DOI:
10.1039/C5SC03591K
(Edge Article)
Chem. Sci., 2016,
7, 1896-1903
Single near-infrared fluorescent probe with high- and low-sensitivity sites for sensing different concentration ranges of biological thiols with distinct modes of fluorescence signals†
Received
22nd September 2015
, Accepted 24th November 2015
First published on 24th November 2015
Abstract
We describe a unique approach for the development of an interesting type of the fluorescent probes, which can show different modes of fluorescence signals to distinct concentration ranges of a target of interest. The key points for the design of the new type of the fluorescent probes include the judicious selection of the dye platforms and the corresponding high- and low-sensitivity sites. It is known that the normal concentrations of biological thiols have significant biological functions. However, up- or down-regulated concentrations of thiols may induce several diseases. Therefore, it is highly important to monitor the changes of thiol concentrations in living systems. Based on the proposed strategy, we engineer the novel NIR fluorescent probe, CHMC-thiol, which remarkably can display a turn-on signal to the low concentration range of thiols and a ratiometric response to the high concentration range of thiols for the first time. We anticipate that the intriguing strategy formulated herein will be widely useful for the development of concentration range-dependent fluorescent probes.
Introduction
Small-molecular-weight biological thiols, including cysteine (Cys), homocysteine (Hcy), and glutathione (GSH), play a critical role in many biological processes. However, abnormal concentrations of Cys, Hcy and GSH are implicated in a variety of diseases, such as liver damage, skin lesions, slowed growth and edema.1–3 Therefore, it is highly important to monitor the changes of concentrations of thiols in living systems.
Although a large amount of fluorescent probes have been developed,4–7 among them only a few single fluorescent probes are capable of monitoring multiple targets in living systems with distinct fluorescence signals.8 A single fluorescent probe with the ability of sensing multiple targets in a consecutive manner imparts several advantages over the combination of several fluorescent probes, as it can avoid the problems such as cross-talk, different localizations, and different metabolisms induced by the combination use of several fluorescent probes. Nevertheless, to the best our knowledge, there is no report yet describing a single fluorescent thiol probe for sensing the different concentration ranges of thiols with distinct fluorescence signals. Thus, it is interesting to develop a probe exhibiting different modes of fluorescence signal for the different concentration ranges of thiols. Obviously, it is very challenging to design such intriguing concentration range-dependent fluorescent probes.
Aware of the great interest for the development of concentration range-dependent fluorescent probes, in particular with near-infrared (NIR) emission properties for potential applications in living animals, several factors should be considered in designing this type of probe. First, the NIR fluorescent dye should have two chemical handles, which can be readily modified to afford two interaction sites for the target of interest. Secondly, the two interaction sites should exhibit distinct sensitivity to the target of interest. This may provide the recognition basis (the interaction step in the overall sensing process). In other words, the probes should contain high and low sensitivity sites for the target of interest. Thirdly, mostly important and challenging, the high-sensitivity site of the probes should interact with the low concentration range of the target to provide one mode of fluorescence signal, while the low-sensitivity site of the probes should interact with the high concentration range of the target to give off the other mode of fluorescence signal. This involves the transduction of the recognition into the optical signal.
In 1992, Patonay et al. developed heptamethine cyanine dyes with a rigid chlorocyclohexenyl ring in the methine chain, which is favorable to increase the photostability and fluorescence quantum yields.9 The rigid structures also render the chloro group as a reactive site for chemical substitution at the central ring. Recently, our group has presented an approach for the regulation of the fluorescence of merocyanine dyes (HD NIR dyes).10 The dyes are advantageous over the traditional 7-hydroxycoumarin and fluorescein with maximal absorption and emission in the NIR region while retaining an optically tunable hydroxyl group. However, both the traditional chloro-substituted heptamethine cyanine dyes and the recently developed HD NIR dyes have only one chemical handle that can be transformed into a reactive site for a target of interest. To address this problem, we envisioned that by the hybridization of the chloro-substituted cyanine and HD NIR dye, we may afford an innovative type of NIR dye, CHMC (chloro-hydroxyl merocyanine), which, notably, contains a key chloro moiety and a critical hydroxyl group that can be readily transformed into two reactive sites for a target of interest (Fig. 1). We thus anticipated that the novel CHMC dye could be exploited as a powerful platform for the design of the concentration range-dependent NIR fluorescent probes for thiols.
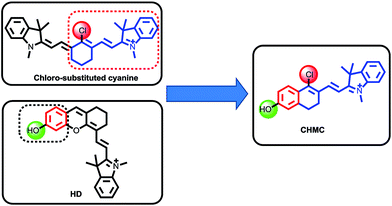 |
| Fig. 1 The design of the novel CHMC dye as a representative platform for the concentration range-dependent NIR fluorescent probes by the hybridization of the chloro-substituted cyanine and HD NIR dye. | |
After selecting the platform, the next step is to choose the two interaction sites for thiols. With the above design criteria for the innovative fluorescent probes in mind, we proposed the new CHMC-thiol (Fig. 2) as a potential candidate probe that may show two modes of fluorescence signals towards low and high concentration ranges of thiols. It is known that the 2,4-dinitrobenzenesulfonate moiety is highly reactive towards thiols,3g,11 thus, it is reasonable to be selected as the high-sensitivity site for thiols. By contrast, the chloro group is less reactive to thiols, and it was chosen as the low-sensitivity site for thiols.2c,2f,12 From the optical point of view, the 2,4-dinitrobenzenesulfonate moiety is a notorious fluorescence quencher,3g,11 thus, we envisioned that interactions of the 2,4-dinitrobenzenesulfonate moiety of the probe CHMC-thiol with thiols may provide a turn-on mode in fluorescence. On the other hand, it is known that the nucleophilic substitution of the chloro moiety of chloro-substituted cyanine with heteroatoms (i.e. sulfur,12 nitrogen9b) may induce a ratiometric mode in fluorescence. We thus anticipated that, similarly, the nucleophilic substitution of the chloro moiety of the probe CHMC-thiol with thiols may induce a ratiometric mode in fluorescence. In this way, the unique probe CHMC-thiol containing both the high-sensitivity site (the 2,4-dinitrobenzenesulfonate moiety) and the low-sensitivity site (the chloro moiety), may provide a turn-on mode and a ratiometric mode in fluorescence, respectively, upon interactions with thiols.
