DOI:
10.1039/C7QM00298J
(Research Article)
Mater. Chem. Front., 2017,
1, 2292-2298
A pH-sensitive multifunctional fluorescent probe based on N-annulated perylene for the sensitive and selective detection of hypochlorous acid†
Received
3rd July 2017
, Accepted 5th August 2017
First published on 8th August 2017
Abstract
Hypochlorous acid (HOCl) is a representative reactive oxygen species (ROS), which play important roles in signaling and homeostasis. And it is also an important cobleaching agent and disinfectant in industry and life. Therefore, it is of significant importance to detect HOCl accurately for the diagnosis or treatment of various diseases and the protection of the environment. Considering its excellent photophysical properties, N-annulated perylene (NP) was chosen as the fluorophore to design a multifunctional fluorescent probe for the sensitive and selective detection of HOCl for the first time. Benefiting from the introduction of a piperazine moiety, the probe became pH-sensitive due to the photo-induced electron transfer (PET) process. In a neutral environment, PNPM was a turn-on probe towards HOCl with a limit of detection of 31.7 nM. However, PNPM became a ratiometric fluorescent probe under weakly acidic conditions with a better limit of detection (15.3 nM). This amazing property meant that the probe possessed the ability to detect pH and HOCl at the same time. Therefore, PNPM was successfully employed to detect HOCl in fetal bovine serum and different water samples, where it could also monitor the pH values of the solution, showing its huge potential in practical applications.
Introduction
Reactive oxygen species (ROS), which play important roles in cell signaling and homeostasis, are chemically reactive chemical species containing oxygen. In a biological context, ROS are formed as a natural byproduct of the normal metabolism of oxygen, including peroxides, superoxides, hydroxyl radicals, singlet oxygen, and so on.1 Among these ROS, hypochlorous acid (HOCl) and its conjugate base hypochlorite (ClO−), which are both highly oxidizing agents in biological processes, are mainly produced by myeloperoxidase (MPO)-catalyzed per-oxidation of chloride ions in phagolysosomes.2,3 In the physical environment, HOCl serves as a “killer” for pathogens in the innate immune system. So maintaining a reasonable HOCl level in vivo is very essential for numerous cellular functions.4 But excessive or uncontrolled production of HOCl may cause tissue damage and various diseases, such as hepatic ischemia-reperfusion injury, atherosclerosis, neuron degeneration, arthritis, and cancer.5–7 Therefore, it is of great significance to develop highly sensitive and selective methods for the accurate detection of HOCl in complex biological environments.8 And in modern life and industry, hypochlorous acid is also commonly employed as a cobleaching agent and disinfectant for potable water. But excessive concentrations of HOCl in water would be harmful for the environment and human health.9 Therefore, it is also very important to detect HOCl accurately for the diagnosis or treatment of various diseases and monitoring the HOCl level in life and industrial production.
Compared with classic instrumental methods including potentiometry, coulometric titration and amperometry, fluorescent probes have attracted increasing interest due to their unique advantages under physiological conditions including high sensitivity, outstanding selectivity, rapid response rate and low cost.6,10 The past decade has witnessed much effort towards the development of small-molecule fluorescent probes for specific imaging and detection of HClO, which have been successfully employed to image HClO biological systems. However, most of these important contributions on HOCl probes are single-functional probes.11–21 So we wanted to develop a novel probe with multiple functions. As reported, the pH of a biological microenvironment is different, and the imbalance of pH in a biological environment may be linked with various diseases.22 And the complicated conditions of industrial and domestic water should also be paid much attention in environmental monitoring. Under this consideration, our expectation is to design a pH-sensitive fluorescent probe for HOCl detection, by which we could obtain different detection phenomena under different pH conditions. In this way, the pH value could also be measured while detecting HOCl.
Various fluorophores such as BODIPY,23–25 1,8-naphthalimide,26,27 rhodamine28,29 and coumarins30,31 have been applied to design a fluorescent probe for HOCl detection. But these fluorophores had obvious drawbacks when employed in practical applications. The small Stokes shifts and low synthetic productivity limit the application of BODIPY. Coumarin suffers a lot from its low brightness and short excitation wavelength. And the short fluorescence emission and few modification sites of 1,8-naphthalimide and rhodamine are both unfavorable for imaging in vivo.32 So it is desirable to develop a new organic fluorophore with excellent optical properties and stability. Recently, we have reported a sensing platform based on N-annulated perylene (NP). Compared with other perylene derivatives, the N-annulated perylene with a nitrogen atom annulated at the bay position also showed high fluorescence quantum yield and excellent stability. Besides, flexible alkyl chains or bulky groups can be readily introduced into the nitrogen atom, which could greatly improve the solubility and suppress dye aggregation effectively.33,34 As an electron donor, N-annulated perylene could be used to construct a donor–acceptor system by connecting an electron-withdrawing group to increase its absorption wavelength and fluorescence. However, N-annulated perylene has seldom been considered in the sensing field to date.
