DOI:
10.1039/D3PY00261F
(Review Article)
Polym. Chem., 2023,
14, 2145-2158
Photopolymerization shrinkage: strategies for reduction, measurement methods and future insights
Received
12th March 2023
, Accepted 27th March 2023
First published on 28th April 2023
Abstract
Herein, a review of the literature on polymerization shrinkage occurring in dental composites was presented. In particular, methods of reducing polymerization shrinkage in dental composites by using alternative monomers and photoinitiators were described. Thus, new alternative materials for use in polymerization processes to obtain composites with limited polymerization shrinkage were presented. The effects of time and type of setting, as well as the type and amount of inorganic fillings, on the value of polymerization shrinkage were also discussed. It was explained why standard dental composites exhibit high polymerization shrinkage and why volume shrinkage and shrinkage stress are key factors in determining the quality of polymeric materials for dental applications. Traditional and new methods for testing polymerization shrinkage of composites were also presented. The advantages and disadvantages of methods for measuring polymerization shrinkage in dental composites were also presented and future insights were highlighted.
1. Introduction
In recent years, particular focus has on finding new solutions for photopolymerization processes has been placed.1–10 This research involves both the search for new initiator systems,7,11–14 fluorescent sensors10,15–17 to monitor the progress of the polymerization process, new resins,18,19 and new applications.17,20,21 In recent years, significant progress in the development of photo-curable dental composites obtained by photopolymerization process was contributed. The research concerned both new photoinitiators,22–26 new monomers19,27–33 for dental fillings. Nevertheless, manufacturers of dental fillings are still looking for composites with reduced polymerization shrinkage and reduced shrinkage stress. An ideal dental composite would experience zero or at least little shrinkage during setting. Zero shrinkage would ensure that the material would remain physically adherent to the tooth surface without changing dimensions afterwards if the material itself did not absorb water over time. However, the typical dimethacrylate monomers used in commercial dental composites absorb water,34 and therefore it may generally be advantageous for some composite shrinkage to occur, at least those based on the monomers, which will then be compensated by delayed expansion during service. In any event, the production of composites with low shrinkage, often described as less than 1.0% by volume, has been a manufacturers target for many years. However, the real problem with cure shrinkage, which is inevitable due to the nature of the polymerization of acrylate monomers to reduce intermolecular size and free volume, is the internal stress generated in the material.35–37 This stress is a result of the restriction of the free contraction of the polymer and is dependent on many factors including the size and nature of the monomers, the stiffness of the material during polymerization, the rate of reaction, and the external constraints imposed by tooth bonding.
This article presents the main strategies for reducing polymerization shrinkage in dental composites with an emphasis on the use of appropriate photoinitiators and monomers as well as new and standard methods of measuring polymerization shrinkage. In this article, we have also presented future insights in the design of resins with limited polymerization shrinkage (Fig. 1).
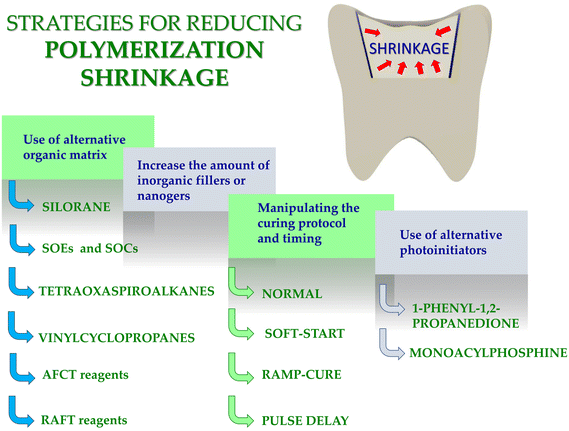 |
| Fig. 1 Scheme illustrating strategies for reducing polymerization shrinkage. | |
2. General information about polymerization shrinkage
Volumetric/polymerization shrinkage and shrinkage stress are often used interchangeably, but they have two different definitions.
Resin composites based on acrylate monomers undergo a volumetric shrinkage of 2–14% after setting, which creates 5–15 MPa contraction stresses between the dental composite and the tooth, straining the interface, leading to detachment, microcracks and bending of the nodule.38 The factor is not the polymerization shrinkage itself, but rather the stress generated by the reconstruction of the tooth interface, while the material shrinks in a confined environment such as tooth cavities or root canals.39
The greater the volumetric shrinkage, the greater the shrinkage stress for a comparable modulus of elasticity. The development of shrinkage stresses depends on the volumetric shrinkage strain and the stiffness of the composite at shrinkage; even low shrink composites can exhibit high stresses when they have a high modulus of elasticity.
It is usually recommended to apply the composite 2 mm in steps and polymerize each increment independently to ensure full depth of cure and reduce the net effect of polymerization shrinkage.40–42 It is assumed that the net shrinkage stress is lower because the smaller volume of the composite may shrink before subsequent additions. On the other hand, Versluis et al. showed that incremental application in combination with the fixed bond with the tooth increased the deformation of the reconstructed tooth, and thus the stress level within the tooth restoration complex.43 Both shrinkage stress44 and volumetric/polymerization shrinkage of dental composites is the subject of much research.
Most popular organic matrixes designated for obtaining dental composites via photopolymerization are (meth)acrylatemonomers (RCB – resin based composites) characterized by high reactivity.18
Typically, monomers that polymerize according to the radical mechanism are 2,2-bis[4-(2-hydroxy-3-methacryloxypropyl)phenyl]propane (Bis-GMA), ethoxylated Bis-GMA (EBPDMA) and 1,6-bis-[2-methacryloxycarbonylamino]-2,4,4-trimethylhexane (UDMA), dodecanedioldimethacrylate (D3MA) or triethyleneglycolmethacrylate (TEGDMA) (Fig. 2). The choice of organic matrix is of considerable importance in the production of dental fillings. Such parameters as reactivity, viscosity, water absorption, swelling and, most importantly, polymerization shrinkage depend on its choice. Unfortunately, the polymerization shrinkage of dental composites that polymerize according to the radical mechanism ranges from 2 to as much as 14%.45 The polymerization shrinkage of small-molecule monomers is more pronounced compared to macromolecular monomers; however, macromolecular monomers are very viscous (Table 1). For these reasons, polymerization shrinkage is dictated by the complex interplay of resin viscosity, polymerization rate, conversion rate and network structure evolution. Each of these parameters is important, and all of the listed parameters depend on each other, so they must be considered as a whole.
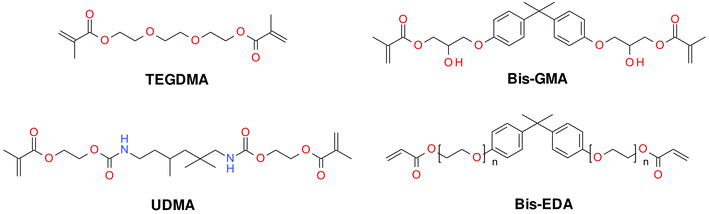 |
| Fig. 2 Examples of monomers used in commercial dental composites based on free-radical photopolymerization mechanism. | |
Table 1 Properties of standard monomers used in production of dental composites
Monomer |
Molecular weight [g mol−1] |
ρ
mon a [g cm−3] |
ρ
pol b [g cm−3] |
ΔVp [%] |
Viscosity [mPa s] |
ρmon – density of monomer.