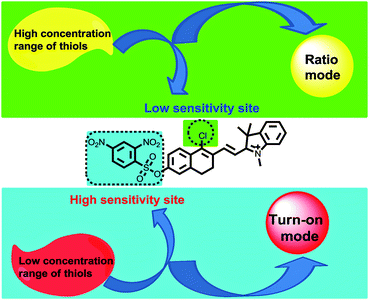 |
| Fig. 2 Rational design of the smart fluorescent thiol probe, CHMC-thiol (CHMC as a platform for CHMC-thiol; the 2,4-dinitrobenzenesulfonate moiety is the high sensitivity site to provide a turn-on mode in fluorescence, and the chloro group is the low-sensitivity site to give off a ratiometric mode in fluorescence). | |
Results and discussion
Synthesis of the fluorescent probe CHMC-thiol
The synthesis of the smart probe CHMC-thiol was accomplished in four steps as shown in Scheme 1. Starting material 1 is commercially available. The intermediate aldehyde 2 was prepared by the Vilsmeier reaction, and it was further used to synthesize the compounds CHMC1-C and CHMC2-C by the Knoevenagel condensation without purification.
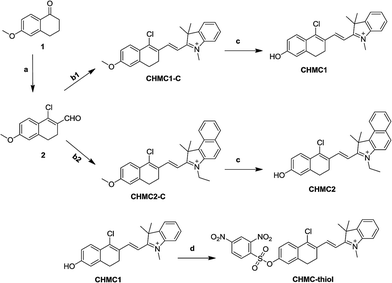 |
| Scheme 1 Synthesis of compounds CHMC-thiol and some controls CHMC1,2. Reagents and conditions: (a) DMF/POCl3, 5 h; (b1) 1-butanol–benzene (7 : 3), indolium salt, 90 °C, 5 h; (b2) 1-butanol–benzene (7 : 3), benz[e]indolium salt, 90 °C, 5 h; (c) BBr3, dry CH2Cl2, 0 °C, overnight. (d) Cs2CO3, CH2Cl2, 2,4-dinitrobenzenesulfonyl chloride, room temperature, 5 h. | |
Treatment of CHMC1-C and CHMC2-C with BBr3 in dry CH2Cl2 afforded the controls CHMC1 and CHMC2, respectively. Then, the CHMC1 dye was reacted with 2,4-dinitrobenzenesulfonyl chloride under basic conditions to provide the target compound CHMC-thiol. The detailed synthetic procedures are presented in the Experimental section. All new compounds were carefully characterized by 1H NMR, 13C NMR and HRMS (ESI†).
Photophysical properties of fluorescent dyes CHMC1,2
To support our design concept of the new fluorescent probe CHMC-thiol, we proceeded to investigate the optical properties of the CHMC dyes. The absorption and emission profiles of compounds CHMC1, CHMC2, CHMC1-C and CHMC2-C in distinct solvents (i.e. EtOH, PBS) are shown in Fig. S1 and Table S1.† First, we investigated the spectral properties of CHMC1, CHMC2, CHMC1-C and CHMC2-C dyes in the protic solvent EtOH. The absorption spectra of CHMC1 and CHMC2 (5 μM) in EtOH possess a broad absorption band due to the various vibrational and rotational states, typical of the merocyanine dyes. Notably, CHMC1 and CHMC2 have a maximum absorption peak at around 670–690 nm in EtOH, in the NIR region, with large molar absorption coefficient (>105). Fluorescence spectra of CHMC1 and CHMC2 exhibit strong fluorescence with a maximum emission peak at around 695 and 714 nm, respectively. This behavior of CHMC1 and CHMC2 NIR dyes is in good agreement with that of the classic NIR carbocyanine dyes. The compounds CHMC1-C and CHMC2-C, which bear no key hydroxyl group, display weak fluorescence. Thus, these data suggest that the optical properties of CHMC1 and CHMC2 dyes can be regulated by alkylation on the hydroxyl group.
To get more insight to the hydroxyl chemical handle in CHMC dyes, we examined the pH effect on the photophysical properties of CHMC1 and CHMC2 dyes in PBS. The standard pH titrations on the compound CHMC1 (5 μM) were performed in aqueous solution (containing 10% EtOH as a co-solvent). With the enhancement of pH values from 3.0 to 10.0, the absorption peak of CHMC1 at around 500 nm significantly diminishes, and simultaneously a new red-shifted absorption band at around 630 nm is observed (Fig. S2†). There is a well-defined isosbestic point at 543 nm in the absorption spectra, suggesting the presence of two species in equilibrium. The red-shift in the absorption spectra with increase of pH can be explained due to the deprotonation of the CHMC1 dye. The pH-dependent absorption spectra of CHMC2 (Fig. S3†) exhibit a similar trend to those of CHMC1 with the enhancement of pH values from 3.0 to 10.0.
The fluorescence emission spectrum of CHMC1 at pH 3.0 displays an emission band with a maximum at 616 nm (Fig. 3a). Deprotonation of CHMC1 increases the electron donating ability of the hydroxyl group and therefore results in a ca. 64 nm red-shift in the emission spectra from 616 to 680 nm (Fig. 3a). The pH-dependent emission spectra of CHMC2 (Fig. 3c) display a red-shift in the emission spectra from 635 to 700 nm. The analysis of emission intensity changes at 680 nm for CHMC1 and 700 nm for CHMC2 as a function of pH by using the Henderson–Hasselbalch-type mass action equation yields pKa values of 7.05 and 7.15, respectively.13 The ratios of fluorescence intensities at 680 and 616 nm (I680/I616) of CHMC1 show a dramatic change from 0.43 at pH 3.0 to 52.3 at pH 10.0 (Fig. S4†), a nearly 121-fold variation in the emission ratios. Similarly, the emission ratios of CHMC2 (I700/I635) exhibit a 258-fold change in the emission ratios (Fig. S5†).
