DOI:
10.1039/C9RA05214C
(Review Article)
RSC Adv., 2019,
9, 26252-26262
Biomaterials for bone tissue engineering scaffolds: a review
Received
9th July 2019
, Accepted 24th July 2019
First published on 21st August 2019
Abstract
Bone tissue engineering has been continuously developing since the concept of “tissue engineering” has been proposed. Biomaterials that are used as the basic material for the fabrication of scaffolds play a vital role in bone tissue engineering. This paper first introduces a strategy for literature search. Then, it describes the structure, mechanical properties and materials of natural bone and the strategies of bone tissue engineering. Particularly, it focuses on the current knowledge about biomaterials used in the fabrication of bone tissue engineering scaffolds, which includes the history, types, properties and applications of biomaterials. The effects of additives such as signaling molecules, stem cells, and functional materials on the performance of the scaffolds are also discussed.
1. Introduction
Bone and its associated diseases, accounting for half of chronic diseases in people over 50 years old, still remain an important clinical challenge.1,2 Although bones have a certain healing and/or regeneration capacity, it cannot be accomplished by itself for large segmental bone defects. Large bone defects or injuries, caused by old age, traffic accident, fracture nonunion, bone tumor resection, etc., are serious problems in orthopaedics, and they bring great harms to health and the quality of life.3,4 Autologous bone grafting is still regarded as the “gold standard” for repairing bone defects. However, the drawbacks of autologous bone grafting include secondary damages, high donor site morbidity, limitation of special shape, insufficiency of autogenous bone and so on. These weaknesses limit its widespread use in clinical settings.
The term “tissue engineering” was first used in 1987.5 It is the utilization of a combination of multidisciplinary approaches to improve or replace biological tissues. In recent years, with the rapid development of tissue engineering technology, bone tissue engineering has become a hopeful approach for repairing bone defects. Scaffolds play a crucial role in bone tissue engineering. Their purpose is to mimic the structure and function of the natural bone extracellular matrix (ECM), which can provide a three-dimensional (3D) environment to promote the adhesion, proliferation, and differentiation and to have adequate physical properties for bone repair. An ideal scaffold should be biodegradable, biocompatible, bioactive, osteoconductive and osteoinductive. Artificial bone scaffolds with biomaterials and additives, such as drugs, growth factors (GFs) and stem cells, have been useful for bone repair.
The biomaterials (biomedical materials), which are basic components of scaffolds, play an important role in bone tissue engineering. Archaeological findings showed that materials such as human or animal bones and teeth, corals, shells, wood, and several metals (gold, silver and amalgam) were used for the replacement of missing human bones and teeth.6 For example, in the ancient times, the Etruscans learnt to replace damaged teeth with artificial graft obtained from the bones of oxen. In the early 1960s, the limitations of biological bone substitute materials resulted in the emergence of a multidisciplinary field called “Biomaterials”.7 Biomaterials are used for the evaluation, treatment, augmentation, repair or replacement of tissues or organs of the body. Ancient alternative materials are mostly bioinert (biologically inert), and these materials interact less with the surrounding tissues and are even toxic to humans. An ideal biomaterial should be non-cytotoxic, printable, biodegradable, bioactive, and osteoconductive in vivo. Due to the various needs of scaffolds, composite materials composed of two or more materials with excellent properties are widely used in bone tissue engineering.
Numerous natural and synthetic polymers such as calcium phosphates, calcium carbonate, and bioactive glasses have been used to fabricate scaffolds. Recent outstanding approaches include the addition of conductive polymers (CPs), inducerons (signaling molecules, unlike bone morphogenetic protein 2 (BMP-2)) and mechanical signals (elastic polymer networks such as hydrogels) to bone tissue engineering scaffolds. With the integration, intercrossing and development of the fields of medicine, biology, materials and other disciplines, biomaterials have been extensively used in the fabrication of bone tissue engineering scaffolds.8
This article gives a brief introduction to the descriptions of the hierarchical structure, chemical composition of natural bone and strategies for bone tissue engineering. It aims to outline the history, types, properties and development methods of common biomaterials used to fabricate scaffolds. Further, the review also highlights the biomaterial scaffolds with additives. Finally, it examines the combination of advanced technology and biomaterials, and emphasizes the challenges and opportunities of biomaterials in bone tissue engineering scaffolds.
2. Materials and methods
All studies (in vitro and in vivo) concerning the application of biomaterials to manufacture scaffolds for bone tissue engineering were researched in duplicate in the Medline (PubMed) online database. The PubMed search was performed to look for articles published in English between January 1, 2010 and January 1, 2019. The Medical Subject Heading (abbreviated as MeSH) terms “bone and bones”, “biocompatible materials” and “tissue scaffolds” were used together with the keywords “bone tissue engineering”, “biomaterials” and “scaffolds” to apply the following search strategy:
(((“Bone and Bones[Mesh] OR (bone[All Fields]) OR (bones[All Fields]) OR (“bones and bone”[All Fields]) OR (“bones and bone tissues”[All Fields]) OR (bone[All Fields] AND (tissue[All Fields] OR tissues[All Fields])) OR (“bone tissue”[All Fields]) OR (“bone tissues”[All Fields])) AND (“biocompatible materials”[Mesh] OR (“biocompatible materials”[All Fields]) OR ((material[All Fields]) AND (biocompatible[All Fields])) OR (biomaterials[All Fields]) OR (biomaterial[All Fields]) OR (“bioartificial materials”[All Fields]) OR (“bioartificial material”[All Fields]) OR ((material[All Fields]) AND (bioartificial[All Fields]))) AND (“tissue scaffolds”[Mesh] OR ((scaffold[All Fields] OR scaffolds[All Fields] OR scaffolding[All Fields] OR scaffoldings[All Fields]) AND tissue[All Fields]) OR (“tissue scaffold”[All Fields]) OR (“tissue scaffolding”[All Fields]) OR (“tissue scaffoldings”[All Fields]))) OR ((bone tissue engineering) AND (biomaterials) AND (scaffolds))) AND (“2010/01/01”[Date-Publication]: “2019/01/01”[Date-Publication]).9,10
The follow-up period or sample size is not limited. Meta analyses and systematic reviews were not included. Scientific research regarding the following topics was not considered: scaffolds for assisted positioning of transplants and help with surgical planning before the surgery.
2.1 Study selection
Two of the authors individually selected the titles and abstracts of the articles obtained by the above-mentioned search. Then, the selected studies were independently carefully sifted by both of the reviewers. Any disagreement was determined through discussions between them.
2.2 Data extraction
Two of the authors separately summarized the search and sought consensus among other authors in the process. The undermentioned information was recorded: the publication information including the author's name and publication data, the biomaterials applied to manufacture scaffolds and their important characteristics.
3. Structure, mechanical properties and materials of natural bone
3.1 Hierarchical structure of bone
As the main part of the human skeletal system, bone plays a crucial role in providing structure, supporting mechanical movement, protecting organs, and producing and hosting blood cells. It has a complex hierarchical structure based on the length and width scale, which consists of the macro scale (trabecular bone, also known as cancellous or spongy bone, and compact bone, also named cortical bone), microscale and sub-microscale (haversian canals, osteons and lamellae), nanoscale (fibrillar collagen) and sub-nanoscale (such as minerals, collagen and so on), as shown in Fig. 1.11 The structure of natural bone has been presented in various articles.11–21 Compact bone is nearly solid, except for ∼3–5% of rooms for canaliculi, osteocytes and so on.18 However, trabecular bone is an interconnected porous network and has a higher bone surface-to-bone volume (BS/BV) ratio than compact bone.