ρpol – density of polymer.
|
TEGDMA |
286 |
1.072 |
1.250 |
−14.3 |
100 |
UDMA |
470 |
1.110 |
1.190 |
−6.7 |
5000–10 000 |
Bis-GMA |
512 |
1.151 |
1.226 |
−6.1 |
500 000–800 000 |
Moreover, because of the non-homogeneous network architecture created during the free-radical photopolymerization process, the final materials to be brittle, and the resulting shrinkage stress might lead to delamination, deformation, or mechanical failure of the final composites materials. The observed shrinkage stress evolves throughout the polymerization procedure as the applied formulation transitions from a liquid to a solid form (i.e. gel point) and is built up during vitrification until the ultimate conversion is attained. Prior to free-radical photopolymerization, the monomers are separated by van der Waal's distance (about 3.4 Å). The shrinkage stress that occurs during gelation is caused in part by the creation of covalent connections between the corresponding monomers, where the disclosing distance is only 1.5 Å.39 One of the key problems of contemporary methacrylates resin-based dental composites is incomplete free-radical photopolymerization, volumetric shrinkage, and stress. Attempts to boost double-bond conversion have often exacerbated polymerization shrinkage and polymerization stress. Furthermore, there is rising concern regarding the safe clinical use of these compounds due to their toxic effects, oral biodegradation,46 and allergic effects.47 Luo et al. proposed an alternative monomer urethane dimethacrylate monomer, which has lower polymerization shrinkage compared to BisGMA and UDMA (Fig. 3).133
 |
| Fig. 3 Structure of urethane dimethacrylate monomer. | |
On the other hand, Carlos E. Cuevas-Suárez proposed allyl carbonate monomer (BPhADAC, Fig. 4) as alternative to TEGDMA. They showed that the introduction of BPhADAC monomer in place of TEGDMA reduces polymerization shrinkage from 5.37% to 4.48%.135
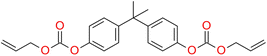 |
| Fig. 4 Structure of BPhADAC monomer. | |
Volumetric shrinkage and shrinkage stress are key factors in determining the quality of polymeric materials for dental applications. The shrinkage stress found in standard dental composites can even exceed the tensile strength of the enamel and cause stress cracks and fractures in the enamel along the interface. Such shrinkage stresses could also cause deformation of the surrounding tooth structure when the composite-tooth bond is strong, predisposing the tooth to fracture.49–51
Moreover this stress may produce defects in the composite-tooth bond, leading to bond failure, micro leakage, postoperative sensitivity, and recurrent caries. Reconstruction failures in the form of hypersensitivity, pulpitis and secondary caries may occur.50–52 In addition, the polymerization shrinkage causes mechanical stresses in the dental composites, which significantly reduces its mechanical strength.
3. Strategies for reducing polymerization shrinkage in dental composites
Many strategies to reduce polymerization shrinkage in dental composites have been developed. In particular, the polymerization shrinkage depends on:
(a) time and type of setting;
(b) type and quantity of inorganic fillings;
(c) type of organic matrix;
(d) type of photoinitiator.
Therefore, in recent years, scientists are working on strategies to reduce shrinkage stress. Below, the primary strategies for reducing the polymerization shrinkage of dental composites are presented (Fig. 1).
3.1. Time and type of setting – alternative curing methods
As the composite is polymerized under various illumination circumstances, shrinkage stress varies. Several research have shown that the modification of irradiance during the photoactivation process affects the shrinkage stress modulus.39 In order to minimize the effects of polymerization shrinkage, alternative light activation protocols have been recommended.53,54Fig. 5 presents the different dental light-curing modes.55 Soft-start techniques have been widely researched. In this type of photoactivation, low light intensity is used for a few seconds at the beginning, followed by a gradual increase in light intensity. This approach is recommended to reduce shrinkage stress, while maintaining a high degree of monomer-to-polymer reactivity.56 However, there is no correlation between time and irradiation parameters for soft-start methods.57
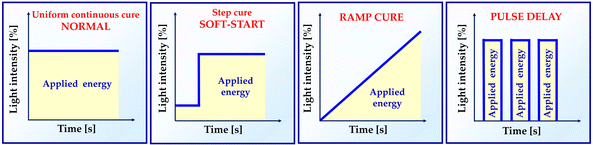 |
| Fig. 5 Different dental light-curing modes. | |
3.2. Type and quantity of inorganic fillings – the increase in the amount of filler in photocurable dental resin
Since 1950, adding more inorganic58 and/or nanogels59,60 fillers has been the main method for minimizing polymerization shrinkage, which results in a decrease in the quantity of organic matrixes in the material. One of the most used fillers is silicon dioxide, which is available in crystal, colloidal, and pyrolytic forms,61 as well as aluminum–lithium boron glass. When nanofiller is used, the amount of this component in composite materials—particularly those based on acrylic monomers—can even reach up to 90% of the mass.62 Zinc oxide is also popular. For example, Pratap et al. added different amounts of micro sized zinc oxide (0%, 3%, 6% and 9%) to the standard monomer mixture used in the production of BisGMA/TEGDMA dental composites. They then showed that polymerization shrinkage was reduced from 15.2% for compositions without filler to 8.2% for compositions with 9% inorganic filler by weight.136
Moreover polyhedraloligomericsilsesquioxane (POSS) is a filler that is increasingly being used to improve the mechanical properties of such composites (POSS). The addition of POOS to the organic matrix can significantly reduce the risk of microleakage and secondary caries.63 By adding this amount of filler to the volume of the composite, the stresses that would otherwise develop during the polymerization reaction are reduced, limiting the deleterious effects of shrinkage.
Nevertheless, by increasing the amount of the filler, the limit value when further shrinkage reduction became impossible has been obtained very quickly. Moreover, a significant amount of the filler, reaching even up to 70%, limits the depth of light penetration into the layer of the composite undergoing the process of photopolymerization, and then the entire conversion of the monomer does not occur.64 Uneven and incomplete photopolymerization of acrylates and methacrylates leads to the leaching of unreacted monomers, where diffused from saliva filling, enters the gastrointestinal tract and further into the bloodstream. Unbound monomers can cause severe allergic and cytotoxic reactions.65
3.3. Type of organic matrix – application of alternative monomers
3.3.1. Use of silorane-based monomers.
Sato et al. created organosilicon compounds containing oxirane for the first time in 1976.66 Similar compounds were studied by Crivello et al.67–69 in the early 1990s. These monomers produced effects that were both intriguing and feasible from a business standpoint. The name “siloxane” was created by 3M-ESPE to describe monomers that include both oxirane and siloxane units.70 These monomers have found applications in the dental industry. They have hydrophobic properties due to their siloxane backbone, and low value polymerization shrinkage is brought on by the cycloaliphatic oxirane molecules that make up their structure. These monomers undergo local volumetric expansion to “open up” their molecular structures. This can thus compensate for polymerization shrinkage resulting from C
C or equivalent polymerization to some extent.64 Comparing the siloxane-based composite system to methacrylates with average mechanical characteristics and high biocompatibility, the siloxane-based composite system exhibits better hydrophobicity and much lower polymerization stress and polymerization shrinkage. When siloxanes are used to create dental composites, polymerization shrinkage is guaranteed to be reduced by up to 0.94%.71
However, some studies demonstrated no beneficial effects of using siloxane-based monomers with regard to stress reduction,72,73 In particular, siloxanes have been introduced into dentistry as promising alternatives for overcoming the negative effects of the shrinkage stress of polymerization. However, these compounds are currently not as attractive as was thought just a few years ago (Fig. 6).
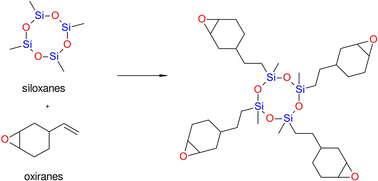 |
| Fig. 6 Scheme of obtaining siloxane monomers. | |
3.3.2. Use of spiroothoesters (SOEs) or spiroorthocarbonates (SOCs).
The next approach to reducing polymerization shrinkage is to use expanding monomers, which undergo volume expansion when polymerized. Spiroorthoesters (SOEs) and spiroorthocarbonates (SOCs) are the most widely studied expanding monomers (Fig. 7).
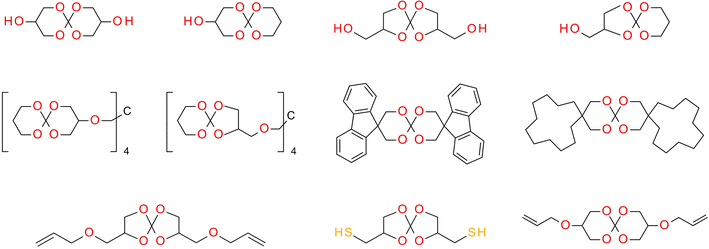 |
| Fig. 7 Structures of spiroorthoesters (SOEs) and spiroorthocarbonates (SOCs). | |
SOCs are double-cyclic acetals that polymerize when catalyzed in an acidic environment but remain stable in a basic environment. Double ring-opening photopolymerization (ROP) produces polymers from these chemicals (ethercarbonates). Due to the rise in excluded free volume brought on by the ring-opening procedure, ROP-cured bicyclic compounds often shrink less during the curing process. Bicyclic substances that can be employed as expansion co-monomers in resin-based composite formulations, such as spiro-orthocarbonates (SOCs), have been the subject of research by Bailey et al.74 Expansion (3.5%) brought on by ring-opening interactions with SOCs can counterbalance the natural contraction. Nevertheless SOCs show poor solubility, minimal copolymerization, partial ring-opening, and negligible shrinkage reduction in dimethacrylate resins.