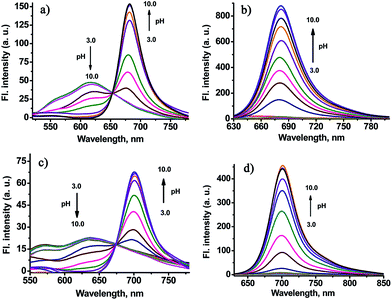 |
| Fig. 3 (a) pH-Dependence of the emission spectra of compound CHMC1 (5 μM) with the arrows indicating the change of the emission intensities with pH enhancement from 3 to 10, excitation at 480 nm; (b) pH-dependence of the emission spectra of compound CHMC1 (5 μM) with the arrows indicating the change of the emission intensities with pH enhancement from 3 to 10, excitation at 630 nm; (c) pH-dependence of the emission spectra of compound CHMC2 (5 μM) with the arrows indicating the change of the emission intensities with pH enhancement from 3 to 10, excitation at 480 nm; (d) pH-dependence of the emission spectra of compound CHMC2 (5 μM) with the arrows indicating the change of the emission intensities with pH enhancement from 3 to 10, excitation at 630 nm. | |
When excited at 630 nm, the emission band of CHMC1 centered at 680 nm rises as the pH value increases, and it shows a 45-fold fluorescence intensity enhancement (Fig. 3b and S6a†). The pH-dependent emission spectra of CHMC2 exhibits the similar trend with pH values increased from 3.0 to 10.0 (Fig. 3d and S6b†). These results indicate that the optical properties of the two-chemical-handle NIR dyes CHMC1 and CHMC2 can be controlled by modifications on the hydroxyl handle. Thus, as designed, CHMC dyes still maintain the tunable hydroxyl handle, just like the previously developed HD NIR dyes.
Sensing of CHMC1 and CHMC2 to high and low concentration ranges of thiols
Like chloro-substituted cyanine, the chloro moiety of CHMC1 and CHMC2 may also provide the dyes with a chemical handle for substitution at the central ring. For further proof of our design concept of the fluorescent probe CHMC-thiol, we must examine the possibility of CHMC1 and CHMC2 to detect thiols in PBS using the chloro group. In preliminary experiments, we first evaluated the capability of CHMC1 and CHMC2 to detect thiols (represented by Cys) in PBS in the presence of a low concentration range of Cys. As shown in Fig. S7 and S8,† addition of a low concentration range of Cys (0–35 μM) induces no marked fluorescence changes after 1 h.
To further understand the ability of CHMC1 and CHMC2 to sense Cys, these two dyes were incubated with a high concentration range of Cys (35–500 μM). As shown in Fig. 4a, upon excitation at 550 nm, the free CHMC1 shows the emission peak at 680 nm. However, upon addition of a high concentration range of Cys, the emission peak at 680 nm gradually decreases, and a new emission band at 625 nm appears. Consistently, a marked blue-shift from 643 to 541 nm is noted in the absorption spectra upon treatment of CHMC1 with Cys (Fig. S9†). When adding 100 equiv. Cys, the ratios of fluorescence intensities at 625 nm and 680 nm (I625/I680) of CHMC1 show a dramatic change from 0.26 to 7.7, a nearly 29-fold enhancement in the emission ratio. Similarly, the ratios of compound CHMC2 (I642/I700) exhibit a 47-fold change (Fig. 4b). The mass spectrometry analyses confirm that the ratiometric fluorescence changes in the emission profiles is due to the substitution reaction by the thiolate of Cys and followed by intramolecular rearrangement (Fig. S10†). The results indicate that, as for the chloro-substituted cyanine dye, the two-chemical-handle NIR dyes CHMC1 and CHMC2 still retain the chloro moiety as the effective interaction site for Cys. In other words, as designed, CHMC1 and CHMC2 can be employed to sense a high concentration range of Cys by the chloro group. Notably, the threshold of high concentration range of Cys which elicits the ratio signal in fluorescence is well within the physiological concentration range of Cys (30–200 uM).14
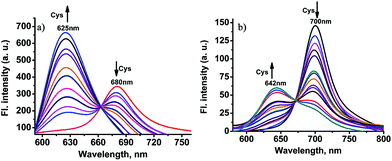 |
| Fig. 4 (a) Fluorescence spectra of CHMC1 (5 μM) in the aqueous buffer in the presence of a high concentration range of Cys (35–500 μM), excitation at 550 nm; (b) fluorescence spectra of the probe CHMC2 (5 μM) in aqueous buffer in the presence of a high concentration range of Cys (35–500 μM), excitation at 550 nm. | |
Sensing of the probe CHMC-thiol to high and low concentration ranges of thiols in PBS
In order to test the design strategy of the probe CHMC-thiol, we evaluated its capability to detect biological thiols (represented by Cys) in PBS. As designed, the free probe is almost non-fluorescent in PBS (Fig. 5a). However, addition of a low concentration range of Cys (0–50 μM) induces significant variations in the fluorescence spectra at 680 nm. In good agreement, a marked red-shift from 442 to 657 nm is present in the absorption spectra upon treatment of the probe with Cys (Fig. S11a†). By contrast, addition of a high concentration range of Cys (50–500 μM) elicits a fluorescence ratiometric response in the fluorescence spectra (Fig. 5b). The corresponding absorption spectra upon treatment of the probe with Cys are shown in Fig. S11b.†
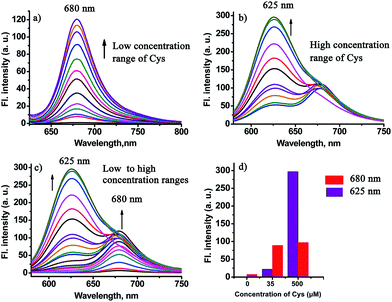 |
| Fig. 5 (a) Fluorescence spectra of the probe CHMC-thiol (5 μM) in the presence of a low concentration range of Cys (0–50 μM); (b) fluorescence spectra of the probe CHMC-thiol (5 μM) in the presence of a high concentration range of Cys (50–500 μM); (c) fluorescence spectra of the probe CHMC-thiol (5 μM) in the presence of low to high concentration ranges of Cys (0–500 μM); (d) fluorescence intensity at 680 or 625 nm of the probe CHMC-thiol (5 μM) in the presence of representative concentrations of Cys (0, 35, 500 μM). All spectra were obtained after adding the analyte for 1 h and excitation at 550 nm. | |
To further estimate the ability of the probe CHMC-thiol, we also examined the response of the probe at a different concentration, i.e. 10 μM. As shown in Fig. S12,† the results are very similar to those of the probe at 5 μM. Taken together, the studies indicate that the probe CHMC-thiol has distinct modes of fluorescence signals towards the different concentration ranges of thiols. The probe exhibits a turn-on mode of the fluorescence signal to the low concentration range of thiols at 680 nm, while displaying a ratiometric mode of the fluorescence signal to the high concentration range of thiols. Thus, the emission peak at 625 nm may be considered as an important marker for the concentration range of Cys (Fig. 5d). To the best of our knowledge, this represents the first fluorescent probe which can sense low and high concentration ranges of thiols with different modes of the fluorescence signals.