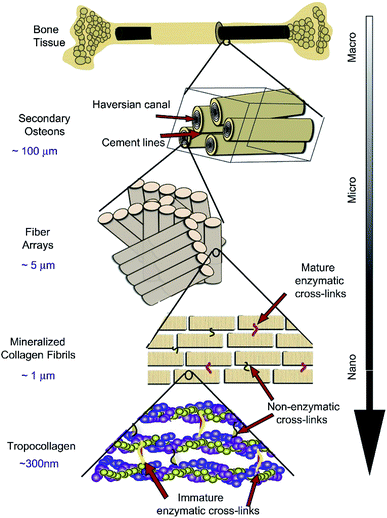 |
| Fig. 1 Hierarchical structure of natural bone. Reproduced from ref. 11 with permission from Elsevier, copyright 2011. | |
3.2 Mechanical properties of bone
The mechanical properties of natural bone vary greatly with respect to age and the body part. Young's modulus and yield stress of natural bone are anisotropic. A complete understanding of the mechanics of living bones remains an important scientific challenge. Table 1 shows the mechanical properties of natural bone obtained from the reported data.18 The longitudinal direction of the compact bone is robuster and stiffer than its transverse direction. The trabecular bone has a porous structure, and the porosity and arrangement of the individual trabeculae determine its mechanical properties.
Table 1 Mechanical properties of natural bone
|
Modulus (GPa) |
|
Strength (MPa) |
|
Poisson's ration |
Compact bone |
Longitudinal |
17.9 ± 3.9 |
Tension |
135 ± 15.6 |
0.4 ± 0.16 |
Compression |
205 ± 17.3 |
|
Transverse |
10.1 ± 2.4 |
Tension |
53 ± 10.7 |
0.62 ± 0.26 |
Compression |
131 ± 20.7 |
|
Shear |
3.3 ± 0.4 |
Shear |
65 ± 4.0 |
|
Trabecular bone |
Vertebra |
0.067 ± 0.045 |
|
2.4 ± 1.6 |
|
Tibia |
0.445 ± 0.257 |
|
5.3 ± 2.9 |
|
Femur |
0.441 ± 0.271 |
|
6.8 ± 4.8 |
|
3.3 Natural composition of bone
The understanding of the material components of natural bone plays a crucial role in the selection of scaffold materials. Natural bone consists of cells, ECM assembled from collagen fibrils and hydroxyapatite (HA), and bound minerals. Collagen and HA together account for ∼95% of natural bone under dry conditions.21 The composition of natural bone is presented in Table 2.19 Biological apatites deviate from the stoichiometric composition of HA and contain certain amounts of ion substitution impurities such as Na+, Mg2+, Cl−, K+, F−, and Zn2+. HA is the major inorganic component of human skeleton.
Table 2 Chemical composition of bone (wt%)
Inorganic Phase |
Organic Phase |
HA ≈ 60 |
Collagen≈20 |
H2O ≈ 9 |
Noncollagenous proteins≈3 |
Carbonate ≈ 4 |
Traces: polysaccharides, lipids, and cytokines |
Citrate ≈ 0.9 |
Primary bone cell: osteoblasts, osteocytes, and osteoclasts |
Na+ ≈ 0.7 |
|
Mg2+ ≈ 0.5 |
|
Cl− |
|
Others: K+, F−, Zn2+, Fe2+,Cu2+,Sr2+, and Pb2+ |
|
4. Bone tissue engineering
Although human bones have a certain self-healing ability, they are powerless for large bone defects. To overcome the problems, bone tissue engineering is proposed on the basis of tissue engineering. Bone tissue engineering aims to induce new tissue repairing and regeneration by the synergy of cells, signals and scaffolds.8 A scaffold composed of biomaterials is a carrier of cells and signals. It plays a key role in bone tissue engineering. Strategies for bone tissue engineering are shown in Fig. 2.22
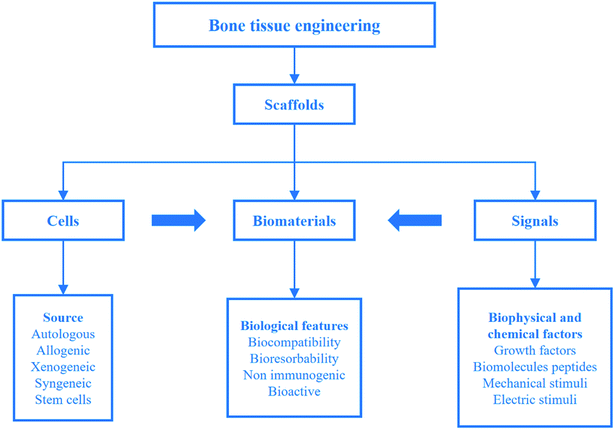 |
| Fig. 2 Strategies for bone tissue engineering. Reproduced from ref. 22 with permission from Springer, copyright 2018. | |
For the large-sized tissues and origins with different shapes, it is necessary to design a temporary support to provide spaces for cell proliferation, differentiation and growth. The support is called scaffold, transplant, template or artificial ECM. As noticed before, an ideal scaffold should have biocompatibility, suitable mechanical properties, high porosity and gradient pore structure. As the new tissue grows, the implanted scaffold gradually degrades until the new tissue completely replaces it. The design and fabrication of scaffolds with customization can be obtained by computer-aided design and computer-aided manufacturing (CAD/CAM) technology. Biomaterials are an important part of the scaffolds, and an ideal biomaterial should possess the following characteristics: (1) biocompatibility; (2) biodegradability; (3) easy printing and processing. During the last decades, researchers have shown increasing interest towards biomaterials for their application in bone tissue engineering scaffolds.
Generally, the obtained scaffolds should be biologically investigated. The main approaches of biological research in vitro as forecasting test before pre-clinical can be divided into two main categories: (1) in vitro culture experiments such as scaffold toxicity tests, animal or human cells (such as BMSCs,23 hMSCs,24 etc.) and (2) in vivo animal experiments (such as repairing of femur defects in rats).25 Scaffolds with non-toxic, good biocompatibility are the basis of bone repair and regeneration, in which biomaterials play an important role in the excellent performance of the scaffolds.
5. Various biomaterials for bone tissue engineering scaffolds
5.1 History of biomaterials
In the long history of human development, tissues and organs have evolved with respect to function after millions of years, but humans have been using artificial substitutes to repair damaged tissues only for decades. In the year 659 AD, the Chinese first used dental amalgam to repair defects in teeth.26 The limitations of bone replacement materials have resulted in the utilization of synthetic alternative materials for bone repair, replacement and enhancement. “Biomaterials” appeared in the early 1960s.7 The history of using biomaterials for scaffolds based on three different generations is briefly introduced below.8
The first generation of biomaterials appeared in the 1960s.27 It aimed to achieve the performance of the biomaterial to match the replaced tissue with the least toxic reaction to the host. They are generally bioinert, and interact minimally with the surrounding tissues. The first generation of biomaterials mainly includes: metals (such as titanium or titanium alloys), synthetic polymers (such as PMMA and PEEK) and ceramics (such as alumina and zirconia).
The most important feature of the second-generation biomaterials is their bioactive nature, and some could be biodegradable in vivo. They consist of synthetic and natural polymers (e.g. collagen), calcium phosphates, calcium carbonate, calcium sulfates, and bioactive glasses.
The third generation of biomaterials are designed to induce specific beneficial biological responses by the addition of instructive substances based on the second-generation biomaterials with excellent properties and/or new biomaterials with outstanding performance. Some of the instructive substances include, but are not limited to, biological factors or external stimuli.