3.3.3. Use of tetraoxaspiroalkanes.
Cecil C. Chappelow et al.75 took a step further and synthesized and evaluated a new tetraoxaspiroalkane monomer containing silicon in siloxane-based systems. They explained that tetraoxaspiroalkanes polymerize via cationic mechanism, similarly to siloxanes. They then demonstrated that formulations containing tetraoxaspiroalkane monomers exhibited photopolymerization stress that were 40–99% less than the no addition control such as silorane-based resin (methylbis[2-(7-oxabicyclo[4.1.0]hept-3-yl)ethyl]phenylsilane and 2,4,6,8-tetramethyl-2,4,6,8-tetrakis-[2-(7-oxabicyclo[4.1.0]hept-3-yl)ethyl]-1,3,5,7-tetraoxa-2,4,6,8-tetrasilacyclooxetane). Examples of tetraoxaspiroalkane derivative structures are presented in Fig. 8.
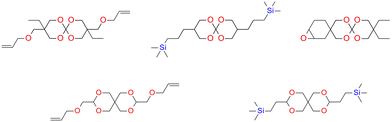 |
| Fig. 8 Structures of oxaspirocyclic monomers. | |
3.3.4. Change the functional groups in monomers to vinylcyclopropanes (VCPs).
Replacement of methacrylates (MAs) with cyclic monomers such as vinylcyclopropanes (VCPs) reduces shrinkage stress.76 The decrease in shrinkage stress for VCP-containing compositions was first observed for 1,1-disubstituted 2-vinylcyclopropanes.77 Both radical-stabilizing and electron-withdrawing groups boost the free-radical ring-opening propensity of very easily accessible 1,1-disubstituted 2-vinylcyclopropanes (Fig. 9).
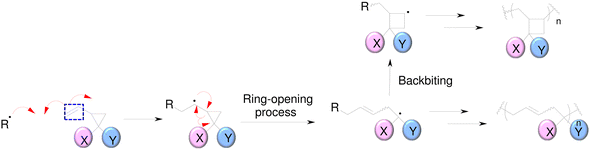 |
| Fig. 9 Radical polymerization of 1,1-disubsituted 2-vinylcyclopropanes (VCPs).78 | |
The high-reactivity substitute structure for a commonly used urethane dimethacrylate (UDMA) that incorporates VCPs was developed by Contreras et al.79 They demonstrated that, VCPs with urethane groups have extremely low shrinkage stress values. While being less reactive than methacrylates, crosslinking vinylcyclopropanes are utilized to promote the network formation of mixes of crosslinking vinylcyclopropanes and dimethacrylates.80
The structures of exemplary vinyl cyclopropanes are shown in Fig. 10.
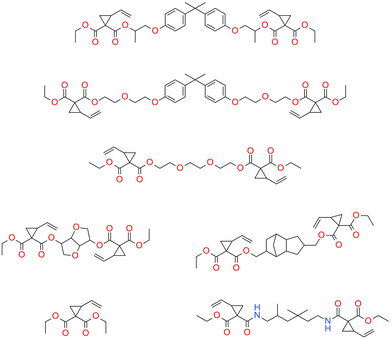 |
| Fig. 10 Examples of vinyl cyclopropanes.78,81 | |
3.3.5. Shifting the polymerization method of methacrylates from conventional free-radical chain-growth polymerization to affect network structure development (AFCT reagents).
Thiol–ene82 or thiol–yne83,84 systems are another tried-and-true method for reducing shrinkage stress brought on by polymerization. Compared to formulations based on free-radical (meth)acrylate photopolymerization; the thiol–ene-based photocurable resins have several benefits. Thiols firstly relieve the oxygen inhibition by acting as strong hydrogen donors to a generated peroxide radical, which in turn produces a reactive thiyl radical.85 Second, composites polymerize via thiol–ene mechanism exhibit lower shrinkage stress than acrylate resins. This is mostly due to the presence of gel point at relatively high conversion (>30%), which is caused by step-growth process of thiol–ene.86 Finally, thiol–ene systems are more biocompatible than (meth)acrylate-based networks.87 Thiol–ene polymerization is a great way to create polymers with specific network structures. While thiol–ene reactions are very efficient, they are restricted in terms of final formulation stability,88 frequently display disagreeable odor,89 and frequently suffer from softness and low Tg due to their thio-ether bridges.90
Since it is known that (meth)acrylate-based photocurable systems result in brittle materials because of their uneven and heavily crosslinked network structure, work has been done to increase homogeneity and, consequently, the toughness of the photocurable dental compositions to comply with the demands of these materials. A (meth)acrylate91 and thiol–ene systems92 can be modified to contain addition–fragmentation chain transfer (AFCT) reagents to control the generation of radical networks (via chain transfer reaction). It is well known that multifunctional monomers (i.e., diacrylates and triacrylates) undergo auto-acceleration in the initial phase of chain growth (free-radical) polymerization due to the fact that termination reactions are limited by mobility. A large kinetic chain length would result in the formation of a network with low homogeneity and high brittleness, which is less effective in stress dissipation, and therefore the shrinkage stress is a higher value.93 Regulation of the final polymer architecture can be achieved using add–fragmentation chain transfer (i.e., AFCT) agents.
The advantage of AFCT functional groups is that they facilitate stress relaxation during the polymerization process. During the process, the bonds are broken and the exact same chemical structure that existed prior to the bond breaking is reconstituted, leading to a rearrangement of the cross-linked strands.92,94,95 For this reason, the approach concentrates on stress dissipation rather than volume shrinkage reduction. The allyl sulphide functional group of AFCT has been incorporated into both thiol–ene53 and thiol-tin96 reactions, yielding significant reductions in polymerization stress.
The reversibility of the AFCT mechanism is only possible for thiol-containing resins, as AFCT involving allyl sulphide requires the presence of thiyl radicals (Fig. 11). Nevertheless, it has also been proven that the introduction of allyl sulphide into methacrylate resins leads to a reduction in stress, but this effect decreases with increasing methacrylate content.97 The presence of a carbon-centred radical in the photopolymerization of methacrylate results to an irreversible AFCT of the allyl sulphide group (Fig. 12).
 |
| Fig. 11 Schematic of the mechanism of allyl sulfide AFCT in the presence of a thiyl radical. The reaction leads to the formation of a reversible symmetric chemical structure. | |
 |
| Fig. 12 Schematic of the mechanism of allyl sulphide AFCT in the presence of a carbon centre radical. The reaction leads to an asymmetric chemical structure, the reaction is irreversible. | |
This AFCT reagent reduces the crosslinking density, favoring a more linear growth of the polymer chain, which shifts the gel point towards higher conversion values and finally reduces the shrinkage stress of the polymerization.98 Therefore, the use of AFCT results in the formation of homogeneous networks and hence provides high toughness networks.99 The implementation of chain transfer via the AFCT mechanism can reduce the shrinkage stress observed in free-radical photopolymerization systems.
The mechanism of addition–fragmentation chain transfer for an ethyl ester activated vinyl sulfone ester (EVS) is presented in Fig. 13.
 |
| Fig. 13 AFCT mechanism for an ethyl ester activated vinyl sulfone ester (EVS). | |
Hamad Algamaiah et al. confirmed that Tetric PowerFill composite (PFill) contains an addition fragmentation chain transfer (AFCT) agent characterized by reduced polymerization shrinkage.100
3.3.6. Shifting the polymerization method of methacrylates from conventional free-radical chain-growth polymerization to affect network structure development (RAFT reagents).