Based on the above vital findings, a possible mechanistic pathway for sensing thiols is proposed in Scheme 2. The probe CHMC-thiol has distinct response pathways towards different concentration ranges of thiols. On the one hand, upon reaction with a low concentration range of Cys, the fluorescence turn-on is due to the thiol-mediated removal of the 2,4-dinitrobenzenesulfonate moiety. Both mass spectrometry (Fig. S13†) and NMR (Fig. S14†) analyses confirm the formation of CHMC1. In addition, from the fluorescence point of view, the normalized emission of the probe CHMC-thiol reaction with the low concentration range of Cys (Fig. S15a†) highly resembles that of CHMC1 (Fig. S15b†). On the other hand, in the presence of a high concentration range of Cys, the chloro group in CHMC-thiol is initially replaced by the thiol group of Cys to produce the 4-sulfhydryl intermediate 3, and the subsequent intramolecular SNAr substitution of the sulfhydryl moiety by the amino group leads to the formation of the 4-amino product 4.2c,2f,12,15 This process is consistent with the results of mass spectrometry (Fig. S16†) and NMR analyses (Fig. S17†). To further confirm that the final product is the 4-amino product 4 instead of the 4-sulfhydryl intermediate 3, we further synthesized compounds 5 and 6 as the amino and sulfhydryl substituted controls, respectively (Scheme 2). The spectral studies indicate that the emission profile of the probe CHMC-thiol reaction with high concentration range of Cys highly resembles that of the control 5 instead of 6 (Fig. S18†). Thus, taken together, these data corroborate the sensing mechanism of CHMC-thiol in the presence of low and high concentration ranges of Cys as proposed in Scheme S2.†
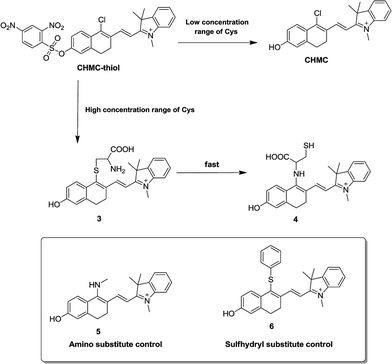 |
| Scheme 2 The proposed reaction mechanism of CHMC-thiol with biological thiols (represented by Cys) and the structures of the amino and sulfhydryl substituted controls 5 and 6. | |
We also evaluated the capability of CHMC-thiol to detect other small-molecular-weight biological thiols (Hcy and GSH). The titrations of the novel probe CHMC-thiol (5 μM) with Hcy were conducted in PBS (pH 7.4, containing 10% EtOH as a cosolvent). The probe CHMC-thiol exhibits a similar behavior towards Hcy as found for Cys. Upon reaction with a low concentration range of Hcy, the fluorescence turn-on at 680 nm is due to the thiol-mediated removal of the 2,4-dinitrobenzenesulfonate moiety. However, on addition of a high concentration range of Hcy, a ratiometric response is observed owing to nucleophilic substitution followed by an intramolecular rearrangement (Fig. S19 and Scheme S1†). Hcy is a potential interferent at certain conditions for the detection of Cys due to the structural and chemical similarity between them. In the presence of a low concentration range of GSH, the probe CHMC-thiol shows a similar trend to Cys and Hcy. However, the treatment of the probe with a high concentration range of GSH only produces the 4-sulfhydryl product due to the steric hindrance, which leads to a 10 nm redshift in the emission spectra (Fig. S20 and Scheme S2†). These results are in good agreement with those reported in the literature.2c,2f,12,15
To examine the selectivity, the probe CHMC-thiol (5 μM) was treated with various biologically relevant species (e.g., representative anions, metal ions, reactive oxygen species, reactive nitrogen species, reducing agents) in aqueous buffer. As shown in Fig. 6, addition of representative anions (Cl−, Br−, I−, AcO−, N3−, CN−, CO32−, NO2−) at 1 mM, metal ions (K+, Mg2+, Ca2+, Zn2+) at 1 mM, reactive oxygen and nitrogen species (H2O2, ClO−, NO), and reducing agents (ascorbic acid, Na2S) at biologically relevant concentrations induces no marked fluorescence enhancement at 680 nm. By contrast, upon treatment of thiols with the probe, a large fluorescence signal was observed at 680 nm (Fig. 6a). The fluorescence signals at 625 nm (Fig. 6b) are consistent with those at 680 nm (Fig. 6a). Thus, these data demonstrate that the probe CHMC-thiol has a high selectivity for thiols over other biological species tested at biologically relevant concentrations, suggesting that the probe CHMC-thiol is favorable for studies of biological thiols in biological systems.