5.2 Simple biomaterial scaffolds
Biomaterials such as metals, natural polymers, synthetic polymers, ceramics, and their composites have been widely used in biomedical fields for decades. Fig. 3a indicates the values (normalized by density) of stiffness and the strength of various materials by an Ashby plot.17 Natural materials, except silk that exhibits excellent toughness, have much lower values of strength and toughness than engineering materials. However, many natural materials have a toughness value that far exceeds their composition and their homogeneous mixture (as shown by the dashed line in Fig. 3b).17 Selection of matrix material plays a crucial role in the properties of bone scaffolds. Various polymers have been developed to fabricate bone tissue engineering scaffolds. An overview of different biomaterials including their characteristics, advantages, and disadvantages is given in Table 3.
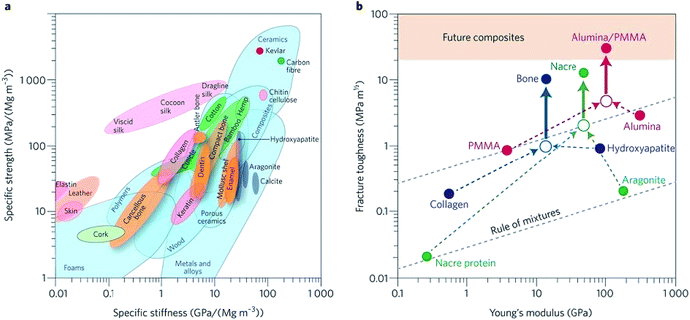 |
| Fig. 3 Performance of natural and synthetic materials. (a) Ashby chart of strength and stiffness for natural and synthetic materials. (b) Calculation for natural and synthetic materials. Reproduced from ref. 17 with permission from Nature Publishing Group, copyright 2014. | |
Table 3 Outlined characteristics of various biomaterials used to fabricate bone tissue engineering scaffolds
Biomaterials |
Characteristics |
Advantages |
Disadvantages |
Ref. |
Metal |
Suitable mechanical properties of biocompatible metallic scaffolds |
Outstanding mechanical properties |
Non-biodegradable |
|
Biocompatible |
Corrosion |
Tantalum |
Bioactive and corrosion resistance |
Extensively used as implant biomaterials |
Almost no degradation lead to a second surgery for removing the implant |
28–32 |
Magnesium |
Good porous and biodegradable implant |
Mechanical properties similar to human bone |
Toxicity risk caused by metal ion or particle leaching |
33–39 |
Biodegradable |
Titanium and titanium alloys |
Durable, biocompatible, highly corrosion resistant and very similar modulus of elasticity for trabecular bone |
High bone affinity |
Non-biodegradable |
40–44 |
Nickel-titanium alloy (nitinol) |
Particular mechanical properties (such as the shape memory and superelastic effects) |
Low modulus of elasticity, pseudo-elasticity, and high damping capacity, better match the properties of natural bone better than any other metals |
Almost no degradation for nitinol, the relatively high stiffness of titanium can cause stress shielding and implant loosening |
45–48 |
Natural polymer |
Similarity to ECM, specific degradation rates and good biological properties |
Biocompatible |
Low mechanical strength |
|
Degradation |
Collagen |
Important part of natural bone organic materials. Excellent biocompatibility |
Biodegradable |
Disinfection and handling are relatively difficult |
49–51 |
Various forms of scaffolds (e.g., sheets) |
Gelatin |
Denaturalized collagen |
Forming blends through cross-linking |
|
52–55 |
Silk fibroin |
Silk fibroin with outstanding mechanical properties |
|
|
56–58 |
Chitosan |
Polysaccharide with positive charge, biocompatibility and resistance to bacteria |
|
|
59 |
Alginate |
Polysaccharide with negative charge, and can crosslink and print by injection |
|
|
60–62 |
Hyaluronic acid |
Glycosaminoglycan with negative charge, biocompatibility, forming hydrogel through cross-linking |
Ease to chemical functionalization and degradability |
|
58,63–66 |
Synthetic polymer |
|
Changeable mechanical and physical properties |
Possible adverse tissue reactions caused by acidic degradation |
|
PLA, PGA and PLGA |
FDA-approved materials for clinical applications |
Water solubility and crystallinity tunable by changing hydroxylation degree |
Non-hydrophobic and shortage of cell adhesion |
67–69 |
PCL |
Excellent crystallinity and mechanical properties |
An crosslink in situ and print by injection |
Degradation rate in years |
70–73 |
PVA |
Hydroxylated synthetic polyvinyl acetate |
Ability to manufacture implants with various characteristics such as shape, porosity and degradation rate |
|
74–77 |
PPF |
Has numerous nonsaturable double bonds and the crosslinks may be toxic |
Adjustable mechanical strength and rates of degradation |
|
78,79 |
Polyurethane (PU) |
Remarkable mechanical properties |
|
|
80–82 |
Bioinert ceramic |
Cannot perform medical reactions with living tissue after implantation |
|
|
|
Aluminum, e.g., α-aluminum oxide (Al2O3) |
Improve mechanical properties; lack of biological activity |
|
|
83–86 |
Zirconia |
Interconnected structures; lack of chemical bonds and biological reactions between living tissues |
|
|
87–89 |
Bioactive ceramic |
Can show medical reactions with living tissue after implantation |
|
|
|
HA |
The main inorganic component of natural bone |
Highly biocompatible, non-toxic and osteoconductive |
|
6,85,90,91 |
Tricalcium phosphate (TCP), e.g., beta-tricalcium phosphate (β-TCP) |
The ratio of calcium to phosphorus is close to natural bone tissue |
Biocompatibility, no rejection and can provide calcium and phosphorus for new tissue |
α-TCP has excessive dissolution and rapid degradation |
56,92–95 |
Degradation rate and osteogenic speed are inconsistent |
Calcium sulfate (CaSO4) |
CaSO4 is a good material to choose after tumor resection |
|
|
96–99 |
Akermanite (ca, Si, Mg) |
Excellent mechanical properties and controllable degradation rate |
|
|
100–102 |
Better osteogenic differentiation and increased gene expression compared to β-TCP |
Diopside (MgCaSi2O6) |
Low temperature and fast firing and good thermal expansion properties |
|
|
103–106 |
Bioactive glasses (BGs) |
The main components for Na2O, CaO, SiO2 and P2O5; brittleness |
|
|
107–113 |
5.3 Composite biomaterial scaffolds
Composite biomaterials are designed to combine two or more materials. The purpose of using composite materials is mainly to improve the processability, printing performance, mechanical properties and bioactivity of the scaffolds. Ti6Al4V, HA, β-TCP and BG are widely used as bioactive biomaterials due to specific biological reactions between scaffolds and living tissues. Bioresorbable biomaterials applied in bone tissue engineering are generally natural polymers (such as collagen, gelatin, silk fibroin, and chitosan), synthetic polymers (such as PLA, PGA, and PCL) and ceramic (such as HA, β-TCP, and BGs). Scaffolds containing additives (such as GFs) have been used in clinical applications because of their excellent bone regeneration capabilities. The general composite biomaterial scaffolds with additives (signaling molecules, stem cells, functional materials, and so on) for bone tissue engineering are summarized in Table 4, which include metal matrix composites, polymer matrix composites, ceramic matrix composites, and functional composites.