The trithiocarbonate functional group is often utilized as a reversible addition–fragmentation chain transfer (RAFT) agent for the synthesis of polymers with low polydispersity.101 In contrast to the allyl sulphide functional group, the trithiocarbonate functional group is able to completely reversible AFCT when it reacts with a carbon-centred radical, such as that present in the polymerisation of methacrylate (Fig. 14). Park et al. investigated bisphenylglycidyl dimethacrylate/triethylene glycol dimethacrylate BisGMA-TEGDMA-based and BisGMA-TTCDMA (S,S′-bis[α,α’-dimethyl-α′′-(acetyloxy)ethyl 2-methyl-2-propenoate]-trithiocarbonate) containing triocarbonate dental composites to demonstrate stress relaxation via RAFT. The trithiocarbonate functional group was introduced to effectively induce RAFT, leading to network rearrangement. The researchers showed that the incorporation of this mechanism resulted in a 65% stress reduction compared to the standard BisGMA-TEGDMA composite.102
 |
| Fig. 14 Schematic of the RAFT mechanism of a trithiocarbonate in the presence of a carbon centered radical. The reaction leads to a symmetric chemical structure, the reaction is reversible. | |
3.4. Type of photoinitiators
Type of photoinitiator in resins for dental applications have influence on degree of conversion and reaction kinetics which was described in the previous article.39 Furthermore, the type and amount of photoinitiators also affects the value of polymerization shrinkage of dental compositions. To date, the most common resin photoinitiator system in the dental industry has been camphoroquinone (CQ) and co initiators in the form of amine derivatives.103–106 For example, it has been demonstrated that reducing the CQ-amine content to approximately half, results in a reduction in conversion rate, polymerization rate and polymerization shrinkage from 17.6% to 5.5%.107 In other studies, it was reported, commercially available dental fillings with additional photoinitiators such as Lucirin TPO and Ivocerin have the lowest polymerisation shrinkage (e.g. Tetric EvoCeram Bleach BLXL containing Camphorquinone, Lucirin TPO or Tetric Evoceram Powerfill IVB containing Camphorquinone, tertiary amines, Ivocerin, TPO) than dental fillings containing only CQ and tertiary amines (Filtek Ultimate A2).108 Indeed, experimental resins containing 1-phenyl-1,2-propanedione/PPD109 and monoacylphosphine/MAPO109 were proved to induce lower stresses compared to a control system containing only CQ. In contrast, PPD was found to slow down the resin polymerization reaction, allowing greater stress relaxation; whereas MAPO showed a more complex stress reduction mechanism. This mechanism was involved in both delaying the diffusion-controlled polymerization propagation step and increasing the reaction temperature, allowing greater reaction mobility, greater stress relaxation and a delayed onset of vitrification compared to the CQ-based resin.
The summary of the polymerization shrinkage values and their respective reduction for different composition variants is shown in Table 2.
Table 2 Table to present the shrinkage values and relevant reduction of different compositions
Composition/factor leading to lower polymerization shrinkage |
Polymerization shrinkage value |
Ref. |
Standard acrylate monomers |
Polymerization shrinkage of acrylate monomers oscillates at 2–14% |
45
|
Replacement of TEGDMA monomer with allyl carbonate monomer (BPhADAC) |
The reduction in shrinkage from 5.37% to 4.48%. |
135
|
Addition of 9% w/w zinc oxide to standard BisGMA/TEGDMA organic matrix |
The reduction in shrinkage from 15.2% to 8.2% |
136
|
Reducing CQ-amine content to approximately half |
The reduction in shrinkage from 17.6% to 5.5% |
107
|
Silorane monomer |
0.94% |
71
|
4. Conventional and new methods for measuring polymerization shrinkage of dental composites
The numerous of methods for the determination of polymerization shrinkage of dental composite restorative materials have been described in the literature (Table 3). This section presents the main methods of determining the polymerization shrinkage.
Table 3 Summary of methods for measuring polymerization shrinkage
Methods |
Advantage |
Disadvantage |
Dilatometry128 |
• Fast |
• Temperature sensitivity of the mercury in the column |
• Simple |
• Potential health hazards |
Linometery |
• Fast |
• The technique is based on measuring linear shrinkage using contact displacement transducers. |
• Simple |
• There may be potential errors due to gravity or non-uniform shrinkage of the sample |
• Insensitive to temperature changes producing constant results |
|
Gas pschychometry128 |
• Not time-consuming |
• Method showing only the final shrinkage |
• Temperature-independent method |
|
• Helium can occupy voids in a material because helium molecules are much smaller than water. |
|
Strain gauge110 |
• Very sensitive to linear dimensional changes |
• Difficulty in placing and holding bulk material samples of sufficient thickness on the strain gauge. |
Archimedes principle (buoyancy method)124 |
• It is the only method for measuring polymerization shrinkage that has published performance standards in ISO 17304 |
• Multi-step, time-consuming and requires the consideration of a number of variables e.g. the presence of voids inside the specimen or air bubbles on the surface of the specimen |
The bonded-disk method110 |
• Simple design |
• Can only be used for relatively thin samples |
• Does not require expensive instrumentation |
|
• The thickness of the samples is small and, therefore, light easily penetrates the material. This ensures equal conversion of the monomer on the top and bottom surfaces and consequently over the entire thickness |
|
Optical coherence tomography (OCT) |
• Non-invasive and non-contact medical diagnostic imaging modality |
• Expensive |
• Produces both high-resolution images of teeth and periodontal tissues without exposing the patient to ionising radiation |
|
X-ray microcomputed tomography |
• High-resolution X-ray μCT allows 3D information |
• Expensive apparatus |
AcuVol™ |
• Easy-to-use device for tracking polymerization shrinkage throughout the curing process |
• Expensive apparatus |
• Accurate measurements of polymerization shrinkage |
|
Digital image correlation (DIC) |
• Full-field sample measurement |
• Expensive apparatus |
• Providing more detailed and useful information on the shrinkage |
|
4.1. Dilatometry
Dilatometry is the most widely applied method for determining polymerization shrinkage. In this method, a non-reactive liquid, such as mercury, surrounds the composite sample during curing. Monitoring the level of mercury in the capillary allows the operator to measure the volume shrinkage (VS) associated with the polymerization of the sample. Thus, the amount of shrinkage is recorded, given that the shrinkage is monitored during the curing period from pre-gel to post-gel phase.110 The disadvantage of the dilatometers is that they are extremely sensitive to variations in temperature.111,112
A recording dilatometer for measuring polymerization shrinkage was described as early as the second half of the 20th century and is still employed today.113,114 For instance, Wang et al. in 2018 measured the polymerization shrinkage of dental resin composites with hydrolytically stable monomers consisting of UDMA and hydrolytically stable triethylene glycol-divinylbenzyl ether TEG-DVBE using a mercury dilatometer (ADA Foundation, Gaithersburg, MD, USA).113 The measurement consisted of placing approximately 0.1 g of the composite on a sandblasted and silanised glass slide. The glass column was clamped onto the slide and filled with mercury. After this, an LVDT (linear variable differential transducer) probe was placed on the slide. The composite was light-cured through the glass slide.115 The modified mercury dilatometer has also been proposed, in which each change in sample volume is recorded every 0.5 s as a change in the height of the mercury in the capillary, which is measured electronically instead of with the naked eye. The device is relatively inexpensive and allows measurements to be made on samples regardless of shape and size.111
4.2. Linometery
In 1969, Lee et al. from California determined linear polymerization shrinkage using a dilatometer to the nearest 0.00005 inch on a cylinder. The measurement consisted of placing a portion of the mixture into a glass tube 6.35 mm in diameter and approximately 9.5 mm long. The plunger was then pressed into the mixed material and the dilatometer was zeroed within 45 seconds of mixing. The sample was left to cure at room temperature for 18 h. The percentage of shrinkage was calculated from:
where: ΔL – change in length, L – cured length.
The average of five readings was taken for each material.116
A few years later, 1993, de Gee et al. introduced a modified linometer that was quite simple, fast and insensitive to temperature changes, giving constant results. They found no significant differences between this method and dilatometry. A composite sample was placed between a glass and an aluminum disc. The distance from the aluminum disc to the glass could be adjusted using a displacement transducer to select the height of the resin sample. The disc and glass were lubricated to avoid adhesion of the composite sample. However, this apparatus only measured linear changes.117 It is worth noting that there are no significant differences between this method and dilatometry.
Interestingly, the method was described by Shah et al. in 2010 for measuring linear shrinkage measurements using a rheometer.118 Nejadebrahim in 2019 determined polymerization shrinkage with a new three-component safranin-based photoinitiating system using an Anton Paar MCR 302 rheometer, which was combined with accessories for light-curing samples. The test was carried out in the oscillatory mode of the rheometer. The smooth aluminum plate was fixated at an initial gap of 0.5 mm and a zero Newton normal force was applied to the samples. An optical fibre was placed perpendicularly under the glass plate to allow the curing process to take place. With polymerization shrinkage, the gap was decreased. The polymerization shrinkage was calculated from the formula:119
where:
dfinal – distances between the glass and aluminum plate at the beginning of photopolymerization,
dinitial – distances between the glass and aluminum plate at the beginning of photopolymerization.
The experimental setup is presented in Fig. 15.