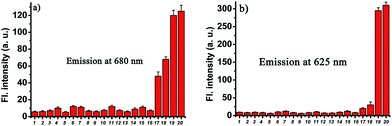 |
| Fig. 6 (a) Fluorescence responses of the probe CHMC-thiol (5 μM) to various biologically relevant species in aqueous buffer. Red bars represent the addition of an excess of the representative anions, metal ions, reactive oxygen species, reactive nitrogen species, reducing agents, small-molecule thiols. (Cl−, Br−, I−, AcO−, N3−, CN−, CO32−, NO2−) at 1 mM, metal ions (K+, Mg2+, Ca2+, Zn2+) at 1 mM, reactive oxygen and nitrogen species (H2O2, OCl−, NO, Na2S) at 100 μM. Ascorbic acid, GSH, Hcy and Cys at 1 mM. (1) Cl−, (2) Br−, (3) I−, (4) AcO−, (5) N3−, (6) CN−, (7) CO32, (8) NO2−, (9) Mg2+, (10) K+, (11) Zn2+, (12) Ca2+, (13) H2O2, (14) ClO−, (15) NO, (16) ascorbic acid, (17) Na2S, (18) GSH, (19) Hcy, (20) Cys. Excitation at 550 nm and emission at 680 nm; (b) fluorescence responses of the probe CHMC-thiol (5 μM) to various biologically relevant species in aqueous buffer. Cl−, Br−, I−, AcO−, N3−, CN−, CO32−, NO2−) at 1 mM, metal ions (K+, Mg2+, Ca2+, Zn2+) at 1 mM, reactive oxygen and nitrogen species (H2O2, OCl−, NO) at 100 μM. Ascorbic acid, GSH, Hcy and Cys at 1 mM. (1) Cl−, (2) Br−, (3) I−, (4) AcO−, (5), N3−, (6) CN−, (7) CO32, (8) NO2−, (9) Mg2+, (10) K+, (11) Zn2+, (12) Ca2+, (13) H2O2, (14) ClO−, (15) NO, (16) ascorbic acid, (17) Na2S, (18) GSH, (19) Hcy, (20) Cys. Excitation at 550 nm and emission at 625 nm. Data were expressed as mean ± SD of three experiments. | |
Kinetic studies of the probe CHMC-thiol
The time course of the probe CHMC-thiol in the presence of thiols (represented by Cys) is displayed in Fig. 7. The response of the 2,4-dinitrobenzenesulfonate moiety (the high-sensitivity site) of CHMC-thiol to Cys is fast, and the reaction is complete within 10 min at 680 nm (Fig. 7a). The pseudo-first-order rate constant of the high-sensitivity site is calculated to be k = 0.3295 min−1 for Cys at 680 nm (Fig. 7b). By contrast, the response of the chloro group (the low sensitivity site) of CHMC-thiol to Cys is slower than the 2,4-dinitrobenzenesulfonate moiety, and the reaction is complete within 60 min at 625 nm (Fig. 7c). The pseudo-first-order rate constant of the second site is calculated to be k = 0.033 min−1 for Cys at 625 nm (Fig. 7d). The pseudo-first-order rate constant of the high sensitivity site is about 10 times that of the low sensitivity site. It is important to note that such a large difference in the pseudo-first-order rate constants of the two sensing sites is highly desirable for the development of concentration range-dependent fluorescent probes.
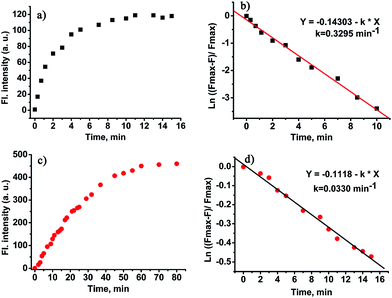 |
| Fig. 7 (a) Reaction-time profiles of CHMC-thiol (5.0 μM) in the presence of 100 μM Cys (■) at 680 nm; (b) pseudo-first-order kinetic plot of the reaction of the probe CHMC-thiol (5 μM) with Cys (100 μM) at 680 nm in pH 7.4, 25 mM PBS. Slope = 0.3295 min−1; (c) reaction-time profiles of CHMC-thiol (5.0 μM) in the presence of 500 μM Cys (■) at 625 nm; (d) pseudo-first-order kinetic plot of the reaction of the probe CHMC-thiol (5 μM) with Cys (500 μM) at 625 nm in pH 7.4, 25 mM PBS. Slope = 0.0330 min−1. | |
Imaging of the CHMC-thiol to different concentration ranges of thiols in live cells
Notably, the threshold of the low and high concentration ranges of the fluorescent probes CHMC-thiol is well within the physiological concentration range of Cys (30–200 μM).14 For preliminary fluorescence imaging applications, the probe CHMC-thiol was incubated with living HeLa cells pretreated with or without N-ethylmaleimide (as a thiol blocking agent). As shown in Fig. 8g, the cells treated only with the probe exhibit strong fluorescence in the red channel. However, when the cells are pretreated with N-ethylmaleimide, then incubated with CHMC-thiol, much weaker fluorescence in the red channel is observed (Fig. 8c). To further study the ability of the probe CHMC-thiol for imaging different concentration ranges of thiols in living cells, the cells were incubated with Cys (100 μM) for 30 min, and then treated with the probe CHMC-thiol (5 μM) for another 30 min. In such a case, the cells exhibit intense fluorescence in the orange channel (Fig. 8j). By contrast, the cells incubated with only the probe display almost no fluorescence in the orange channel (Fig. 8f). Thus, in the living cells, the probe shows a turn-on mode in the presence of the low concentration range of Cys (Fig. 8c and g) and a ratiometric mode in the presence of the high concentration range of Cys (Fig. 8f, g, j and k, and 9). Similar results are noted when the probe is treated with another type of cells, MCF-7 cells (Fig. S21†). These imaging data in the living cells are consistent with the results in solution (Fig. 5). Thus, as designed, the probe CHMC-thiol is cell membrane permeable and can report low and high concentration ranges of thiols with distinct modes of fluorescence signals in the living cells.