Table 4 Summary of composites materials used to manufacture scaffolds for bone tissue engineering
Type |
Raw materials |
Additives |
Study outcome |
Ref. |
Metal matrix composites |
Ti6Al4V |
|
Young's modulus similar to human natural bone, improved the mechanical shielding |
114,115 |
Ti6Al4V |
Tantalum (Ta) |
Better bone ingrowth in Ta-coated scaffolds |
116 |
Ti6Al4V |
Simvastatin/Hydrogel |
Significantly improved neovascularization, osteointegration and bone ingrowth |
117 |
Ti6Al4V |
HA/pDA |
Significantly promoted bone regeneration and improved osteointegration and osteogenesis |
118 |
Ti6Al4V/Fibrin glue |
Vascular endothelial growth factor (VEGF) and BMP-2 |
Significantly enhanced both osteogenesis and angiogenesis for a single factor or dual factors, but synergistic effects of two-factor combination can observe angiogenesis but lack osteogenesis |
119 |
Polymer matrix composites |
Bioactive glass (BG) |
Collagen-glycosaminoglycan (CG) |
Promoting bone tissue regeneration and overcoming the problem of inadequate graft vascularization in tissue engineering |
120 |
Poly(L/DL lactide) (PLDL)/PCL |
Osteogenon-drug |
Use of osteogenon improves mineralization, cell adhesion and cell differentiation |
121 |
PEG/PU |
BMSCs |
The polymer matrix is highly thermally stable, regulatable, degradable at an acidic pH (5.8), biodegradable, cell compatible and has excellent porosity |
23 |
PLA |
Bioactive organically modified glass (ormoglass) |
The fibers are coated with different ormoglass components and their properties (roughness, stiffness and morphology) are adjusted by altering the trial parameters |
122 |
Poly(D,L-Lactide) (PDLLA) |
BGs and CuO/ZnO |
By appropriately adding Cu- and Zn-doped BG in the PDLLA, composite scaffolds can be obtained with improved bioactivity |
123 |
Ceramic matrix composites |
Titanium dioxide |
PLGA/gentamicin |
Confirmed the effective antibacterial activity of the released gentamicin and the compatibility of the scaffold on osteoblast-like cells(MG-63) |
124 |
HA/β-TCP |
BMP-2 |
Real application possibilities for bone tissue engineering purposes |
125 |
β-TCP |
Iron-containing |
Iron maybe help to promote the bone conduction properties of calcium phosphate (CaP) ceramics |
126 |
n-HA/poly(D,L-lactide-co-glycolide) (PLAGA) |
hMSCs |
Allowing for the generation of engineered bone tissue |
24 |
HA/Poly(D,L-lactic acid)-co-poly-(ethylene glycol)-co-poly(D,L-lactic acid) (PELA) |
BMSCs/rhBMP-2 |
Making the scaffolds suitable for evaluating bone regeneration approaches based on cell/the PELA/HA scaffolds with 500 ng of rhBMP-2 |
25 |
Functional composites |
Photocrosslinking of PCL and bioactive polydopamine coating |
Temperature |
The capacity to automatically fit into irregular defects and superior bioactivity because of polydopamine-coating |
127 |
Polypyrrole (PPy), HA, gelatin and mesoporous silica |
Electrical stimulation |
Good mechanical properties, higher protein adsorption |
128 |
PLGA |
Black phosphorus (BP)/SrCl2 |
The obtained scaffolds had good biocompatibility and good bone regeneration ability under near-infrared (NIR) irradiation in vivo in rats |
129 |
Polypyrrole/alginate (PPy/Alg) |
hMSCs and electrical stimulation |
Enhanced cell adhesion and growth |
130 |
Gelatin/bioactive glass |
Poly(3,4-ethylenedioxythiophene):poly(4-styrene sulfonate) (PEDOT:PSS) and electrical stimulation |
Adding PEDOT stabilizes the structure of scaffolds and enhances the cellular properties of mesenchymal stem cells |
131 |
Transglutaminase cross-linked gelatin (TG-Gel) |
BMP-2, matrix rigidity and mechanical signaling |
The combination of hydrogel hardness and BMP-2 has a synergistic effect on cellular osteogenic differentiation |
132 |
Bioactive metal matrix composites are widely used in clinical medical settings because of their outstanding mechanical properties, excellent biocompatibility, thermal stability, and corrosion resistance. Titanium, tantalum and their respective alloys are considered to be the preferred biomaterials for scaffolds. However, the high costs of manufacturing scaffolds limit their widespread development. Ti6Al4V is an outstanding representative of metal matrix composites. Young's modulus of the suitable porous Ti6Al4V scaffolds can be similar to natural bone and improve the mechanical shielding to the living tissue.114,115 The Ti6Al4V scaffolds can significantly increase bone ingrowth, osteointegration, and osteogenesis by covering the tantalum coating,116 adding simvastatin/hydrogel,117 or polydopamine-assisted hydroxyapatite coating (HA/pDA),118 as summarized in Table 4. Although metal matrix composites, such as Ti6Al4V, have many outstanding advantages; the non-biodegradable properties of metal matrix composites fundamentally limit their potential to become ideal materials.
In recent years, the application of polymer matrix composites and ceramic matrix composites has made great progress in bone tissue engineering scaffolds. Polymer composites have various excellent properties, such as biodegradability and mechanical properties.122–131 Ceramic materials, especially HA, are the main inorganic constituents of natural bone.19 Composite materials composed of ceramic materials and polymer materials have desirable properties for the manufacturing of scaffolds for bone tissue engineering.125,126,130,131 The composite scaffolds with additives (signaling molecules, stem cells and functional materials) have superior performance compared to just composite scaffolds (Table 4). The composite scaffolds with additives could further enhance the performance of the scaffolds. As shown in Fig. 4a, scaffolds with bioactive polydopamine coating have the capacity to automatically fit into irregular defects at higher temperatures. Wang et al. fabricated BP-SrCl2/PLGA scaffolds for rat femoral defects, and the near-infrared light-triggered platform significantly enhanced bone regeneration, as seen in Fig. 4b.129
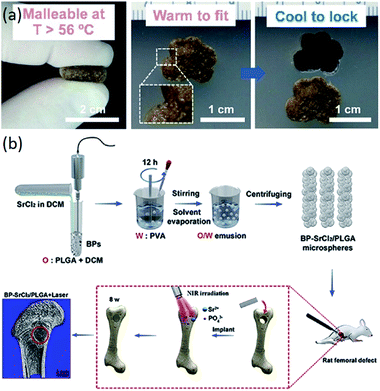 |
| Fig. 4 Functional composite bone tissue engineering scaffolds. (a) Effect of temperature on the scaffolds. Reproduced from ref. 127 with permission from Elsevier, copyright 2014. (b) Effect of near-infrared light on the scaffolds. Reproduced from ref. 129 with permission from Elsevier, copyright 2018. | |
6. Conclusion
In this paper, the summarized literature, which involves biomaterials for bone tissue engineering scaffolds, has been reviewed. The application and properties of various biomaterials used to fabricate scaffolds have also been elaborated. In particular, composite materials such as metal matrix composites, polymer matrix composites, ceramic matrix composites, and functional composites have been discussed. It was found that additives such as signaling molecules, stem cells, and functional materials can enhance the performance of the scaffolds. Although it was impossible forty years ago to find a material that is not repelled by living tissue, nowadays biomaterials have been used for bone repair. Improved performance of ideal biomaterials is required for their positive interactions with host tissues. The approaches for bone regeneration will make giant steps with the exploitation of novel biomaterials and new strategies, particularly the deep integration of nanotechnology, stem cell science and other fields.