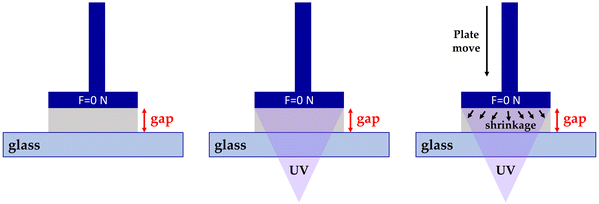 |
| Fig. 15 Schematic representation of the shrinkage measurement apparatus. | |
Polymerization shrinkage is also common in photo-cured 3D printing, due to the fact that the base material is also acrylate monomers are characterized by high polymerization shrinkage. Therefore, polymerization shrinkage tests using a rheometer are becoming increasingly popular and are also used to measure the polymerization shrinkage of objects used in 3D printing.120
4.3. Gas pschychometry
In 1999, Cook et al. used a non-contact method called a displacement pycnometer to determine the dry volume changes of composite materials during the polymerization process. The disadvantage of this method is that it only measures the final shrinkage. Nevertheless, the advantage of this method is that it is not time-consuming.
The standard measurement is that a sample of the composite paste is placed between two small sheets of thin Mylar sheet. The volume of the sample including the Mylar sheets is then determined at constant room temperature (23 °C ± 2 °C) using a controlled helium tank gas pycnometer.
The volume of the sample placed in the chamber is determined by varying the helium pressure. Then, after curing, the volume of the composite sample and Mylar sheets is determined again, and the difference between the uncured and cured samples (ΔV) is calculated. The Mylar sheets are then removed from the sample and the volume (V) of the cured sample itself is determined. The quantification of the percentage shrinkage (S) is determined using the formula:121
ΔV – difference between the uncured and cured samples, V – volume of the cured sample.
4.4. Strain gauge
Strain gauge are very sensitive to linear dimensional changes. Here, the gauge is connected to the substrate and the linear dimensional changes occurring in the substrate are transferred to the gauge and measured. This method can be applied to measure the post-gel shrinkage of composites. This is because the substrate has a measurable modulus, to induce stress on the gauge, the linear dimensional changes will be transferred to the gauge.122
4.5. Archimedes principle
Archimedes’ principle (buoyancy of a material in a liquid) is a simple and inexpensive method that has been used to determine the volume change of a sample by measuring density changes.123 The method involves weighing materials several times in two environments of known density: one is conventional air, the other can be distilled water, siliconized oil, mercury and sodium lauryl sulphate of at least 99% purity.124
ρ – density of the materials, mwater – mass of sample in water (g), mair – mass of sample in air (g), ρwater – density of water at a precisely measured temperature, ρair – density of the air (air density: 0.0012 g cm−1).124
In turn, the change in volume is calculated:
In this method, sample size and geometry are not considered a problem when applying Archimedes’ principles. The process is multi-step, time-consuming and requires the consideration of a number of variables e.g. the presence of voids inside the specimen or air bubbles on the surface of the specimen, which may affect the test results. Nevertheless, it is the only method for measuring polymerization shrinkage that has published performance standards in ISO 17304.124 Therefore, this method is popular for determining the polymerization shrinkage of dental composites.44,125,126 For example, Zhao et al. studied the polymerization shrinkage by this method of hybrid composites used for 3D printing of tooth crowns.127
4.6. The bonded-disk method
The method was developed in 1991 by Watts and Cash.128 The method involves placing a disc of resin composite in a brass ring (16 mm × 1.5 mm), which is bonded to a glass slide. The composite is covered with a microscope coverslip (approximately 0.1 mm thick). The composite sample is cured from below by the glass slide. As the composite shrinks, it pulls the coverslip downwards and its deflection is monitored by the linear vertical displacement transducer (LVDT) probe attached to the middle of the coverslip. Shrinkage is calculated by dividing the measured deflection of the coverslip by the initial height of the composite. Because the composite sample must exhibit some stiffness to flex the slide, this method is likely to measure shrinkage after gelling.128
4.7. Optical coherence tomography (OCT)
OCT is a non-invasive and non-contact medical diagnostic imaging modality. The principle of OCT is analogous to methods such as CT, MRI and B-scan ultrasonography, which use X-rays, spin resonance and sound waves respectively, except that only light is used.
OCT is based on a Michelson interferometer with a low-coherence and broadband light source. The apparatus consists of a scanning probe, a base unit containing a superluminescent diode as light source and a computer. In this method, 3 scans are performed: first, a scan is performed on a hollow cylindrical mould made of Teflon, then the composite is introduced and a 2nd scan is performed, followed by a 3rd scan after 15 minutes of photo-curing. The linear shrinkage is calculated from the formula:
RC0 min – thickness of the composition between 1 and 3 points of unpolymerized state, RC15 min – thickness of the composition between 1 and 3 of the polymerized state.
The OCT technique produces both high-resolution images of teeth and periodontal tissues without exposing the patient to ionising radiation.44
4.8. X-ray microcomputed tomography (μCT)
X-ray microcomputed tomography (μCT) is used to study the 3D marginal adaptation of light-cured composite resin restorations and the tooth-composite interface. In addition, high-resolution X-ray μCT allows 3D information to be obtained from the cavity during the polymerization process. This method for determining polymerization shrinkage was used by Rizzante et al. In the same work, he showed a strong correlation between filler content and volumetric shrinkage, the higher the inorganic filler content, the lower the polymerization shrinkage.129 Sampaio et al. have shown that both μCT and OCT techniques can be used to detect pulp floor fissures in restorations.48
4.9. AcuVol™
The AcuVol instrument from Biso applies the video imaging method and allows accurate measurements of polymerization shrinkage. This device includes a table-top instrument that can be connected to a computer. This method enables comparison of the volume of composites before curing (before polymerization shrinkage) and after curing (after polymerization shrinkage). In addition, it is an easy-to-use device for tracking polymerization shrinkage throughout the curing process.130
Dano et al. investigated the polymerization shrinkage of oxirane/acrylate interpenetrating polymer network (IPN) composite resins using the AcuVol™ video imaging technique. Low volumes of samples (∼15 μl) of the composite material were placed in the AcuVol and cured from above. During the measurement, images are generated that simultaneously show the sample before and after curing. It registers the relative change in volume (shrinkage). In addition, a titanium dioxide pigment (0.01 wt%) was introduced into the monomers to ensure accurate identification of the droplet outline by the imaging software.131 Ender Akan studied the effect of the shade of adhesive resin cement on its polymerization shrinkage using the AcuVol technique. He demonstrated that different shades showed different volumetric shrinkage. Group 5 (opaque yellow, 2.62%) and group 2 (universal, brown, 3.96%) showed the lowest and highest percentage of shrinkage, respectively.127 Using this device, Canellas et al. determined the polymerization shrinkage of dental composites containing methacrylethylpolyhedral oligomeric silsesquioxane (ME-POSS) and showed that the addition of this compound reduces the polymerization shrinkage of composites.134
4.10. Digital image correlation (DIC)
Digital image correlation is a non-contact method that was developed in the 1980s at the University of South Carolina. The idea of the method is to visualize patterns on the surface of the sample from sequential images taken during the deformation of the material in a full-field measurement, which allows displacement and deformation to be determined by tracing movements on the surface of the sample. The full-field measurement capability enables accurate analysis of inhomogeneous deformations and stresses.130,132 This allows more accurate decisions to be made regarding the choice of appropriate materials and embedding techniques for the restoration of damaged or decayed teeth.
4.11. Future insights
Major progress has been achieved in reducing polymerization shrinkage in dental composites. However, considering the complexity of the problem due to the dependence of polymerization shrinkage on numerous factors such as the type of monomer, initiators, curing methods and curing time, the researchers are still looking for a dental material characterized not only by a low polymerization shrinkage of less than 1%, but also a lack of toxicity, complete reactivity of the monomer to the polymer, good mechanical properties and a cheap. Therefore, the issue of producing dental composites with low polymerization shrinkage is still a topic that is being addressed by researchers from different areas of the world.
5. Conclusion
This paper presents the influence of the choice of photopolymerization process conditions and also the composition of the composition on the polymerization shrinkage of dental composites. In particular, it was demonstrated that the polymerization shrinkage of dental composites depends on the type of monomers, photoinitiators, the type and amount of inorganic fillers and the curing method. This paper presents alterative curing mechanisms, alternative monomers, photoinitiators, and inorganic fillers and curing method to minimize undesirable polymerization shrinkage in dental composites. The second section demonstrates standard and novel methods for testing the polymerization shrinkage. It is shown that each method has its own advantages and disadvantages. In addition, due to the diversity of apparatus performance, polymerization shrinkage values should be assessed using the same analytical method. It is also necessary to have a standardized apparatus to compare polymerization shrinkage for different dental fillings. In addition, researchers are still looking for a dental material characterized by low polymerization shrinkage but also a lack of toxicity, complete reactivity of the monomer to the polymer, good mechanical properties and a low cost.