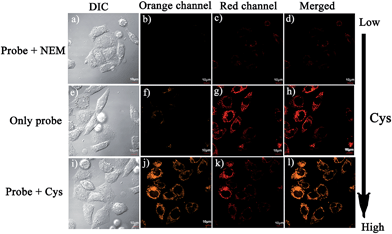 |
| Fig. 8 Bright-field and fluorescence images of HeLa cells stained with the CHMC-thiol: (a–d) bright-field and fluorescence images of the cells incubated with N-ethylmaleimide, and then co-incubated with CHMC-thiol for 30 min: (e–h) bright-field and fluorescence images of cells incubated only with the probe for 30 min: (i–l) bright-field and fluorescence images of cells incubated with Cys for 30 min, and then treated with the probe for 30 min: the orange and red channels are corresponding to the emission windows of 580–640, and 650–750 nm, respectively. Scale bar = 10 μm. | |
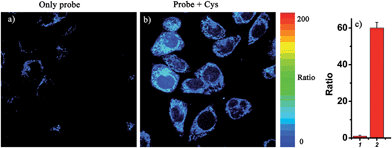 |
| Fig. 9 Pseudo-colored ratiometric images (Forange/Fred) of thiols in HeLa cells. (a) Ratio image (Forange/Fred) of the cells incubated only with the probe CHMC-thiol (5 μM) for 30 min; (b) ratio image (Forange/Fred) of the cells incubated with Cys (100 μM) for 30 min, and then treated with the probe (5 μM) for another 30 min; (c) average Forange/Fred intensity ratios in (a, b). The orange and red channels are corresponding to the emission windows of 580–640, and 650–750 nm, respectively. Data are expressed as mean ± SD of three experiments. | |
Imaging of CHMC-thiol to different concentration ranges of thiols in live animals
Based on the above studies, the prominent features of the probe CHMC-thiol include excellent NIR photophysical properties, two reactive sites with different sensitivity and excellent selectivity. In particular, the threshold of the low and high concentration ranges of CHMC-thiol is well located in the physiological concentration range of Cys (30–200 μM).14 These favorable attributes encourage us to further investigate the suitability of the probe for detecting different concentration ranges of thiols with distinct modes of fluorescence signals in the context of living mice. The Kunming mice were divided into three groups (Fig. 10). One group was given saline (100 μL) in the peritoneal cavity, followed by intraperitoneal (i.p.) injection with CHMC-thiol (20 μM, in 20 μL DMSO). The second group was given an i.p. injection of N-ethylmaleimide (1 mM, in 100 μL saline), and followed by i.p. injection with CHMC-thiol (20 μM, in 20 μL DMSO) as the negative control experiment. The third group was given an i.p. injection of Cys (100 μM, in 100 μL saline), and followed by i.p. injection with CHMC-thiol (20 μM, in 20 μL DMSO). As shown in Fig. 10b, the mice treated with only the probe exhibit a much higher fluorescence readout (pseudocolor) than the mice treated with NEM (Fig. 10a) in the red channel. In addition, the mice treated with only the probe display a much higher fluorescence readout (pseudocolor) than the mice incubated with NEM and the probe (Fig. 10d and e) in the orange channel, but less than the mice incubated with Cys and the probe (Fig. 10f). Thus, the probe exhibits a turn-on mode to the low concentration range of Cys (Fig. 10a, b and g(1, 2)) and a ratiometric signal to the high concentration range of Cys (Fig. 10b, c, e, f and h). Notably, the imaging data in the living animals are consistent with both the results in the solution (Fig. 5) and the living cells (Fig. 8). Thereby, these results demonstrate that the robust fluorescent probe CHMC-thiol can image the different concentration ranges of thiols with distinct modes of fluorescence signals in the living animals, for the first time.
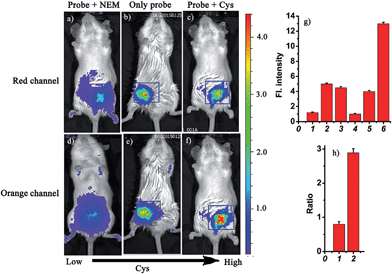 |
| Fig. 10 Representative fluorescent images (pseudo-color) of thiols (Cys) in the mice: (a) and (d) the mice were given an i.p. injection of N-ethylmaleimide and followed by injection with CHMC-thiol; (b) and (e) only the CHMC-thiol was injected in the peritoneal cavity of the mice; (c) and (f) the mice were given an i.p. injection of Cys and followed by an i.p. injection with CHMC-thiol. (g) Quantification of the fluorescence emission intensity from the abdominal area of the mice of groups a(1), b(2), c(3); d(4), e(5), f(6); (h) average Forange/Fred intensity ratios of the only probe group (1) and probe + Cys group (2). The mice were imaged with an excitation filter of 550 nm and the orange and red channels are corresponding to the emission windows of 580–640 nm, and 650–750 nm, respectively. Data were expressed as mean ± SD of three experiments. | |
Conclusions
In summary, we have described a unique approach for the development of concentration range-dependent fluorescent probes. Based on the proposed strategy, we have synthesized the first concentration range-dependent NIR fluorescent probe, CHMC-thiol, which, interestingly, is capable of monitoring the low concentration range of thiols with a turn-on fluorescence signal and providing a ratiometric fluorescence response to the high concentration range of thiols. We have further demonstrated that the new probe can be employed to sense the changes in the thiol concentration ranges with distinct modes of fluorescence signals in both living cells and living animals. In addition, the innovative NIR dye, CHMC, is superior to the traditional chloro-substituted cyanine and the recently developed HD NIR dyes with retaining both the regulation sites of these two types of NIR dyes. We believe that CHMC, which intrinsically bears two chemical handles, may serve as a useful fluorescent platform for the design of the concentration range-dependent NIR fluorescent probes as powerful molecular tools for biological and clinical investigations in living systems.
Acknowledgements
Funding was partially provided by NSFC (21172063, 21472067) and the startup fund of University of Jinan.
Notes and references
-
(a) X. Chen, Y. Zhou, X. Peng and J. Yoon, Chem. Soc. Rev., 2010, 39, 2120 RSC;
(b) C. Yin, F. Huo, J. Zhang, R. Martínez-Máñez, Y. Yang, H. Lv and S. Li, Chem. Soc. Rev., 2013, 42, 6032 RSC;
(c) H. S. Jung, X. Chen, J. S. Kim and J. Yoon, Chem. Soc. Rev., 2013, 42, 6019 RSC;
(d) L.-Y. Niu, Y.-Z. Chen, H.-R. Zheng, L.-Z. Wu, C.-H. Tung and Q.-Z. Yang, Chem. Soc. Rev., 2015, 44, 6143 RSC.