In this work, N-annulated perylene was chosen as a high-performance fluorophore to design a fluorescent probe for HOCl detection for the first time (Scheme 1). Malononitrile was conjugated to this fluorophore as the recognition moiety towards HOCl.35,36 As a strong electron-withdrawing group, the malononitrile moiety could also increase the absorption wavelength and fluorescence emission greatly, due to which the probe would have lower phototoxicity, better tissue penetration and light scattering resistance.37,38 The oxyalkyl chain would greatly increase the aqueous solubility of the probe. And piperazine, a well-known pH-sensitive moiety, could make the probe emit different fluorescent signals under neutral or weak acidic conditions via the protonation process.39–42
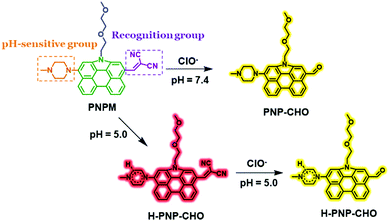 |
| Scheme 1 The structure of probe PNPM and the ClO− sensing mechanism. | |
Results and discussion
Design and synthesis of PNPM
The PNPM probe was prepared according to the synthetic route shown in Scheme 2. Compound 1 was obtained according to the previous literature.43,44 The oxyalkyl chain was attached to the nitrogen atom of compound 1 in the presence of NaH to improve the aqueous solubility of the probe. Then an aldehyde group was introduced via the Vilsmeier–Haack reaction. After introducing the bromine atom with NBS, methyl piperazine could be linked to the fluorophore as the pH-sensitive group. Finally, malononitrile which could serve as the recognition group towards HOCl was connected to the NP fluorophore according to the Knoevenagel reaction to yield the target product PNPM. All of the structures of the new compounds were already determined by 1H NMR, 13C NMR, and HRMS, and are shown in the ESI.†
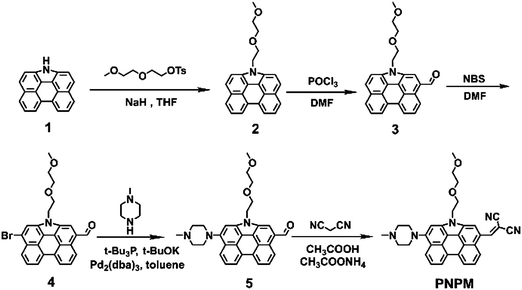 |
| Scheme 2 Synthetic route for probe PNPM. | |
Photophysical properties of probe PNPM
With probe PNPM in hand, the photophysical properties were studied by measuring the absorption and fluorescence responses towards HOCl in DMSO-PBS buffer solution (DMSO
:
PBS = 1
:
9, v/v) at 37 °C under different pH conditions. And NaClO was selected as the main source of HOCl. As shown in Fig. 1, there was almost no fluorescence under neutral conditions. But with the decrease of the pH, red fluorescence emission at 650 nm was observed due to the protonation of the piperazine moiety, causing the blocking of the photo-induced electron transfer (PET) process. Under weakly acidic conditions (pH = 5.0), the red fluorescence of the probe decreased gradually with the addition of HOCl, and the yellow fluorescence at approximately 545 nm meanwhile increased (Fig. 2A), which could be attributed to the weakening of the electron-withdrawing ability after oxidation of the malononitrile group. And the hypsochromic shift of the maximum absorption wavelength could also explain the decrease of the intramolecular charge transfer (ICT) process. The ratiometric fluorescence intensity changes (I545
nm/I650
nm), which could help the probe have a better signal-to-noise ratio (SNR) and lower system error, showed a good linear relationship with the low concentration of HOCl (Fig. 2B), and the limit of detection (LOD) was calculated to be 15.3 nM. Surprisingly, PNPM became a typical turn-on fluorescent probe in a neutral or weakly basic environment. The solution of the probe was non-fluorescent in itself. But a strong yellow fluorescence emission at about 535 nm could be observed with the addition of HOCl (Fig. 2C), which could also be seen with the naked eye. And a good linear relationship could also be observed between the relative fluorescence intensity at 535 nm and the low-concentration of HOCl (Fig. 2D). The limit of detection under neutral conditions was calculated as 31.7 nM, not as good as that in the weakly acidic environment, where PNPM would be regarded as a ratiometric fluorescent probe. And probe PNPM was pretty sensitive towards HOCl and all the chemical reactions could complete in less than one minute.