Abbreviations
3D | Three-dimensional |
ECM | Bone extracellular matrix |
GFs | Growth factors |
CPs | Conducting polymers |
MeSH | Medical subject heading |
BS/BV | Bone surface to bone volume |
HA | Hydroxyapatite |
n-HA | Nano-hydroxyapatite |
CAD | Computer-aided design |
CAM | Computer-aided manufacturing |
PMMA | Poly(methyl methacrylate) |
PEEK | Polyether ether ketone |
PLA | Poly(lactic acid) |
PGA | Poly(glycolic acid) |
PLGA | Poly(lactic-co-glycolic acid) |
PVA | Poly(vinyl alcohol) |
PPF | Poly(propylene fumarate) |
PU | Polyurethane |
Al2O3 | α-Aluminum oxide |
TCP | Tricalcium phosphate |
β-TCP | beta-tricalcium phosphate |
CaP | Calcium phosphate |
CaSO4 | Calcium sulphate |
HA/pDA | polydopamine-assisted hydroxyapatite coating |
Ta | Tantalum |
CG | Collagen-glycosaminoglycan |
PLDL | Poly(L/DL lactide) |
BP | Black phosphorus |
NIR | Near-infrared |
BMSCs | Bone marrow stromal cells |
PEG | Poly-(ethylene glycol) |
PDLLA | Poly(D,L-Lactide) |
BMP-2 | Bone morphogenetic protein 2 |
VEGF | Vascular endothelial growth factor |
PELA | Poly(D,L-lactic acid)-co-poly-(ethylene glycol)-co-poly(D,L-lactic acid) |
PLAGA | Poly(D,L-lactide-co-glycolide) |
rhBMP-2 | Recombinant human bone morphogenetic protein-2 |
PPy/Alg | Polypyrrole/alginate |
hMSCs | Human mesenchymal stem cells |
PEDOT | PSS poly(3,4-ethylenedioxythiophene): poly(4-styrene sulfonate) |
TG-Gel | Transglutaminase cross-linked gelatin |
Conflicts of interest
There are no conflicts to declare.
Acknowledgements
This research was supported by National Natural Science Foundation of China (81704054).
References
- M. R. Brinker and D. P. O'Connor, J. Bone Jt. Surg., 2004, 86A, 290–297 CrossRef.
- B. Baroli, J. Pharm. Sci., 2009, 98, 1317–1375 CrossRef CAS PubMed.
- C. Laurencin, Y. Khan and S. F. El-Amin, Expert Rev. Med. Devices, 2006, 3, 49–57 CrossRef CAS PubMed.
- X. H. Wang, Q. Ao, X. H. Tian, J. Fan, Y. J. Wei, W. J. Hou, H. Tong and S. L. Bai, Materials, 2016, 9, 23 CrossRef PubMed.
- R. Langer and J. P. Vacanti, Science, 1993, 260, 920–926 CrossRef CAS PubMed.
- S. V. Dorozhkin, Biomaterials, 2010, 31, 1465–1485 CrossRef CAS PubMed.
- F. Burny, M. Donkerwolcke and D. Muster, Materials Science and Engineering: A, 1995, 199, 53–59 CrossRef CAS.
- X. H. Yu, X. Y. Tang, S. V. Gohil and C. T. Laurencin, Adv. Healthc. Mater., 2015, 4, 1268–1285 CrossRef CAS PubMed.
- G. Brunello, S. Sivolella, R. Meneghello, L. Ferroni, C. Gardin, A. Piattelli, B. Zavan and E. Bressan, Biotechnol. Adv., 2016, 34, 740–753 CrossRef CAS PubMed.
- W. Yu, X. Sun, H. Y. Meng, B. C. Sun, P. Chen, X. J. Liu, K. H. Zhang, X. Yang, J. Peng and S. B. Lu, Biomater. Sci., 2017, 5, 1690–1698 RSC.
- H. D. Barth, E. A. Zimmermann, E. Schaible, S. Y. Tang, T. Alliston and R. O. Ritchie, Biomaterials, 2011, 32, 8892–8904 CrossRef CAS PubMed.
- J. Y. Rho, L. Kuhn-Spearing and P. Zioupos, Med. Eng. Phys., 1998, 20, 92–102 CrossRef CAS PubMed.
- S. Weiner and H. D. Wagner, Annu. Rev. Mater. Res., 1998, 28, 271–298 CrossRef CAS.
- P. Fratzl, H. S. Gupta, E. P. Paschalis and P. Roschger, J. Mater. Chem., 2004, 14, 2115–2123 RSC.
- P. Fratzl and R. Weinkamer, Prog. Mater. Sci., 2007, 52, 1263–1334 CrossRef CAS.
- M. J. Olszta, X. G. Cheng, S. S. Jee, R. Kumar, Y. Y. Kim, M. J. Kaufman, E. P. Douglas and L. B. Gower, Mater. Sci. Eng. R Rep., 2007, 58, 77–116 CrossRef.
- U. G. K. Wegst, H. Bai, E. Saiz, A. P. Tomsia and R. O. Ritchie, Nat. Mater., 2015, 14, 23–36 CrossRef CAS PubMed.
- X. J. Wang, S. Q. Xu, S. W. Zhou, W. Xu, M. Leary, P. Choong, M. Qian, M. Brandt and Y. M. Xie, Biomaterials, 2016, 83, 127–141 CrossRef CAS PubMed.
- N. Eliaz and N. Metoki, Materials, 2017, 10, 104 CrossRef PubMed.
- S. Elsharkawy and A. Mata, Adv. Healthc. Mater., 2018, 7, 19 Search PubMed.
- H. S. Ma, C. Feng, J. Chang and C. T. Wu, Acta Biomater., 2018, 79, 37–59 CrossRef CAS PubMed.
- Pearlin, S. Nayak, G. Manivasagam and D. Sen, Curr. Osteoporos. Rep., 2018, 16, 169–181 CrossRef CAS PubMed.
- R. Geesala, N. Bar, N. R. Dhoke, P. Basak and A. Das, Biomaterials, 2016, 77, 1–13 CrossRef CAS PubMed.
- Q. Lv, M. Deng, B. D. Ulery, L. S. Nair and C. T. Laurencin, Clin. Orthop. Relat. Res., 2013, 471, 2422–2433 CrossRef PubMed.
- A. B. Kutikov, J. D. Skelly, D. C. Ayers and J. Song, ACS Appl. Mater. Interfaces, 2015, 7, 4890–4901 CrossRef CAS PubMed.
- M. E. Ring, Dentistry: An Illustrated History, Abrams, Inc., New York, 1985 Search PubMed.
- B. D. Ratner, A. S. Hoffman, F. J. Schoen and J. E. Lemons, Biomaterials Science: An Introduction to Materials in Medicine, Elsevier, San Diego, 2004 Search PubMed.
- K. Alvarez and H. Nakajima, Materials, 2009, 2, 790–832 CrossRef CAS.
- S. Bose, M. Roy and A. Bandyopadhyay, Trends Biotechnol., 2012, 30, 546–554 CrossRef CAS PubMed.
- K. Jamil, K. H. Chua, S. Joudi, S. L. Ng and N. H. Yahaya, J. Orthop. Surg. Res., 2015, 10, 27 CrossRef PubMed.
- A. Jonitz, K. Lochner, T. Lindner, D. Hansmann, A. Marrot and R. Bader, J. Mater. Sci.: Mater. Med., 2011, 22, 2089–2095 CrossRef CAS PubMed.
- D. A. Shimko, V. F. Shimko, E. A. Sander, K. F. Dickson and E. A. Nauman, J. Biomed. Mater. Res., Part B, 2005, 73, 315–324 CrossRef PubMed.