-
(a) O. Rusin, N. N. St Luce, R. A. Agbaria, J. O. Escobedo, S. Jiang, I. M. Warner, F. B. Dawan, K. Lian and R. M. Strongin, J. Am. Chem. Soc., 2004, 126, 438 CrossRef CAS PubMed;
(b) X. Yang, Y. Guo and R. M. Strongin, Angew. Chem., Int. Ed., 2011, 50, 10690 CrossRef CAS PubMed;
(c) L. Y. Niu, Y. S. Guan, Y. Z. Chen, L. Z. Wu, C. H. Tung and Q. Z. Yang, J. Am. Chem. Soc., 2012, 134, 18928 CrossRef CAS PubMed;
(d) J. Yin, Y. Kwon, D. Kim, D. Lee, G. Kim, Y. Hu, J.-H. Ryu and J. Yoon, J. Am. Chem. Soc., 2014, 136, 5351 CrossRef CAS PubMed;
(e) S. Y. Lim, K. H. Hong, D. I. Kim, H. Kwon and H. J. Kim, J. Am. Chem. Soc., 2014, 136, 7018 CrossRef CAS PubMed;
(f) J. Liu, Y. Q. Sun, Y. Huo, H. Zhang, L. Wang, P. Zhang and W. Guo, J. Am. Chem. Soc., 2014, 136, 574 CrossRef CAS PubMed;
(g) Z. Guo, S. W. Nam, S. Park and J. Yoon, Chem. Sci., 2012, 3, 2760 RSC.
-
(a) X. Lou, Y. Hong, S. Chen, C. W. T. Leung, N. Zhao, B. Situ, J. W. Y. Lam and B. Z. Tang, Sci. Rep., 2014, 4 DOI:10.1038/srep04272;
(b) H. S. Hewage and E. V. Anslyn, J. Am. Chem. Soc., 2009, 131, 13099 CrossRef CAS PubMed;
(c) C. S. Lim, G. Masanta, H. J. Kim, J. H. Han, H. M. Kim and B. R. Cho, J. Am. Chem. Soc., 2011, 133, 11132 CrossRef CAS PubMed;
(d) M. Zhang, M. Yu, F. Li, M. Zhu, M. Li, Y. Gao, T. Yi and C. Huang, J. Am. Chem. Soc., 2007, 129, 10322 CrossRef CAS PubMed;
(e) B. Tang, Y. Xing, P. Li, N. Zhang, F. Yu and G. Yang, J. Am. Chem. Soc., 2007, 129, 11666 CrossRef CAS PubMed;
(f) Y. H. Ahn, J. S. Lee and Y. T. Chang, J. Am. Chem. Soc., 2007, 129, 4510 CrossRef CAS PubMed;
(g) J. Shao, H. Sun, H. Guo, S. Ji, J. Zhao, W. Wu and T. D. James, Chem. Sci., 2012, 3, 1049 RSC;
(h) R. Wang, L. Chen, P. Liu, Q. Zhang and Y. Wang, Chem.–Eur. J., 2012, 18, 11343 CrossRef CAS PubMed;
(i) F. Wang, Z. Guo, X. Li, X. Li and C. Zhao, Chem.–Eur. J., 2014, 20, 11471 CrossRef CAS PubMed.
-
(a) L. D. Lavis and R. T. Raines, ACS Chem. Biol., 2014, 9, 855 CrossRef CAS PubMed;
(b) B. A. Smith, W. J. Akers, W. M. Leevy, A. J. Lampkins, S. Z. Xiao, W. Wolter, M. A. Suckow, S. Achilefu and B. D. Smith, J. Am. Chem. Soc., 2010, 132, 67 CrossRef CAS PubMed;
(c) K. Kundu, S. F. Knight, N. Willett, S. Lee, W. R. Taylor and N. Murthy, Angew. Chem., Int. Ed., 2009, 48, 299 CrossRef CAS PubMed;
(d) N. Karton-Lifshin, L. Albertazzi, M. Bendiko, P. S. Baran and D. Shabat, J. Am. Chem. Soc., 2012, 134, 20412 CrossRef CAS PubMed;
(e) W. Xuan, C. Sheng, Y. Cao, W. He and W. Wang, Angew. Chem., Int. Ed., 2012, 51, 2282 CrossRef CAS PubMed;
(f) X. Wu, M. Yu, B. Lin, H. Xing, J. Han and S. Han, Chem. Sci., 2015, 6, 798 RSC;
(g) J. J. Hu, N.-K. Wong, S. Ye, X. Chen, M.-Y. Lu, A. Q. Zhao, Y. Guo, A. C.-H. Ma, A. Y.-H. Leung, J. Shen and D. Yang, J. Am. Chem. Soc., 2015, 137, 6837 CrossRef CAS PubMed;
(h) C. Huang, T. Jia, M. Tang, Q. Yin, W. Zhu, C. Zhang, Y. Yang, N. Jia, Y. Xu and X. Qian, J. Am. Chem. Soc., 2014, 136, 14237 CrossRef CAS PubMed.
-
(a) K. Kiyose, H. Kojima and T. Nagano, Chem.–Asian J., 2008, 3, 506 CrossRef CAS;
(b) M. Lin, B. Fimmel, K. Radacki and F. Würthner, Angew. Chem., Int. Ed., 2011, 50, 10847 CrossRef CAS PubMed;
(c) G. M. Fischer, E. Daltrozzo and A. Zumbusch, Angew. Chem., Int. Ed., 2011, 50, 1406 CrossRef CAS PubMed;
(d) T. Hirayamaa, G. C. Van de Bittnera, L. W. Grayb, S. Lutsenkob and C. J. Chang, Proc. Natl. Acad. Sci. U. S. A., 2012, 109, 2228 CrossRef PubMed;
(e) M. Cui, M. Ono, H. Watanabe, H. Kimura, B. Liu and H. Saji, J. Am. Chem. Soc., 2014, 136, 3388 CrossRef CAS PubMed;
(f) A. T. Wrobel, T. C. Johnstone, A. Deliz-Liang, S. J. Lippard and P. Rivera-Fuentes, J. Am. Chem. Soc., 2014, 136, 4697 CrossRef CAS PubMed.