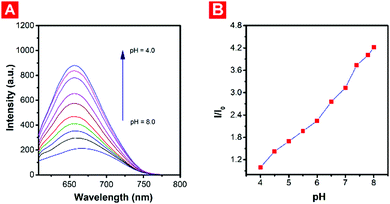 |
| Fig. 1 (A) Fluorescence emission spectra of PNPM (10 μM) at different pH values; λex = 480 nm. (B) Plotting of the changes in fluorescence intensity at 650 nm (I/I0) as a function of pH for the probe PNPM. I0 represents the initial intensity at 650 nm. | |
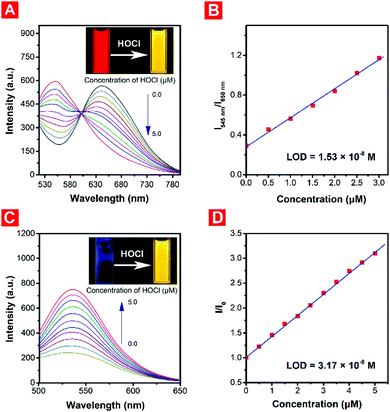 |
| Fig. 2 (A) Concentration-dependent fluorescence responses towards different concentrations of HOCl (0–5.0 μM) at pH 5.0 (λex = 480 nm). Inset: Photograph of PNPM before and after reaction with HOCl under a UV lamp (365 nm) at pH 5.0. (B) Linear correction between the ratiometric fluorescence (I545 nm/I650 nm) towards different concentrations of HOCl. (C) Concentration-dependent fluorescence responses towards different concentrations of HOCl (0–5.0 μM) at pH 7.4 (λex = 480 nm). Inset: Photograph of PNPM before and after reaction with HOCl under a UV lamp (365 nm) at pH 7.4. (D) Linear correction between the fluorescence intensity at 535 nm (I/I0) towards different concentrations of HOCl. I0 represents the initial intensity at 535 nm. | |
Selectivity and stability
To study the selectivity of the probe, different biomolecules (ATP, ADP, GSH, Cys, Fe3+, Zn2+, Cu2+) and ROS (H2O2, t-BuOOH, ONOO−, O2−, ˙OH) were used to study whether the existence of these analytes would affect the detection of HOCl when applied in complex environments (Fig. 3A and Fig. S2, ESI†). Just as expected, compared with HOCl, no obvious fluorescence changes were observed when probe PNPM was incubated with these biological molecules under weakly acidic or neutral conditions. Although the biothiols with strong nucleophilic ability might cause a few unfavorable effects, the reaction rate and fluorescence changes were much lower than those with HOCl even if their concentration was much higher, indicating the good selectivity of the probe.
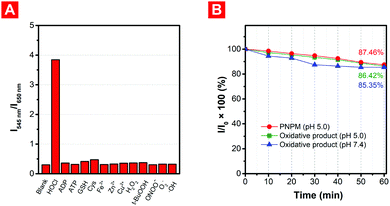 |
| Fig. 3 (A) The selectivity of probe PNPM (10 μM) towards HOCl (5 μM) at pH 5.0 in the presence of a variety of different biomolecules (ATP, ADP, GSH, Cys, Fe3+, Zn2+, Cu2+, 1 mM) and ROS (H2O2, t-BuOOH, ONOO−, O2−, ˙OH). (B) The photostability of PNPM (10 μM) and its oxidative product at different pH values was studied under 200 W m−2 light irradiation for 0–60 minutes. I0 was the initial fluorescence intensity and I was the fluorescence intensity of the sample after light irradiation at certain time intervals. | |
Photostability is another important indicator for fluorescent probes. The fluorescence of PNPM and its oxidized product is kept stable when exposed to strong light irradiation for a long time (Fig. 3B and Fig. S3, ESI†). The intensity decreased by no more than 18% during exposure to xenon lamps, indicating that strong irradiation would not destroy the structure of the probe and affect the fluorescence emission before or after the reaction with HOCl at different pH values. The excellent selectivity and photostability of the probe showed its feasibility in practical applications.
Proposed detection mechanism
To investigate the reaction mechanism of PNPM towards HClO, 1H NMR titrations were performed. As shown in the spectra (Fig. 4), the peak at 9.65 ppm, which is ascribed to the hydrogen atom on the olefin, decreased after the addition of HOCl and a peak at 10.41 ppm was captured due to the production of a formyl group (Scheme 1). And the chemical shifts of the hydrogen atoms on the benzene rings also moved to the high field slightly due to the decline of the electron-withdrawing ability. These phenomena could provide a good proof for the oxidation reaction. In another experiment, all the peaks belonging to methyl piperazine moved to the low field in the acidic solutions, showing that the protonation process of the nitrogen atom actually happened and affected the fluorescence emission of the probe.
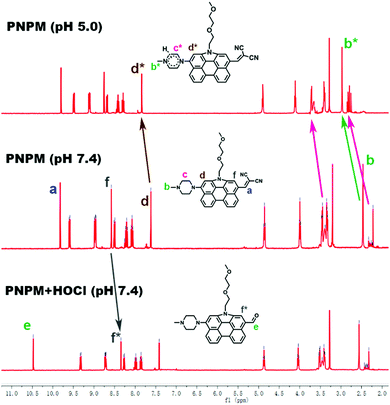 |
| Fig. 4 The 1H NMR titration spectroscopy of PNPM. | |
Practical applications in real samples
As an innovative multifunctional fluorescent probe with excellent properties, PNPM was employed to detect and quantify HOCl under various real samples, including real fetal bovine serum and environmental conditions. Due to strong oxidizing properties, HOCl is not only an important ROS in a biological environment, but also regarded as the main component of many commercial disinfectants, which are widely employed in the purification of tap water and swimming pools. Hypochlorous acid has been investigated as a possible wound care agent as well, and the U.S. Food and Drug Administration (FDA) has approved products whose main active ingredient is HOCl for use in treating wounds and various infections in humans and pets.45 But excessive levels of HOCl in them would cause negative effects on environmental protection and human health. Therefore, it is of great significance to realize the accurate quantification of HOCl with the fluorescent probe PNPM, which had outstanding sensitivity and simple operation.