- K. F. Farraro, K. E. Kim, S. L. Woo, J. R. Flowers and M. B. McCullough, J. Biomech., 2014, 47, 1979–1986 CrossRef PubMed.
- K. J. Kim, S. Choi, Y. Sang Cho, S. J. Yang, Y. S. Cho and K. K. Kim, J. Mater. Sci.: Mater. Med., 2017, 28, 96 CrossRef PubMed.
- Y. Li, J. Zhou, P. Pavanram, M. A. Leeflang, L. I. Fockaert, B. Pouran, N. Tumer, K. U. Schroder, J. M. C. Mol, H. Weinans, H. Jahr and A. A. Zadpoor, Acta Biomater., 2018, 67, 378–392 CrossRef CAS PubMed.
- Y. J. Liu, Z. Y. Yang, L. L. Tan, H. Li and Y. Z. Zhang, Braz. J. Med. Biol. Res., 2014, 47, 715–720 CrossRef CAS PubMed.
- M. Nabiyouni, T. Bruckner, H. Zhou, U. Gbureck and S. B. Bhaduri, Acta Biomater., 2018, 66, 23–43 CrossRef CAS PubMed.
- M. Yazdimamaghani, M. Razavi, D. Vashaee, K. Moharamzadeh, A. R. Boccaccini and L. Tayebi, Mater. Sci. Eng., C, 2017, 71, 1253–1266 CrossRef CAS PubMed.
- M. Yazdimamaghani, M. Razavi, D. Vashaee and L. Tayebi, Mater. Sci. Eng., C, 2015, 49, 436–444 CrossRef CAS PubMed.
- Y. Chen, J. E. Frith, A. Dehghan-Manshadi, H. Attar, D. Kent, N. D. M. Soro, M. J. Bermingham and M. S. Dargusch, J. Mech. Behav. Biomed. Mater., 2017, 75, 169–174 CrossRef CAS PubMed.
- H. J. Haugen, M. Monjo, M. Rubert, A. Verket, S. P. Lyngstadaas, J. E. Ellingsen, H. J. Ronold and J. C. Wohlfahrt, Acta Biomater., 2013, 9, 5390–5399 CrossRef CAS PubMed.
- J. H. Kim, D. K. Kim, O. J. Lee, H. W. Ju, J. M. Lee, B. M. Moon, H. J. Park, D. W. Kim, J. H. Lee and C. H. Park, Int. J. Biol. Macromol., 2016, 82, 160–167 CrossRef CAS PubMed.
- J. Liu, J. Ruan, L. Chang, H. Yang and W. Ruan, Mater. Sci. Eng., C, 2017, 78, 503–512 CrossRef CAS PubMed.
- T. Takizawa, N. Nakayama, H. Haniu, K. Aoki, M. Okamoto, H. Nomura, M. Tanaka, A. Sobajima, K. Yoshida, T. Kamanaka, K. Ajima, A. Oishi, C. Kuroda, H. Ishida, S. Okano, S. Kobayashi, H. Kato and N. Saito, Adv. Mater., 2018, 30, 1703608 CrossRef PubMed.
- I. Gotman, D. Ben-David, R. E. Unger, T. Bose, E. Y. Gutmanas and C. J. Kirkpatrick, Acta Biomater., 2013, 9, 8440–8448 CrossRef CAS PubMed.
- T. Habijan, C. Haberland, H. Meier, J. Frenzel, J. Wittsiepe, C. Wuwer, C. Greulich, T. A. Schildhauer and M. Koller, Mater. Sci. Eng., C, 2013, 33, 419–426 CrossRef CAS PubMed.
- W. Hoffmann, T. Bormann, A. Rossi, B. Muller, R. Schumacher, I. Martin, M. de Wild and D. Wendt, J. Tissue Eng., 2014, 5 CAS , 2041731414540674.
- S. Strauss, A. Neumeister, S. Barcikowski, D. Kracht, J. W. Kuhbier, C. Radtke, K. Reimers and P. M. Vogt, PLoS One, 2013, 8, e53309 CrossRef CAS PubMed.
- J. Elango, J. Zhang, B. Bao, K. Palaniyandi, S. Wang, W. Wenhui and J. S. Robinson, Int. J. Biol. Macromol., 2016, 91, 51–59 CrossRef CAS PubMed.
- J. Lee and G. Kim, ACS Appl. Mater. Interfaces, 2018, 10, 35801–35811 CrossRef CAS PubMed.
- Y. Wang, N. Van Manh, H. Wang, X. Zhong, X. Zhang and C. Li, Int. J. Nanomed., 2016, 11, 2053–2067 CAS.
- T. Mazaki, Y. Shiozaki, K. Yamane, A. Yoshida, M. Nakamura, Y. Yoshida, D. Zhou, T. Kitajima, M. Tanaka, Y. Ito, T. Ozaki and A. Matsukawa, Sci. Rep., 2014, 4, 4457 CrossRef PubMed.
- W. Shi, M. Sun, X. Hu, B. Ren, J. Cheng, C. Li, X. Duan, X. Fu, J. Zhang, H. Chen and Y. Ao, Adv. Mater., 2017, 29, 1701089 CrossRef PubMed.
- B. Wang, Y. Guo, X. Chen, C. Zeng, Q. Hu, W. Yin, W. Li, H. Xie, B. Zhang, X. Huang and F. Yu, Int. J. Nanomed., 2018, 13, 7395–7408 CrossRef CAS PubMed.
- Y. Xia, F. Mei, Y. Duan, Y. Gao, Z. Xiong, T. Zhang and H. Zhang, J. Biomed. Mater. Res. A, 2012, 100, 1044–1050 CrossRef.
- D. H. Lee, N. Tripathy, J. H. Shin, J. E. Song, J. G. Cha, K. D. Min, C. H. Park and G. Khang, Int. J. Biol. Macromol., 2017, 95, 14–23 CrossRef CAS.
- J. Melke, S. Midha, S. Ghosh, K. Ito and S. Hofmann, Acta Biomater., 2016, 31, 1–16 CrossRef CAS.
- S. Gokila, T. Gomathi, K. Vijayalakshmi, F. A. Alsharani, S. Anil and P. N. Sudha, Int. J. Biol. Macromol., 2018, 120, 876–885 CrossRef PubMed.
- S. Preethi Soundarya, A. Haritha Menon, S. Viji Chandran and N. Selvamurugan, Int. J. Biol. Macromol., 2018, 119, 1228–1239 CrossRef CAS PubMed.
- Y. M. Kolambkar, K. M. Dupont, J. D. Boerckel, N. Huebsch, D. J. Mooney, D. W. Hutmacher and R. E. Guldberg, Biomaterials, 2011, 32, 65–74 CrossRef CAS PubMed.
- J. Sun and H. Tan, Materials, 2013, 6, 1285–1309 CrossRef CAS PubMed.
- J. Venkatesan, I. Bhatnagar, P. Manivasagan, K. H. Kang and S. K. Kim, Int. J. Biol. Macromol., 2015, 72, 269–281 CrossRef CAS PubMed.
- N. Cui, J. Qian, T. Liu, N. Zhao and H. Wang, Carbohydr. Polym., 2015, 126, 192–198 CrossRef CAS PubMed.
- C. Manferdini, V. Guarino, N. Zini, M. G. Raucci, A. Ferrari, F. Grassi, E. Gabusi, S. Squarzoni, A. Facchini, L. Ambrosio and G. Lisignoli, Biomaterials, 2010, 31, 3986–3996 CrossRef CAS PubMed.
- J. Patterson, R. Siew, S. W. Herring, A. S. Lin, R. Guldberg and P. S. Stayton, Biomaterials, 2010, 31, 6772–6781 CrossRef CAS PubMed.