Conflicts of interest
The authors declare no conflict of interest.
Acknowledgements
The work was funded by the National Science Centre (NCN, Poland), PRELUDIUM 17, project no: 2019/33/N/ST5/03015.
References
- J. V. Crivello, J. Macromol. Sci., Part A: Pure Appl. Chem., 2015, 52, 336–344 CrossRef CAS.
- V. A. Bobrin, Y. Yao, X. Shi, Y. Xiu, J. Zhang, N. Corrigan and C. Boyer, Nat. Commun., 2022, 13, 3577 CrossRef CAS PubMed.
- J. Ortyl, M. Galica, R. Popielarz and D. Bogdał, Pol. J. Chem. Technol., 2014, 16, 75–80 CrossRef CAS.
- E. Hola, M. Topa, A. Chachaj-Brekiesz, M. Pilch, P. Fiedor, M. Galek and J. Ortyl, RSC Adv., 2020, 10, 7509–7522 RSC.
- M. Topa, E. Hola, M. Galek, F. Petko, M. Pilch, R. Popielarz, F. B. G. Morlet-Savary, J. Laleveé and J. Ortyl, Polym. Chem., 2020, 11, 5261–5278 RSC.
- Z. Liu and F. Dumur, Eur. Polym. J., 2022, 177, 111449 CrossRef CAS.
- A. Al Mousawi, C. Dietlin, B. Graff, F. Morlet-Savary, J. Toufaily, T. Hamieh, J. P. Fouassier, A. Chachaj-Brekiesz, J. Ortyl and J. Lalevée, Macromol. Chem. Phys., 2016, 217, 1955–1965 CrossRef.
- M. Topa, F. Petko, M. Galek, M. Jankowska, R. Popielarz and J. Ortyl, Eur. Polym. J., 2021, 156, 110612 CrossRef CAS.
- H. C. Kiliclar, G. Yilmaz, Z. Li, Y. Zheng and Y. Yagci, Macromol. Chem. Phys., 2023, 224, 2200343 CrossRef CAS.
- M. Topa, F. Petko, M. Galek and J. Ortyl, Sensors, 2020, 20, 3043 CrossRef CAS PubMed.
- J. Shao, Y. Huang and Q. Fan, Polym. Chem., 2014, 5, 4195–4210 RSC.
- W. Tomal, M. Pilch, A. Chachaj-Brekiesz, M. Galek, F. Morlet-Savary, B. Graff, C. Dietlin, J. Lalevée and J. Ortyl, Polym. Chem., 2020, 11, 4604–4621 RSC.
- E. Hola, M. Pilch, M. Galek and J. Ortyl, Polym. Chem., 2020, 11, 480–495 RSC.
- M. Bouzrati-Zerelli, J. Kirschner, C. P. Fik, M. Maier, C. Dietlin, F. Morlet-Savary, J. P. Fouassier, J. M. Becht, J. E. Klee and J. Lalevée, Macromolecules, 2017, 50, 6911–6923 CrossRef CAS.
- J. Ortyl, K. Sawicz and R. Popielarz, J. Polym. Sci., Part A: Polym. Chem., 2010, 48, 4522–4528 CrossRef CAS.
- J. Ortyl, J. Wilamowski, P. Milart, M. Galek and R. Popielarz, Polym. Test., 2015, 48, 151–159 CrossRef CAS.
- J. Ortyl, M. Galek, P. Milart and R. Popielarz, Polym. Test., 2012, 31, 466–473 CrossRef CAS.
- N. Moszner, Macromol. Symp., 2004, 217, 63–76 CrossRef CAS.
- K. Yoshinaga, K. Yoshihara and Y. Yoshida, Dent. Mater., 2021, 37, e391–e398 CrossRef CAS PubMed.
- A. Bagheri and J. Jin, ACS Appl. Polym. Mater., 2019, 1, 593–611 CrossRef CAS.
- A. Jandyal, I. Chaturvedi, I. Wazir, A. Raina, M. Irfan and U. Haq, Sustainable Oper. Comput., 2022, 3, 33–42 CrossRef.
- A. Kowalska, J. Sokolowski and K. Bociong, Polymers, 2021, 13, 1–17 Search PubMed.
- M. Topa and J. Ortyl, J. Photopolym. Sci. Technol., 2021, 34, 259–262 CrossRef CAS.
- M. V. Salvador, B. M. Fronza, V. G. A. Pecorari, F. A. Ogliari, R. R. Braga, J. D. Oxman and A. F. Lima, Dent. Mater., 2021, 37, 1358–1365 CrossRef CAS PubMed.
- A. A. Pérez-Mondragón, C. E. Cuevas-Suárez, J. A. González-López, N. Trejo-Carbajal and A. M. Herrera-González, J. Photochem. Photobiol., A, 2020, 403, 112844 CrossRef.
- M. Topa and J. Ortyl, Materials, 2020, 13, 4093 CrossRef CAS PubMed.
- J. He, S. Garoushi, E. Säilynoja, P. K. Vallittu and L. Lassila, Dent. Mater., 2019, 35, 627–635 CrossRef CAS PubMed.
- Y. R. Riva and S. F. Rahman, AIP Conf. Proc., 2019, 2193, 020011 CrossRef.
- J. A. González-López, A. A. Pérez-Mondragón, C. E. Cuevas-Suárez, S. C. Esparza González and A. M. Herrera-González, J. Mech. Behav. Biomed. Mater., 2020, 110, 103955 CrossRef PubMed.
- M. L. Berlanga Duarte, L. A. Reyna Medina, P. Torres Reyes, S. C. Esparza González and A. M. Herrera González, J. Appl. Polym. Sci., 2019, 136, 1–10 CrossRef.
- Y. Catel, J. Angermann, P. Fässler, U. Fischer, T. Schnur and N. Moszner, Dent. Mater., 2021, 37, 351–358 CrossRef CAS PubMed.
- J. Wu, X. Xie, H. Zhou, F. R. Tay, M. D. Weir, M. A. S. Melo, T. W. Oates, N. Zhang, Q. Zhang and H. H. K. Xu, Polym. Degrad. Stab., 2019, 163, 87–99 CrossRef CAS.
- L. Song, Q. Ye, X. Ge, A. Misra, C. Tamerler and P. Spencer, Acta Biomater., 2019, 83, 130–139 CrossRef CAS PubMed.
- S. Venz and B. Dickens, J. Biomed. Mater. Res., 1991, 25, 1231–1248 CrossRef CAS PubMed.
- Y. Kinomoto, M. Torii, F. Takeshige and S. Ebisu, J. Dent., 1999, 27, 383–389 CrossRef CAS PubMed.
- J. W. Park and J. L. Ferracane, Dent. Mater., 2005, 21, 882–889 CrossRef CAS PubMed.
- J. W. Park and J. L. Ferracane, J. Dent. Res., 2006, 85, 945–949 CrossRef CAS PubMed.
- A. J. Feilzer, A. J. de Gee and C. L. Davidson, J. Dent. Res., 1987, 66, 1636–1639 CrossRef CAS PubMed.
- R. R. Braga, R. Y. Ballester and J. L. Ferracane, Dent. Mater., 2005, 21, 962–970 CrossRef CAS PubMed.
- T. Dikova, J. Maximov, V. Todorov, G. Georgiev and V. Panov, Processes, 2021, 9, 779 CrossRef CAS.
- J. W. Stansbury, J. Esthetic Restor. Dent., 2000, 12, 300–308 CrossRef CAS PubMed.
- M. Daronch, F. A. Rueggeberg, M. F. De Goes and R. Giudici, J. Dent. Res., 2006, 85, 38–43 CrossRef CAS PubMed.
- A. Versluis, W. H. Douglas, M. Cross and R. L. Sakaguchi, J. Dent. Res., 1996, 75, 871–878 CrossRef CAS PubMed.
- P. Ausiello, A. M. d. O. Dal Piva, A. L. S. Borges, A. Lanzotti, F. Zamparini, E. Epifania and J. P. M. Tribst, Appl. Sci., 2021, 11, 1–12 Search PubMed.
- R. B. Price, A. S. Rizkalla and G. C. Hall, Am. J. Dent., 2000, 13, 176–180 CAS.