Considering this, PNPM was firstly employed for the rapid detection of HOCl in real fetal bovine serum. The fluorescence responses of the probe towards low concentrations of HOCl were firstly measured in diluted fetal bovine serum. Actually, the serum solution of mammals is usually neutral or weakly basic, where PNPM is a classical turn-on fluorescent probe. So there was no doubt that an excellent linear relationship was displayed between the fluorescence intensity at 535 nm and the HOCl concentration (0–9.0 μM), showing its ability for quantitative detection in the fetal bovine serum solution without the interference of biological disrupters (Fig. 5A). To perform the recovery experiments, the diluted fetal bovine serum was firstly spiked with different concentrations of HClO (1.7, 2.3, 3.8, 4.7, 6.2 and 8.1 μM), followed by the addition of probe PNPM (10 μM). The recovered HOCl concentration was measured according to the concentration plot (Fig. 5B). As shown in Table 1, excellent recovery values and small standard deviation was observed, indicating the outstanding applicability of probe PNPM for quantifying HOCl in real serum.
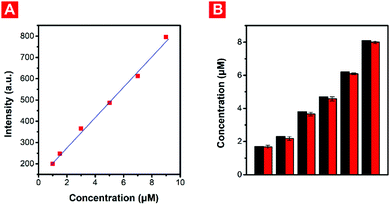 |
| Fig. 5 (A) Plotting the fluorescence intensity at 535 nm as a function of low HOCl concentration (0–9 μM) for PNPM (10 μM) in diluted serum solution. (B) The spiked (1.7, 2.3, 3.8, 4.7, 6.2 and 8.1 μM) and measured concentrations (1.68, 2.17, 3.66, 4.58, 6.09 and 8.00 μM) of HOCl in diluted serum solution. | |
Table 1 Results of the HOCl determination experiments performed in real samples
Spiked (μM) |
Fetal bovine serum |
Drinking water |
Pool water |
Measured (μM) |
Recovery value (%) |
Measured (μM) |
Recovery value (%) |
Measured (μM) |
Recovery value (%) |
1.7 |
1.68 |
98.8 |
1.61 |
94.7 |
1.62 |
95.3 |
2.3 |
2.17 |
94.3 |
2.08 |
91.4 |
2.20 |
95.7 |
3.8 |
3.66 |
96.3 |
3.66 |
96.3 |
3.70 |
97.4 |
4.7 |
4.58 |
97.4 |
4.61 |
98.1 |
4.66 |
99.1 |
6.2 |
6.09 |
98.2 |
6.19 |
99.8 |
6.31 |
101.8 |
8.1 |
8.00 |
98.8 |
8.29 |
102.3 |
7.96 |
98.3 |
Average recovery value (%) |
97.3 |
97.0 |
97.9 |
Standard deviation |
1.7 |
3.9 |
2.4 |
Differing from serum, the pH values of water samples were more complex. Drinking water might have a neutral pH, however, the pH of untreated tap water or pool water might be weakly acidic due to the existence of various decolorizers and disinfectors. And it is inspiring that probe PNPM could monitor the pH and quantify the HOCl concentration at the same time. All the water samples were collected and sampled from the campus of East China University of Science and Technology. The pH values of the water samples could be confirmed firstly according to the functional relationship between the relative fluorescence intensity at 650 nm of probe PNPM and the pH values (Fig. 1B). After reacting with HOCl for several minutes, the concentrations of HOCl could be measured according to the fluorescence responses of PNPM at different pH values. For example, in the drinking water sample, the pH of which was measured as 7.4 in this way, PNPM showed turn-on fluorescence responses. So the HOCl level in drinking water could be measured according to the standard linear relationship between the fluorescence intensity at 535 nm and the concentration of HOCl at pH 7.4 (Fig. S4A, ESI†). In the recovery experiment, the drinking water samples were spiked with different concentrations of HOCl (1.7, 2.3, 3.8, 4.7, 6.2 and 8.1 μM), followed by the addition of PNPM (10 μM). Then the recovered HOCl concentrations (1.61, 2.08, 3.66, 4.61, 6.19 and 8.29 μM) were determined with the standard linear plot (Fig. S4A and B, ESI†). Then the HOCl concentration of the pool water samples, whose pH value was measured as 6.3, was also measured by similar means. Ratiometric fluorescence changes were displayed under the weakly acidic conditions. And the recovered concentrations (1.62, 2.20, 3.70, 4.66, 6.31 and 7.96 μM) were determined according to the standard linear plot between the ratiometric fluorescence changes and the HOCl concentration at pH 6.3 (Fig. S4C and D, ESI†). Excellent recovery values of PNPM in these water samples with different pH values could be observed (Table 1). It was found that the average recovery values and standard deviations were a little better in pool water samples due to the favorable ratiometric fluorescence signals under weakly acidic conditions. The excellent results of the HOCl determination experiments performed in real serum and water samples showed the huge utilization potentiality of the probe.