- E. D. Pre, G. Conti and A. Sbarbati, Stem Cell Rev. Rep., 2016, 12, 664–681 CrossRef CAS PubMed.
- S. H. Zaky, K. W. Lee, J. Gao, A. Jensen, K. Verdelis, Y. Wang, A. J. Almarza and C. Sfeir, Acta Biomater., 2017, 54, 95–106 CrossRef CAS PubMed.
- Y. Boukari, O. Qutachi, D. J. Scurr, A. P. Morris, S. W. Doughty and N. Billa, J. Biomater. Sci., Polym. Ed., 2017, 28, 1966–1983 CrossRef CAS PubMed.
- W. Shao, J. He, F. Sang, Q. Wang, L. Chen, S. Cui and B. Ding, Mater. Sci. Eng., C, 2016, 62, 823–834 CrossRef CAS PubMed.
- S. H. Ahn, H. J. Lee and G. H. Kim, Biomacromolecules, 2011, 12, 4256–4263 CrossRef CAS PubMed.
- S. N. Gorodzha, A. R. Muslimov, D. S. Syromotina, A. S. Timin, N. Y. Tcvetkov, K. V. Lepik, A. V. Petrova, M. A. Surmeneva, D. A. Gorin, G. B. Sukhorukov and R. A. Surmenev, Colloids Surf., B, 2017, 160, 48–59 CrossRef CAS PubMed.
- Z. Guo, J. Xu, S. Ding, H. Li, C. Zhou and L. Li, J. Biomater. Sci., Polym. Ed., 2015, 26, 989–1001 CrossRef CAS PubMed.
- F. Sharifi, S. M. Atyabi, D. Norouzian, M. Zandi, S. Irani and H. Bakhshi, Int. J. Biol. Macromol., 2018, 115, 243–248 CrossRef CAS PubMed.
- C. Gao, Q. Gao, Y. Li, M. N. Rahaman, A. Teramoto and K. Abe, J. Biomed. Mater. Res. A, 2012, 100, 1324–1334 CrossRef PubMed.
- X. Li, Y. Li, Y. Zuo, D. Qu, Y. Liu, T. Chen, N. Jiang, H. Li and J. Li, J. Biomed. Mater. Res. A, 2015, 103, 3226–3236 CrossRef CAS PubMed.
- D. Qu, J. Li, Y. Li, A. Khadka, Y. Zuo, H. Wang, Y. Liu and L. Cheng, J. Biomed. Mater. Res., Part B, 2011, 96, 9–15 CrossRef PubMed.
- T. Wan, G. K. Stylios, M. Giannoudi and P. V. Giannoudis, Injury, 2015, 46(suppl. 8), S39–S43 CrossRef PubMed.
- D. L. Alge, J. Bennett, T. Treasure, S. Voytik-Harbin, W. S. Goebel and T. M. Chu, J. Biomed. Mater. Res. A, 2012, 100, 1792–1802 CrossRef PubMed.
- R. Mishra, B. M. Roux, M. Posukonis, E. Bodamer, E. M. Brey, J. P. Fisher and D. Dean, Biomaterials, 2016, 77, 255–266 CrossRef CAS PubMed.
- M. Meskinfam, S. Bertoldi, N. Albanese, A. Cerri, M. C. Tanzi, R. Imani, N. Baheiraei, M. Farokhi and S. Fare, Mater. Sci. Eng., C, 2018, 82, 130–140 CrossRef CAS PubMed.
- W. Yang, S. K. Both, Y. Zuo, Z. T. Birgani, P. Habibovic, Y. Li, J. A. Jansen and F. Yang, J. Biomed. Mater. Res. A, 2015, 103, 2251–2259 CrossRef CAS PubMed.
- T. Yoshii, A. E. Hafeman, J. M. Esparza, A. Okawa, G. Gutierrez and S. A. Guelcher, J. Tissue Eng. Regener. Med., 2014, 8, 589–595 CrossRef CAS PubMed.
- D. Kytyr, P. Zlamal, P. Koudelka, T. Fila, N. Krcmarova, I. Kumpova, D. Vavrik, A. Gantar and S. Novak, Mater. Des., 2017, 134, 400–417 CrossRef CAS.
- M. L. Lastra, M. S. Molinuevo, I. Blaszczyk-Lezak, C. Mijangos and M. S. Cortizo, J. Biomed. Mater. Res. A, 2018, 106, 570–579 CrossRef CAS PubMed.
- S. Mondal, G. Hoang, P. Manivasagan, M. S. Moorthy, T. P. Nguyen, T. T. V. Phan, H. H. Kim, M. H. Kim, S. Y. Nam and J. Oh, Ceram. Int., 2018, 44, 15735–15746 CrossRef CAS.
- A. Pietraszek, A. Karewicz, M. Widnic, D. Lachowicz, M. Gajewska, A. Bernasik and M. Nowakowska, Colloids Surf., B, 2019, 173, 1–8 CrossRef CAS PubMed.
- A. Afzal, Mater. Express, 2014, 4, 1–12 CrossRef CAS.
- X. Q. Huang, H. Y. Yang, T. Luo, C. Huang, F. R. Tay and L. N. Niu, Acta Biomater., 2018, 67, 366–377 CrossRef CAS PubMed.
- M. Latifi, T. Talaei-Khozani, H. Mehraban-Jahromi, M. Sani, M. Sadeghi-Atabadi, A. Fazel-Anvari and M. Kabir-Salmani, Bioinspired, Biomimetic Nanobiomater., 2018, 7, 122–130 CrossRef.
- C. Yang, Z. G. Huan, X. Y. Wang, C. T. Wu and J. Chang, ACS Biomater. Sci. Eng., 2018, 4, 608–616 CrossRef CAS.
- Y. Lei, Z. L. Xu, Q. F. Ke, W. J. Yin, Y. X. Chen, C. Q. Zhang and Y. P. Guo, Mater. Sci. Eng., C, 2017, 72, 134–142 CrossRef CAS PubMed.
- G. Fielding and S. Bose, Acta Biomater., 2013, 9, 9137–9148 CrossRef CAS PubMed.
- D. Ke and S. Bose, Mater. Sci. Eng., C, 2017, 78, 398–404 CrossRef CAS PubMed.
- R. Taktak, A. Elghazel, J. Bouaziz, S. Charfi and H. Keskes, Mater. Sci. Eng., C, 2018, 86, 121–128 CrossRef CAS PubMed.
- K. Yang, J. Zhang, X. Ma, Y. Ma, C. Kan, H. Ma, Y. Li, Y. Yuan and C. Liu, Mater. Sci. Eng., C, 2015, 56, 37–47 CrossRef CAS PubMed.
- X. Qi, P. Pei, M. Zhu, X. Du, C. Xin, S. Zhao, X. Li and Y. Zhu, Sci. Rep., 2017, 7, 42556 CrossRef CAS PubMed.
- Y. Shen, S. Yang, J. Liu, H. Xu, Z. Shi, Z. Lin, X. Ying, P. Guo, T. Lin, S. Yan, Q. Huang and L. Peng, ACS Appl. Mater. Interfaces, 2014, 6, 12177–12188 CrossRef CAS PubMed.
- C. Shuai, J. Zhou, D. Gao, C. Gao, P. Feng and S. Peng, Molecules, 2016, 21, 378 CrossRef PubMed.
- Z. Zhou, F. Buchanan, C. Mitchell and N. Dunne, Mater. Sci. Eng., C, 2014, 38, 1–10 CrossRef CAS PubMed.