- N. Ivković, D. Božović, S. Ristić, V. Mirjanić and O. Janković, Contemp. Mater., 2013, 4, 84–91 Search PubMed.
- K. Aalto-Korte, K. Alanko, O. Kuuliala and R. Jolanki, Contact Dermatitis, 2007, 57, 324–330 CrossRef CAS PubMed.
- C. S. Sampaio, J. Fernández Arias, P. J. Atria, E. Cáceres, C. Pardo Díaz, A. Z. Freitas and R. Hirata, Dent. Mater., 2019, 35, 1568–1575 CrossRef CAS PubMed.
- J. L. Ferracane, Oper. Dent., 2008, 33, 247–257 CrossRef PubMed.
- J. L. Ferracane, Dent. Mater., 2005, 21, 36–42 CrossRef CAS PubMed.
- R. Labella, P. Lambrechts, B. Van Meerbeek and G. Vanherle, Dent. Mater., 1999, 15, 128–137 CrossRef CAS PubMed.
- C. J. Kleverlaan and A. J. Feilzer, Dent. Mater., 2005, 21, 1150–1157 CrossRef CAS PubMed.
- Z. Tarle, T. Attin, D. Marovic, L. Andermatt, M. Ristic and T. T. Tauböck, Clin. Oral Invest., 2015, 19, 831–840 CrossRef CAS PubMed.
- H. Al Sunbul, N. Silikas and D. C. Watts, Dent. Mater., 2016, 32, 998–1006 CrossRef CAS PubMed.
- I. Aljosa, L. Tijana, B. Larisa and V. Marko, Procedia Eng., 2014, 69, 921–930 CrossRef CAS.
- R. C. B. Alonso, W. C. Brandt, E. J. C. Souza-Junior, R. M. Puppin-Rontani and M. A. C. Sinhoreti, Appl. Adhes. Sci., 2014, 2, 1–11 CrossRef.
- C. A. K. Shimokawa, P. M. A. Carneiro, T. R. da S. Lobo, V. E. Arana-Chavez, M. N. Youssef and M. L. Turbino, Int. Dent. J., 2016, 66, 257–263 CrossRef PubMed.
- Z. Wang, F. A. Landis, A. A. M. Giuseppetti, S. Lin-Gibson and M. Y. M. Chiang, Dent. Mater., 2014, 30, 1316–1324 CrossRef CAS PubMed.
- J. Han, S. Jiang, Y. Gao and F. Sun, J. Mater. Chem. C, 2016, 4, 10675–10683 RSC.
- R. R. Moraes, J. W. Garcia, M. D. Barros, S. H. Lewis, C. S. Pfeifer, J. Liu and J. W. Stansbury, Dent. Mater., 2011, 27, 509–519 CrossRef CAS PubMed.
- V. Greig, Br. Dent. J., 2012, 213, 90–90 CrossRef.
- M. H. Chen, J. Dent. Res., 2010, 89, 549–560 CrossRef CAS PubMed.
- S. B. Burujeny, H. Yeganeh, M. Atai, H. Gholami and M. Sorayya, Dent. Mater., 2017, 33, 119–131 CrossRef CAS PubMed.
- Z. Wang and M. Y. M. Chiang, Dent. Mater., 2016, 32, 551–560 CrossRef CAS PubMed.
- K. S. Gregson, J. Terrence O'Neill, J. A. Platt and L. Jack Windsor, Dent. Mater., 2008, 24, 1461–1467 CrossRef CAS PubMed.
-
Sato et al., JP Pat., 51033541, 1976 Search PubMed.
- J. V. Crivello, Polym. Eng. Sci., 1992, 32, 1462–1465 CrossRef CAS.
- J. V. Crivello and J. L. Lee, J. Polym. Sci., Part A: Polym. Chem., 1990, 28, 479–503 CrossRef CAS.
- P. H. Chung, J. V. Crivello and M. Fan, J. Polym. Sci., Part A: Polym. Chem., 1993, 31, 1741–1746 CrossRef CAS.
- J. D. Eick, S. P. Kotha, C. C. Chappelow, K. V. Kilway, G. J. Giese, A. G. Glaros and C. S. Pinzino, Dent. Mater., 2007, 23, 1011–1017 CrossRef CAS PubMed.
- N. Hamano, Y. C. Chiang, I. Nyamaa, H. Yamaguchi, S. Ino, R. Hickel and K. H. Kunzelmann, Dent. Mater., 2012, 28, 894–902 CrossRef CAS PubMed.
- G. Marchesi, L. Breschi, F. Antoniolli, R. Di Lenarda, J. Ferracane and M. Cadenaro, Dent. Mater., 2010, 26, 947–953 CrossRef CAS PubMed.
- A. R. Aleixo, R. D. Guiraldo, A. P. P. Fugolin, S. B. Berger, R. L. X. Consani, A. B. Correr, A. Gonini-Júnior and M. B. Lopes, Photomed. Laser Surg., 2014, 32, 267–273 CrossRef CAS PubMed.
-
(a) W. J. Bailey and T. Endo, J. polym. sci., Polym. symp., 2007, 64, 17–26 CrossRef;
(b) W. J. Bailey and R. L. Sun, Polym. Prepr. (Am. Chem. Soc., Div. Polym. Chem.), 1972, 13, 281 CAS;
(c) T. Endo and W. J. Bailey, J. Polym. Sci. Polym. Lett. Ed., 1980, 18, 25 CrossRef CAS.
-
C. Chappelow, C. Pinzino, S-S. Chen and J. D. Eick, US20070072954A1, 2006.
- N. Moszner and U. Salz, Macromol. Mater. Eng., 2007, 292, 245–271 CrossRef CAS.
- F. Sanda, T. Takata and T. Endo, Macromolecules, 1993, 26, 1818–1824 CrossRef CAS.
- S. Tauscher, Y. Catel, P. Fässler, U. Fischer and N. Moszner, J. Appl. Polym. Sci., 2017, 134, 1–9 CrossRef.
- P. Pineda Contreras, C. Kuttner, A. Fery, U. Stahlschmidt, V. Jérôme, R. Freitag and S. Agarwal, Chem. Commun., 2015, 51, 11899–11902 RSC.
- N. Moszner, T. Völkel, U. K. Fischer and V. Rheinberger, Macromol. Rapid Commun., 1999, 20, 33–35 CrossRef.
- S. Schoerpf, Y. Catel, N. Moszner, C. Gorsche and R. Liska, Polym. Chem., 2019, 10, 1357–1366 RSC.
- C. E. Hoyle and C. N. Bowman, Angew. Chem., Int. Ed., 2010, 49, 1540–1573 CrossRef CAS PubMed.
- R. Hoogenboom, Angew. Chem., Int. Ed., 2010, 49, 3415–3417 CrossRef CAS PubMed.
- A. Oesterreicher, C. Gorsche, S. Ayalur-Karunakaran, A. Moser, M. Edler, G. Pinter, S. Schlögl, R. Liska and T. Griesser, Macromol. Rapid Commun., 2016, 37, 1701–1706 CrossRef CAS PubMed.
- B. Husár, S. C. Ligon, H. Wutzel, H. Hoffmann and R. Liska, Prog. Org. Coat., 2014, 77, 1789–1798 CrossRef.
- O. D. McNair, A. P. Janisse, D. E. Krzeminski, D. E. Brent, T. E. Gould, J. W. Rawlins and D. A. Savin, ACS Appl. Mater. Interfaces, 2013, 5, 11004–11013 CrossRef CAS PubMed.
- A. Oesterreicher, J. Wiener, M. Roth, A. Moser, R. Gmeiner, M. Edler, G. Pinter and T. Griesser, Polym. Chem., 2016, 7, 5169–5180 RSC.
- P. Esfandiari, S. C. Ligon, J. J. Lagref, R. Frantz, Z. Cherkaoui and R. Liska, J. Polym. Sci., Part A: Polym. Chem., 2013, 51, 4261–4266 CrossRef CAS.
- M. Podgórski, E. Becka, M. Claudino, A. Flores, P. K. Shah, J. W. Stansbury and C. N. Bowman, Dent. Mater., 2015, 31, 1263–1270 CrossRef PubMed.
- M. Podgórski, C. Wang, Y. Yuan, D. Konetski, I. Smalyukh and C. N. Bowman, Chem. Mater., 2016, 28, 5102–5109 CrossRef.
- C. Gorsche, K. Seidler, P. Knaack, P. Dorfinger, T. Koch, J. Stampfl, N. Moszner and R. Liska, Polym. Chem., 2016, 7, 2009–2014 RSC.