Conclusions
In summary, N-annulated perylene (NP) was chosen as the fluorophore to design a fluorescent probe via relatively simple synthesis routes for the sensitive and selective detection of HOCl for the first time considering its excellent photoproperties and multiple reactive sites. A malononitrile moiety was introduced as the recognition group towards HOCl and electron-withdrawing group. Benefiting from the introduction of the piperazine moiety, the probe became pH-sensitive because of the PET process. In a neutral environment, probe PNPM was non-fluorescent in itself, but strong yellow fluorescence emission at 535 nm appeared with the addition of HOCl. And the LOD was calculated to be 31.7 nm. However, the probe had strong red fluorescence at approximately 650 nm under weakly acidic conditions. And the fluorescence would turn to yellow gradually with increasing HOCl concentration. The favorable ratiometric fluorescence changes were advantageous to improve the antijamming capability and decrease the LOD (15.3 nM). This amazing phenomenon showed that PNPM possessed the ability to monitor HOCl concentration and pH value at the same time. And excellent results of the HOCl determination experiments performed in real serum and various water samples with different pH values showed the huge utilization potentiality of the probe, providing a significant method for the design of multifunctional fluorescent probes.
Experimental section
Materials
N,N-Dimethylformamide (DMF) was refluxed with calcium hydride and distilled before use. Tetrahydrofuran (THF) was pre-dried over 4 Å molecular sieves and distilled under an argon atmosphere from sodium benzophenone ketyl immediately prior to use. Chloroform and dichloromethane (DCM) were refluxed with calcium hydride and distilled before use. All the other reagents were purchased from Sigma-Aldrich and used as received. The solutions for analytical studies were prepared with deionized water treated using a Milli-Q System (Billerica, MA, USA).
Instruments
1H and 13C NMR spectra were recorded on a Bruker AM-400 spectrometer using chloroform-d (CDCl3) as a solvent and tetramethylsilane (δ = 0) as an internal reference. Electrospray ionization and time-of-flight analyzer (ESI-TOF) mass spectra were determined using a Waters Micromass LCT mass spectrometer. Absorption spectra were recorded on a Varian Cary 500 UV-vis spectrophotometer. Fluorescence spectra were measured on a Varian Cary Eclipse spectrometer.
Synthesis of compound 2
A mixture of compound 1 (1.0 g, 3.77 mmol), 2-(2-methoxyethoxy)ethyl 4-methylbenzenesulfonate (4.14 g, 15.08 mmol), and NaH (362 mg, 15.08 mmol) was dissolved in 20 mL THF under an argon atmosphere. The reaction solution was stirred at 70 °C overnight. After being cooled to room temperature, the reaction mixture was extracted by dichloromethane and water (3 × 15 mL). The combined organic layers were washed with water and dried over anhydrous Na2SO4. After the removal of the solvent, the crude product was purified by column chromatography on silica gel using petroleum ether/DCM (1/1, v/v) as an eluent to afford compound 3 (752 mg, 54.3% yield) as a yellow solid. 1H NMR (400 MHz, CDCl3) δ: 8.62 (d, J = 8.0 Hz, 2H), 8.10 (d, J = 8.0 Hz, 2H), 7.89 (d, J = 8.0 Hz, 2H), 7.83–7.77 (m, 4H), 7.83–7.74 (m, 4H), 4.86 (t, J = 7.5 Hz, 2H), 4.02 (t, J = 7.5 Hz, 2H), 3.47–3.45 (m, 2H), 3.38–3.36 (m, 2H), 3.28 (s, 3H). 13C NMR (100 MHz, CDCl3) δ: 132.12, 130.39, 128.86, 125.00, 124.80, 124.56, 123.62, 120.66, 117.53, 113.56, 71.96, 71.07, 70.85, 59.05, 45.95. HRMS (ESI) (m/z): [M + H]+ calcd for C25H22NO2: 368.1651, found: 368.1959.
Synthesis of compound 3
Compound 2 (500 mg, 1.36 mmol) was dissolved in 15 mL of DMF. Then phosphorus oxychloride (1 mL) was added dropwise in an ice bath under an argon atmosphere. Then the reaction solution was stirred at 70 °C overnight. After being cooled down to room temperature, the mixture was poured into ice water and stirred until the ice melted. With the addition of a sodium hydroxide solution to adjust the pH value to about 8.0, a large amount of orange solid precipitated from the solution. After filtration, the crude product was purified by silica gel chromatography and eluted with dichloromethane/ethyl acetate (20
:
1, v/v) to afford compound 3 as an orange powdered solid (450 mg, 83.6%). 1H NMR (400 MHz, CDCl3) δ: 10.44 (s, 1H), 9.27 (d, J = 8.0 Hz, 1H), 8.67 (d, J = 8.0 Hz, 2H), 8.25 (s, 1H),8.13 (d, J = 8.0 Hz, 1H), 8.00–7.93 (m, 2H), 7.84 (t, J = 6.0 Hz, 1H), 7.76 (d, J = 8.0 Hz, 1H), 4.79 (t, J = 8.0 Hz, 2H), 3.99 (t, J = 4.0 Hz, 2H), 3.49–3.46 (m, 2H), 3.37–3.25 (m, 2H), 3.25 (s, 3H). 13C NMR (100 MHz, CDCl3) δ: 192.76, 135.02, 130.04, 128.60, 127.01, 125.59, 124.99, 121.82, 121.18, 113.03, 71.88, 70.30, 67.07, 66.84, 33.27, 20.38. HRMS (ESI) (m/z): [M + H]+ calcd for C26H22NO3: 396.1600, found: 396.1625.