- A. Liu, M. Sun, X. Yang, C. Ma, Y. Liu, X. Yang, S. Yan and Z. Gou, J. Biomater. Appl., 2016, 31, 650–660 CrossRef CAS PubMed.
- H. Shokrollahi, F. Salimi and A. Doostmohammadi, J. Mech. Behav. Biomed. Mater., 2017, 74, 365–370 CrossRef CAS PubMed.
- C. Shuai, Z. Han, P. Feng, C. Gao, T. Xiao and S. Peng, J. Mater. Sci.: Mater. Med., 2015, 26, 188 CrossRef PubMed.
- Z. Ba, Z. Chen, Y. Huang, D. Feng, Q. Zhao, J. Zhu and D. Wu, Int. J. Nanomed., 2018, 13, 3883–3896 CrossRef CAS PubMed.
- J. P. Kumar, L. Lakshmi, V. Jyothsna, D. R. Balaji, S. Saravanan, A. Moorthi and N. Selvamurugan, J. Biomed. Nanotechnol., 2014, 10, 970–981 CrossRef CAS.
- T. Liu, P. Wu, C. Gao, P. Feng, T. Xiao, Y. Deng, C. Shuai and S. Peng, BioMed Res. Int., 2016, 2016, 7090635 Search PubMed.
- C. Wu, Y. Ramaswamy and H. Zreiqat, Acta Biomater., 2010, 6, 2237–2245 CrossRef CAS PubMed.
- D. Bellucci, A. Sola, R. Salvatori, A. Anesi, L. Chiarini and V. Cannillo, Mater. Sci. Eng., C, 2014, 43, 573–586 CrossRef CAS PubMed.
- C. Bian, H. Lin, F. Zhang, J. Ma, F. Li, X. Wu and F. Qu, IET Nanobiotechnol., 2014, 8, 275–281 CrossRef PubMed.
- A. Goel, S. Kapoor, R. R. Rajagopal, M. J. Pascual, H. W. Kim and J. M. Ferreira, Acta Biomater., 2012, 8, 361–372 CrossRef CAS PubMed.
- Y. Gu, W. Huang, M. N. Rahaman and D. E. Day, Acta Biomater., 2013, 9, 9126–9136 CrossRef CAS PubMed.
- A. Hoppe, N. S. Guldal and A. R. Boccaccini, Biomaterials, 2011, 32, 2757–2774 CrossRef CAS PubMed.
- B. Sarker, J. Hum, S. N. Nazhat and A. R. Boccaccini, Adv. Healthc. Mater., 2015, 4, 176–194 CrossRef CAS PubMed.
- D. Sriranganathan, N. Kanwal, K. A. Hing and R. G. Hill, J. Mater. Sci.: Mater. Med., 2016, 27, 39 CrossRef.
- F. Yang, C. Chen, Q. Zhou, Y. Gong, R. Li, C. Li, F. Klampfl, S. Freund, X. Wu, Y. Sun, X. Li, M. Schmidt, D. Ma and Y. Yu, Sci. Rep., 2017, 7, 45360 CrossRef CAS PubMed.
- L. Y. Zhu, L. Li, J. P. Shi, Z. A. Li and J. Q. Yang, Am. J. Transl. Res., 2018, 10, 3443–3454 Search PubMed.
- X. Li, L. Wang, X. Yu, Y. Feng, C. Wang, K. Yang and D. Su, Mater. Sci. Eng., C, 2013, 33, 2987–2994 CrossRef CAS PubMed.
- H. Liu, W. Li, C. Liu, J. Tan, H. Wang, B. Hai, H. Cai, H. J. Leng, Z. J. Liu and C. L. Song, Biofabrication, 2016, 8, 045012 CrossRef PubMed.
- Y. Li, W. Yang, X. Li, X. Zhang, C. Wang, X. Meng, Y. Pei, X. Fan, P. Lan, C. Wang, X. Li and Z. Guo, ACS Appl. Mater. Interfaces, 2015, 7, 5715–5724 CrossRef CAS.
- J. Lv, P. Xiu, J. Tan, Z. Jia, H. Cai and Z. Liu, Biomed. Mater., 2015, 10, 035013 CrossRef PubMed.
- E. Quinlan, S. Partap, M. M. Azevedo, G. Jell, M. M. Stevens and F. J. O'Brien, Biomaterials, 2015, 52, 358–366 CrossRef CAS PubMed.
- I. Rajzer, E. Menaszek and O. Castano, Mater. Sci. Eng., C, 2017, 77, 493–499 CrossRef CAS PubMed.
- N. Sachot, M. A. Mateos-Timoneda, J. A. Planell, A. H. Velders, M. Lewandowska, E. Engel and O. Castano, Nanoscale, 2015, 7, 15349–15361 RSC.
- J. Bejarano, R. Detsch, A. R. Boccaccini and H. Palza, J. Biomed. Mater. Res. A, 2017, 105, 746–756 CrossRef CAS PubMed.
- L. Rumian, H. Tiainen, U. Cibor, M. Krok-Borkowicz, M. Brzychczy-Wloch, H. J. Haugen and E. Pamula, Mater. Sci. Eng., C, 2016, 69, 856–864 CrossRef CAS PubMed.
- A. Abarrategi, C. Moreno-Vicente, F. J. Martinez-Vazquez, A. Civantos, V. Ramos, J. V. Sanz-Casado, R. Martinez-Corria, F. H. Perera, F. Mulero, P. Miranda and J. L. Lopez-Lacomba, PLoS One, 2012, 7, e34117 CrossRef CAS PubMed.
- A. Manchon, M. Hamdan Alkhraisat, C. Rueda-Rodriguez, J. C. Prados-Frutos, J. Torres, J. Lucas-Aparicio, A. Ewald, U. Gbureck and E. Lopez-Cabarcos, Biomedical materials, Bristol, England, 2015, 10, p. 055012 Search PubMed.
- D. Zhang, O. J. George, K. M. Petersen, A. C. Jimenez-Vergara, M. S. Hahn and M. A. Grunlan, Acta Biomater., 2014, 10, 4597–4605 CrossRef CAS PubMed.
- N. Zanjanizadeh Ezazi, M. A. Shahbazi, Y. V. Shatalin, E. Nadal, E. Makila, J. Salonen, M. Kemell, A. Correia, J. Hirvonen and H. A. Santos, Int. J. Pharm., 2018, 536, 241–250 CrossRef CAS PubMed.
- X. Z. Wang, J. D. Shao, M. Abd El Raouf, H. H. Xie, H. Huang, H. Y. Wang, P. K. Chu, X. F. Yu, Y. Yang, A. M. AbdEl-Aal, N. H. M. Mekkawy, R. J. Miron and Y. F. Zhang, Biomaterials, 2018, 179, 164–174 CrossRef CAS PubMed.
- S. Yang, L. Jang, S. Kim, J. Yang, K. Yang, S. W. Cho and J. Y. Lee, Macromol. Biosci., 2016, 16, 1653–1661 CrossRef CAS PubMed.
- A. Shahini, M. Yazdimamaghani, K. J. Walker, M. A. Eastman, H. Hatami-Marbini, B. J. Smith, J. L. Ricci, S. V. Madihally, D. Vashaee and L. Tayebi, Int. J. Nanomed., 2014, 9, 167–181 Search PubMed.
- S. Tan, J. Y. Fang, Z. Yang, M. E. Nimni and B. Han, Biomaterials, 2014, 35, 5294–5306 CrossRef CAS PubMed.
|
This journal is © The Royal Society of Chemistry 2019 |
Click here to see how this site uses Cookies. View our privacy policy here.