- T. F. Scott, A. D. Schneider, W. D. Cook and C. N. Bowman, Science, 2005, 308, 1615–1617 CrossRef CAS PubMed.
- S. C. Ligon-Auer, M. Schwentenwein, C. Gorsche, J. Stampfl and R. Liska, Polym. Chem., 2016, 7, 257–286 RSC.
- J. C. Kloxin, F. T. Scott and N. C. Bowman, Macromolecules, 2009, 42, 2551–2556 CrossRef PubMed.
- T. F. Scott, R. B. Draughon and C. N. Bowman, Adv. Mater., 2006, 18, 2128–2132 CrossRef CAS.
- H. Y. Park, C. J. Kloxin, T. F. Scott and C. N. Bowman, Macromolecules, 2010, 43, 10188–10190 CrossRef CAS PubMed.
- H. Y. Park, C. J. Kloxin, T. F. Scott and C. N. Bowman, Dent. Mater., 2010, 26, 1010–1016 CrossRef CAS PubMed.
- C. Gorsche, T. Koch, N. Moszner and R. Liska, Polym. Chem., 2015, 6, 2038–2047 RSC.
- C. Gorsche, M. Griesser, G. Gescheidt, N. Moszner and R. Liska, Macromolecules, 2014, 47, 7327–7336 CrossRef CAS.
- H. Algamaiah, N. Silikas and D. C. Watts, Dent. Mater., 2021, 37, 559–567 CrossRef CAS PubMed.
- T. Roshan, E. Rizzardo, J. Chiefari, J. Krstina, G. Moad, A. Postma and S. H. Thang, Macromolecules, 2000, 33, 243–245 CrossRef.
- H. Y. Park, C. J. Kloxin, M. F. Fordney and C. N. Bowman, Dent. Mater., 2012, 28, 888–893 CrossRef CAS PubMed.
- M. Oguri, Y. Yoshida, K. Yoshihara, T. Miyauchi, Y. Nakamura, S. Shimoda, M. Hanabusa, Y. Momoi and B. Van Meerbeek, Acta Biomater., 2012, 8, 1928–1934 CrossRef CAS PubMed.
- F. Morlet-Savary, J. E. Klee, F. Pfefferkorn, J. P. Fouassier and J. Lalevée, Macromol. Chem. Phys., 2015, 216, 2161–2170 CrossRef CAS.
- N. Ilie and R. Hickel, Dent. Mater. J., 2008, 27, 221–228 CrossRef PubMed.
- L. Roedel, V. Bednarzig, R. Belli, A. Petschelt, U. Lohbauer and J. Zorzin, Clin. Oral Invest., 2017, 21, 1735–1741 CrossRef PubMed.
- C. S. Pfeifer, J. L. Ferracane, R. L. Sakaguchi and R. R. Braga, Am. J. Dent., 2009, 22, 206–2010 Search PubMed.
- A. Kowalska, J. Sokolowski, T. Gozdek, M. Krasowski, K. Kopacz and K. Bociong, Polymers, 2021, 13, 3972 CrossRef CAS PubMed.
- W. M. Palin, M. A. Hadis, J. G. Leprince, G. Leloup, L. Boland, G. J. P. Fleming, G. Krastl and D. C. Watts, Dent. Mater., 2014, 30, 507–516 CrossRef CAS PubMed.
- R. L. Sakaguchi, B. D. Wiltbank and N. C. Shah, Dent. Mater., 2004, 20, 388–396 CrossRef CAS PubMed.
- T. G. Oberholzer, S. R. Grobler, C. H. Pameijer and R. J. Rossouw, Meas. Sci. Technol., 2002, 13, 78–83 CrossRef CAS.
- R. Mulder, S. R. Grobler and Y. I. Osman, Oral Biol. Dent., 2013, 1, 1 CrossRef.
- X. Wang, G. Huyang, S. V. Palagummi, X. Liu, D. Skrtic, C. Beauchamp, R. Bowen and J. Sun, Dent. Mater., 2018, 34, 228–237 CrossRef CAS PubMed.
- A. J. de Gee, C. L. Davidson and A. Smith, J. Dent., 1981, 9, 36–42 CrossRef CAS PubMed.
- X. Wang, G. Huyang, S. V. Palagummi, X. Liu, D. Skrtic, C. Beauchamp, R. Bowen and J. Sun, Dent. Mater., 2018, 34, 228–237 CrossRef CAS PubMed.
- H. L. Lee, M. L. Swartz and F. F. Smith, J. Dent. Res., 1969, 48, 526–535 CrossRef CAS PubMed.
- A. J. de Gee, A. J. Feilzer and C. L. Davidson, Dent. Mater., 1993, 9, 11–14 CrossRef CAS PubMed.
- D. U. Shah and P. J. Schubel, Polym. Test., 2010, 29, 629–639 CrossRef CAS.
- A. Nejadebrahim, M. Ebrahimi, X. Allonas, C. Croutxé-Barghorn, C. Ley and B. Métral, RSC Adv., 2019, 9, 39709–39720 RSC.
- M. Topa-Skwarczyńska, A. Świeży, D. Krok, K. Starzak, P. Niezgoda, B. Oksiuta, W. Wałczyk and J. Ortyl, Int. J. Mol. Sci., 2022, 23, 10470 CrossRef PubMed.
- W. D. Cook, M. Forrest and A. A. Goodwin, Dent. Mater., 1999, 15, 447–449 CrossRef CAS PubMed.
- R. L. Sakaguchi, C. T. Sasik, M. A. Bunczak and W. H. Douglas, J. Dent., 1991, 19, 312–316 CrossRef CAS PubMed.
- G. Q. D. M. Monteiro, M. A. J. R. Montes, T. V. Rolim, C. C. B. de Oliveira Mota, B. D. B. C. Kyotoku, A. S. L. Gomes and A. Z. De Freitas, Dent. Mater., 2011, 27, e176–e185 CrossRef CAS PubMed.
- ISO – ISO 17304:2013 – Dentistry—Polymerization shrinkage: Method for determination of polymerization shrinkage of polymer-based restorative materials, https://www.iso.org/standard/59772.html, (accessed 25 January 2023).
- S. Luo, F. Liu and J. He, J. Mech. Behav. Biomed. Mater., 2019, 94, 222–228 CrossRef CAS PubMed.
- J. He, S. Garoushi, P. K. Vallittu and L. Lassila, Acta Biomater. Odontol. Scand., 2018, 4, 30–37 CrossRef CAS PubMed.
- M. Zhao, Y. Geng, S. Fan, X. Yao, M. Zhu and Y. Zhang, Compos. Sci. Technol., 2021, 213, 108902 CrossRef CAS.
- D. C. Watts and A. J. Cash, A. J. Dent. Mater., 1991, 7, 281–287 CrossRef CAS PubMed.
- F. A. P. Rizzante, J. A. Duque, M. A. H. Duarte, R. F. L. Mondelli, G. Mendonça and S. K. Ishikiriama, Dent. Mater. J., 2019, 38, 403–410 CrossRef CAS PubMed.
- J. Y. Li, A. Lau and A. S. L. Fok, J. Zhejiang Univ., Sci., A, 2013, 14, 1–10 CrossRef CAS.
- R. Danso, B. Hoedebecke, K. Whang, S. Sarrami, A. Johnston, S. Flipse, N. Wong and H. R. Rawls, Dent. Mater., 2018, 34, 1459–1465 CrossRef CAS PubMed.
- J. Li, P. Thakur and A. S. L. Fok, J. Visualized Exp., 2014, 1–9 Search PubMed.
- S. Luo, F. Liu, B. Yu and J. He, J. Biomater. Sci. Polym. Ed., 2019, 30, 137–149 CrossRef CAS PubMed.
- T. A. T. Canellas, A. de Almeida Neves, I. K. dos Santos, A. R. P. de Rezende, C. E. Fellows and E. M. da Silva, J. Mech. Behav. Biomed. Mater., 2019, 90, 566–574 CrossRef CAS PubMed.
- C. E. Cuevas-Suárez, J. A. González-López, A. F. da Silva, E. Piva and A. M. Herrera-González, J. Mech. Behav. Biomed. Mater., 2018, 87, 148–154 CrossRef PubMed.
- B. Pratap, R. K. Gupta, B. Bhardwaj and M. Nag, Mater. Today: Proc., 2020, 33, 2567–2569 CAS.
|
This journal is © The Royal Society of Chemistry 2023 |
Click here to see how this site uses Cookies. View our privacy policy here.