Synthesis of compound 4
A mixture of compound 3 (300 mg, 0.76 mmol) and 1-bromopyrrolidine-2,5-dione (270 mg, 1.52 mmol) was dissolved in 20 mL of DMF under the protection of an argon atmosphere. After stirring at 50 °C overnight and cooling down to room temperature, the mixture was poured into water. A large amount of orange solid precipitated from the solution and the crude product was obtained after filtration. And the crude product was applied for the next reaction.
Synthesis of compound 5
The mixture of compound 4 (400 mg, 0.84 mmol), 1-methylpiperazine (170 mg, 1.69 mmol) and potassium tert-butoxide (378 mg, 3.37 mmol) was dissolved in 20 mL of toluene under an argon atmosphere. After stirring at 50 °C for 20 min, tri-tert-butylphosphane (0.5 mL) was added to the solution. Then the reaction temperature was adjusted to 120 °C. 12 hours later, the fluorescence of the solution changed from blue to yellow. After being cooled to room temperature, the reaction mixture was extracted by dichloromethane and water (3 × 15 mL). The combined organic layers were washed with water and dried over anhydrous Na2SO4. After the removal of the solvent, the crude product was purified by column chromatography on silica gel using ethyl alcohol/DCM (1/20, v/v) to afford compound 5 (154 mg, 37.0%) as an orange solid with yellow fluorescence. 1H NMR (400 MHz, CDCl3) δ: 10.47 (s, 1H), 9.32 (d, J = 8.0 Hz, 1H), 8.74–8.71 (m, 2H), 8.34 (s, 1H), 8.27 (d, J = 8.0 Hz, 1H), 8.01–7.97 (m, 1H), 7.86 (t, J = 8.0 Hz, 1H), 7.42 (s, 1H), 4.87 (t, J = 4.0 Hz, 2H), 4.04 (t, J = 6.0 Hz, 2H), 3.51–3.41 (m, 10H), 3.28 (s, 3H), 2.56 (s, 3H), 2.43–2.32 (m, 2H). 13C NMR (100 MHz, CDCl3) δ: 194.35, 146.72, 142.29, 135.38, 131.48, 131.08, 129.13, 127.52, 126.65, 126.30, 125.32, 124.52, 124.03, 122.97, 122.93, 116.63, 116.19, 114.35, 97.96, 73.68, 71.48, 69.54, 57.85, 52.65, 50.97, 46.06, 44.57. HRMS (ESI) (m/z): [M + H]+ calcd for C31H32N3O3: 494.2444, found: 494.2401.
Synthesis of probe PNPM
A mixture of compound 5 (200 mg, 0.45 mmol), malononitrile (54 mg, 0.81 mmol) and ammonium acetate (125 mg, 1.62 mmol) was dissolved in 20 mL of acetic acid. After refluxing for 12 hours under the protection of argon, the reaction mixture was extracted by dichloromethane and water (3 × 15 mL). The combined organic layers were washed with water. After the removal of the solvent, the crude product was purified by column chromatography on silica gel using ethyl alcohol/DCM (1/40, v/v) to afford compound PNPM (110 mg, 50.1%) as a red solid. 1H NMR (400 MHz, CDCl3) δ: 9.66 (s, 1H), 9.44 (d, J = 8.0 Hz, 1H), 8.86–8.83 (m, 2H), 8.46 (s, 1H), 8.39 (d, J = 8.0 Hz, 1H), 8.15–8.09 (m, 1H), 7.98 (t, J = 8.0 Hz, 1H), 7.54 (d, J = 8.0 Hz, 1H), 7.54 (s, 1H), 4.87 (t, J = 4.0 Hz, 2H), 4.04 (t, J = 6.0 Hz, 2H), 3.51–3.41 (m, 10H), 3.28 (s, 3H), 2.56 (s, 3H), 2.43–2.32 (m, 2H). 13C NMR (100 MHz, CDCl3) δ: 163.06, 133.66, 133.61, 127.90, 126.13, 125.55, 125.20, 122.60, 119.14, 118.86, 118.77, 118.37, 112.36, 100.54, 89.37, 76.26, 74.06, 72.12, 60.43, 55.23, 53.55, 48.64, 47.15. HRMS (ESI) (m/z): [M + H]+ calcd for C34H32N5O3: 542.2556, found: 542.2537.