-
(a) M. Schäerling, Angew. Chem., Int. Ed., 2012, 51, 3532 CrossRef PubMed;
(b) Y. Yang, Q. Zhao, W. Feng and F. Li, Chem. Rev., 2013, 113, 192 CrossRef CAS PubMed;
(c) X. Li, X. Gao, W. Shi and H. Ma, Chem. Rev., 2014, 114, 590 CrossRef CAS PubMed;
(d) R. Guliyev, A. Coskun and E. U. Akkaya, J. Am. Chem. Soc., 2009, 131, 9007 CrossRef CAS PubMed;
(e) Y. Ueno, J. Jose, A. Loudet, C. Pérez-Bolívar, P. Anzenbacher Jr. and K. Burgess, J. Am. Chem. Soc., 2011, 133, 51 CrossRef CAS PubMed;
(f) E. Heyer, P. Retailleau and R. Ziessel, Org. Lett., 2014, 16, 2330 CrossRef CAS PubMed;
(g) F. Yu, P. Li, B. Wang and K. Han, J. Am. Chem. Soc., 2013, 135, 7674 CrossRef CAS PubMed;
(h) J. Ling, G. Naren, J. Kelly, T. S. Moody and A. P. de Silva, J. Am. Chem. Soc., 2015, 137, 3763 CrossRef CAS PubMed.
-
(a) M. H. Lee, B. Yoon, J. S. Kim and J. L. Sessler, Chem. Sci., 2013, 4, 4121 RSC;
(b) X. Wang, L. Cui, N. Zhou, W. Zhu, R. Wang, X. Qian and Y. Xu, Chem. Sci., 2013, 4, 2936 RSC;
(c) C. Liu, W. Chen, W. Shi, B. Peng, Y. Zhao, H. Ma and M. Xian, J. Am. Chem. Soc., 2014, 136, 7257 CrossRef CAS PubMed;
(d) Z. Huang, S. Yu, K. Wen, X. Yu and L. Pu, Chem. Sci., 2014, 5, 3457 RSC;
(e) D. R. Rooker and D. Buccella, Chem. Sci., 2015, 6, 6456 RSC;
(f) Y. You and W. Nam, Chem. Sci., 2014, 5, 4123 RSC;
(g) Y.-J. Huang, W.-J. Ouyang, X. Wu, Z. Li, J. S. Fossey, T. D. James and Y.-B. Jiang, J. Am. Chem. Soc., 2013, 135, 1700 CrossRef CAS PubMed;
(h) J. Zhou, L. Li, W. Shi, X. Gao, X. Li and H. Ma, Chem. Sci., 2015, 6, 4884 RSC;
(i) Q. Zhao, X. Zhou, T. Cao, K. Y. Zhang, L. Yang, S. Liu, H. Liang, H. Yang, F. Li and W. Huang, Chem. Sci., 2015, 6, 1825 RSC.
-
(a) H. Komatsu, T. Miki, D. Citterio, T. Kubota, Y. Shindo, Y. Kitamura, K. Oka and K. Suzuki, J. Am. Chem. Soc., 2005, 127, 10798 CrossRef CAS PubMed;
(b) L. Yuan, W. Lin, Y. Xie, B. Chen and S. Zhu, J. Am. Chem. Soc., 2012, 134, 1305 CrossRef CAS PubMed;
(c) X. Gao, X. Li, L. Li, J. Zhou and H. Ma, Chem. Commun., 2015, 51, 9388 RSC.
-
(a) L. Strekowski, M. Lipowska and G. Patonay, J. Org. Chem., 1992, 57, 4578 CrossRef CAS;
(b) X. Peng, F. Song, E. Lu, Y. Wang, W. Zhou, J. Fan and Y. Gao, J. Am. Chem. Soc., 2005, 127, 4170 CrossRef CAS PubMed.
- L. Yuan, W. Lin, S. Zhao, W. Gao, B. Chen, L. He and S. Zhu, J. Am. Chem. Soc., 2012, 134, 13510 CrossRef CAS PubMed.
-
(a) H. Maeda, H. Matsuno, M. Ushida, K. Katayama and K. Saeki, Angew. Chem., Int. Ed., 2005, 44, 2922 CrossRef CAS PubMed;
(b) J. Zhang, A. Shibata, M. Ito, S. Shuto, Y. Ito, B. Mannervik, H. Abe and R. Morgenstern, J. Am. Chem. Soc., 2011, 133, 14109 CrossRef CAS PubMed;
(c) W. Jiang, Q. Fu, H. Fan, J. Ho and W. Wang, Angew. Chem., Int. Ed., 2007, 46, 8445 CrossRef CAS PubMed;
(d) X. Liu, W. Zhang, C. Li, W. Zhou, Z. Li, M. Yu and L. Weia, RSC Adv., 2015, 5, 4941 RSC.
-
(a) L.-Y. Niu, H.-R. Zheng, Y.-Z. Chen, L.-Z. Wu, C.-H. Tung and Q.-Z. Yang, Analyst, 2014, 139, 1389 RSC;
(b) X. Wang, J. Lv, X. Yao, Y. Li, F. Huang, M. Li and B. Tang, Chem. Commun., 2014, 50, 15439 RSC.
- The pKa was calculated according to the Henderson–Hasselbalch-type mass action equation (log[(Fmax − F)/(F − Fmin)] = pKa − pH).
-
(a) T. K. Chung, M. A. Funk and D. H. Baker, J. Nutr., 1990, 120, 158 CAS;
(b) S. Park and J. A. Imlay, J. Bacteriol., 2003, 185, 1942 CrossRef CAS PubMed.
-
(a) J. Liu, Y.-Q. Sun, H. Zhang, Y. Huo, Y. Shi and W. Guo, Chem. Sci., 2014, 5, 3183 RSC;
(b) Y. Kim, S. V. Mulay, Mi. Choi, S. B. Yu, S. Jon and D. G. Churchill, Chem. Sci., 2015, 6, 5435 RSC;
(c) F. Wang, L. Zhou, C. Zhao, R. Wang, Q. Fei, S. Luo, Z. Guo, H. Tian and W.-H. Zhu, Chem. Sci., 2015, 6, 2584 RSC.
Footnote |
† Electronic supplementary information (ESI) available. See DOI: 10.1039/c5sc03591k |
|
This journal is © The Royal Society of Chemistry 2016 |
Click here to see how this site uses Cookies. View our privacy policy here.