., 1985, 92, 56 CrossRef CAS
.
- J. H. Yu, H. R. Lee, S. S. Hong, D. Kong, H. W. Lee, H. Wang, F. Xiong, S. Wang and Y. Cui, Nano Lett., 2015, 15, 1031 CrossRef CAS PubMed
.
- A. C. Ferrari, J. C. Meyer, V. Scardaci, C. Casiraghi, M. Lazzeri, F. Mauri, S. Piscanec, D. Jiang, K. S. Novoselov, S. Roth and A. K. Geim, Phys. Rev. Lett., 2006, 97, 187401 CrossRef CAS PubMed
.
- A. Katrib, F. Hemming, P. Wehrer, L. Hilaire and G. Maire, J. Electron. Spectrosc. Relat. Phenom., 1995, 76, 195 CrossRef CAS
.
- W. A. Abdallah and A. E. Nelson, J. Mater. Sci., 2005, 40, 2679 CrossRef CAS
.
- N. D. Boscher, C. J. Carmalt and I. P. Parkin, J. Mater. Chem., 2006, 16, 122 RSC
.
- D. Wan, C. Yang, T. Lin, Y. Tang, M. Zhou, Y. Zhong, F. Huang and J. Lin, ACS Nano, 2012, 6, 9068 CrossRef CAS PubMed
.
- Z. Liu, N. Li, H. Zhao and Y. Du, J. Mater. Chem. A, 2015, 3, 19706 CAS
.
- H. Vrubel, D. Merki and X. Hu, Energy Environ. Sci., 2012, 5, 6136 CAS
.
- W. Zhou, D. Hou, Y. Sang, S. Yao, J. Zhou, G. Li, L. Li, H. Liu and S. Chen, J. Mater. Chem. A, 2014, 2, 11358 CAS
.
- J. G. N. Thomas, Trans. Faraday Soc., 1961, 57, 1603 RSC
.
- W. Zhou, J. Zhou, Y. Zhou, J. Lu, K. Zhou, L. Yang, Z. Tang, L. Li and S. Chen, Chem. Mater., 2015, 27, 2026 CrossRef CAS
.
- D. Kong, H. Wang, Z. Lu and Y. Cui, J. Am. Chem. Soc., 2014, 136, 4897 CrossRef CAS PubMed
.
Footnote |
† Electronic supplementary information (ESI) available: SEM and TEM images of GO and optimal conditional experiments, and the comparison of HER performance for different ratio of WSe2/rGO hybrid catalysts. See DOI: 10.1039/c5qi00216h |
|
This journal is © the Partner Organisations 2016 |
Click here to see how this site uses Cookies. View our privacy policy here.