Detection of HOCl levels in fetal bovine serum
For the quantification measurements, fetal bovine serum (FBS) was firstly dealt with saturated ammonium sulfate to remove the protein by the salting out effect. The probe PNPM (10 μM) was firstly added to the diluted serum (40%, FBS/PBS buffer) solution and then reacted with various concentrations of additional HOCl (1.0, 1.5, 3.0, 5.0, 7.0 and 9.0 μM) for 10 min at room temperature to obtain the linear relationship between the fluorescence intensity at 535 nm and HOCl concentration. In order to perform the recovery detection, different concentrations of HOCl (1.7, 2.3, 3.8, 4.7, 6.2 and 8.1 μM) were spiked into the solution, followed by addition of the probes (10 μM) and reaction for 10 min. The fluorescence emissions of the solution were finally determined by using a spectrometer.
Conflicts of interest
There are no conflicts to declare.
Acknowledgements
We greatly appreciate the National Basic Research 973 Program (2103CB733700), NSFC/China (21421004, 21372082, 21772040 and 21572062), the Fundamental Research Funds for the Central Universities and the Programme of Introducing Talents of Discipline to Universities (B16017) for their financial support of this research.
Notes and references
- M. Hayyan, M. A. Hashim and I. M. AlNashef, Chem. Rev., 2016, 116, 3029–3085 CrossRef CAS PubMed.
- L. Yuan, L. Wang, B. K. Agrawalla, S. J. Park, H. Zhu, B. Sivaraman, J. Peng, Q. Q. H. Xu and Y. T. Chang, J. Am. Chem. Soc., 2015, 137, 5930–5938 CrossRef CAS PubMed.
- H. Xiao, J. Li, J. Zhao, G. Yin, Y. Quan, J. Wang and R. Wang, J. Mater. Chem. B, 2015, 3, 1633–1638 RSC.
- M. Whiteman, P. Rose, J. L. Siau, N. S. Cheung, G. S. Tan, B. Halliwell and J. S. Armstrong, Free Radical Biol. Med., 2005, 38, 1571–1584 CrossRef CAS PubMed.
- L. Liang, C. Liu, X. Jiao, L. Zhao and X. Zeng, Chem. Commun., 2016, 52, 7982–7985 RSC.
- Y. Yue, F. Huo, C. Yin, J. O. Escobedo and R. M. Strongin, Analyst, 2016, 141, 1859–1873 RSC.
- J. J. Hu, N. K. Wong, M. Y. Lu, X. Chen, S. Ye, A. Q. Zhao, P. Gao, R. Y. T. Kao, J. Shen and D. Yang, Chem. Sci., 2016, 7, 2094–2099 RSC.
- J. T. Hou, K. Li, J. Yang, K. K. Yu, Y. X. Liao, Y. Z. Ran, Y. H. Liu, X. D. Zhou and X. Q. Yu, Chem. Commun., 2015, 51, 6781–6784 RSC.
- X. Jin, Y. Jia, W. Chen, P. Chui and Z. Yang, Sens. Actuators, B, 2016, 232, 300–305 CrossRef CAS.
- X. Zhang, Y. Hang, W. Qu, Y. Yan, P. Zhao and J. Hua, RSC Adv., 2016, 6, 20014–20020 RSC.
- H. Xiao, K. Xin, H. Dou, G. Yin, Y. Quan and R. Wang, Chem. Commun., 2015, 51, 1442–1445 RSC.
- P. Xing, K. Gao, B. Wang, J. Gao, H. Yan, J. Wen, W. Li, Y. Xu, H. Li, J. Chen, W. Wang and S. Sun, Chem. Commun., 2016, 52, 5064–5066 RSC.
- Y. R. Zhang, X. P. Chen, J. Shao, J. Y. Zhang, Q. Yuan, J. Y. Miao and B. X. Zhao, Chem. Commun., 2014, 50, 14241–14244 CAS.
- W. Yin, H. Zhu and R. Wang, Dyes Pigm., 2014, 107, 127–132 CrossRef CAS.
- S. L. Shen, J. Y. Ning, X. F. Zhang, J. Y. Miao and B. X. Zhao, Sens. Actuators, B, 2017, 244, 907–913 CrossRef CAS.
- X. Chen, F. Wang, J. Y. Hyun, T. Wei, J. Qiang, X. Ren, I. Shin and J. Yoon, Chem. Soc. Rev., 2016, 45, 2976–3016 RSC.
- Q. Xu, C. H. Heo, G. Kim, H. W. Lee, H. M. Kim and J. Yoon, Angew. Chem., Int. Ed., 2015, 54, 4890–4894 CrossRef CAS PubMed.
- J. Zhou, L. Li, W. Shi, X. Gao, X. Li and H. Ma, Chem. Sci., 2015, 6, 4884–4888 RSC.
- X. Chen, K. A. Lee, X. Ren, J. C. Ryu, G. Kim, J. H. Ryu, W. J. Lee and J. Yoon, Nat. Protoc., 2016, 11, 1219–1228 CrossRef CAS PubMed.
- Q. Xu, K. A. Lee, S. Lee, K. M. Lee, W. J. Lee and J. Yoon, J. Am. Chem. Soc., 2013, 135, 9944–9949 CrossRef CAS PubMed.
- Y. Koide, Y. Urano, K. Hanaoka, T. Terai and T. Nagano, J. Am. Chem. Soc., 2011, 133, 5680–5682 CrossRef CAS PubMed.
- P. Montcourrier, P. H. Mangeat, C. Valembois, G. Salazar, A. Sahuquet, C. Duperray and H. Rochefort, J. Cell Sci., 1994, 107, 2381–2391 Search PubMed.
- W. C. Chen, P. Venkatesan and S. P. Wu, Anal. Chim. Acta, 2015, 882, 68–75 CrossRef CAS PubMed.
- G. F. Wu, F. Zeng and S. Z. Wu, Anal. Methods, 2013, 5, 5589–5596 RSC.
- J. J. Hu, N. K. Wong, Q. S. Gu, X. Y. Bai, S. Ye and D. Yang, Org. Lett., 2014, 16, 3544–3547 CrossRef CAS PubMed.
- T. Guo, L. Cui, J. N. Shen, R. Wang, W. P. Zhu, Y. F. Xu and X. H. Qian, Chem. Commun., 2013, 49, 1862–1864 RSC.
- Z. R. Lou, P. Li, Q. Pan and K. L. Han, Chem. Commun., 2013, 49, 2445–2447 RSC.
- Y. K. Yang, H. J. Cho, J. Lee, I. Shin and J. Tae, Org. Lett., 2009, 11, 859–861 CrossRef CAS PubMed.
- X. Q. Chen, X. C. Wang, S. J. Wang, W. Shi, K. Wang and H. M. Ma, Chem. – Eur. J., 2008, 14, 4719–4724 CrossRef CAS PubMed.
- G. P. Li, D. J. Zhu, Q. Liu, L. Xue and H. Jiang, Org. Lett., 2013, 15, 2002–2005 CrossRef CAS PubMed.
- J. O. Moon, J. W. Lee, M. G. Choi, S. Ahn and S. K. Chang, Tetrahedron Lett., 2012, 53, 6594–6659 CrossRef CAS.
- X. Zhang, H. Tan, Y. Yan, Y. Hang, F. Yu, X. Qu and J. Hua, J. Mater. Chem. B, 2017, 5, 2172–2180 RSC.
- J. Luo, M. F. Xu, R. Z. Li, K. W. Huang, C. Y. Jiang, Q. B. Qi, W. D. Zeng, J. Zhang, C. Y. Chi, P. Wang and J. S. Wu, J. Am. Chem. Soc., 2014, 136, 265–272 CrossRef CAS PubMed.
- W. Jiang, H. L. Qian, Y. Li and Z. H. Wang, J. Org. Chem., 2008, 73, 7369–7372 CrossRef CAS PubMed.
- J. Park, H. Kim, Y. Choi and Y. Kim, Analyst, 2013, 138, 3368–3371 RSC.
- B. C. Zhu, Y. H. Xu, W. Q. Liu, C. X. Shao, H. F. Wua, H. L. Jiang, B. Dua and X. L. Zhang, Sens. Actuators, B, 2014, 191, 473–478 CrossRef CAS.
- M. H. Lee, J. S. Kim and J. L. Sessler, Chem. Soc. Rev., 2015, 44, 4185–4191 RSC.
- T. D. Ashton, K. A. Jolliffe and F. M. Pfeffer, Chem. Soc. Rev., 2015, 44, 4547–4595 RSC.
- D. Asanuma, Y. Takaoka, S. Namiki, K. Takikawa, M. Kamiya, T. Nagano, Y. Urano and K. Hirose, Angew. Chem., Int. Ed., 2014, 53, 6085–6089 CrossRef CAS PubMed.
- M. H. Lee, N. Park, C. Yi, J. H. Han, J. H. Hong, K. P. Kim, D. H. Kang, J. L. Sessler, C. Kang and J. S. Kim, J. Am. Chem. Soc., 2014, 136, 14136–14141 CrossRef CAS PubMed.
- T. Myochin, K. Kiyose, K. Hanaoka, H. Kojima, T. Terai and T. Nagano, J. Am. Chem. Soc., 2011, 133, 3401–3409 CrossRef CAS PubMed.
- L. Huang and S. W. T. Chang, Chem. Commun., 2011, 47, 2291–2293 RSC.
- W. Jiang, H. Qian, Y. Li and Z. Wang, J. Org. Chem., 2008, 73, 7369–7372 CrossRef CAS PubMed.
- Z. Yao, H. Wu, Y. Ren, Y. Guo and P. Wang, Energy Environ. Sci., 2015, 8, 1438–1442 CAS.
- J. B. Selkon, G. W. Cherry, J. M. Wilson and M. A. Hughes, J. Wound Care, 2006, 15, 33–37 CrossRef CAS PubMed.
Footnote |
† Electronic supplementary information (ESI) available. See DOI: 10.1039/c7qm00298j |
|
This journal is © the Partner Organisations 2017 |
Click here to see how this site uses Cookies. View our privacy policy here.