DOI:
10.1039/D4SU00219A
(Perspective)
RSC Sustain., 2024,
2, 2190-2198
A greener prescription: the power of natural organic materials in healthcare
Received
4th May 2024
, Accepted 9th July 2024
First published on 9th July 2024
Abstract
Natural organic materials (NM), which are biodegradable, biocompatible, renewable, and electroactive, offer a sustainable solution to the environmental impact of technological progress. This perspective emphasizes NM's potential to reduce environmental impact and create sustainable solutions for medical devices and disposable products. Nonetheless, challenges remain in scaling up production and addressing durability. Integrating natural systems into technological processes can help achieve a more eco-friendly and balanced future.
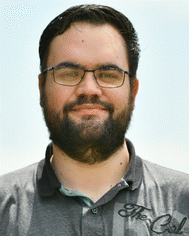 João V. Paulin | Dr João V. Paulin is a researcher at São Paulo State University (UNESP) in the Department of Physics and Meteorology. He earned his PhD in Science and Technology of Materials from UNESP, and his scientific contributions were recognized with the prestigious Thesis Award from the Brazilian Coordination for the Improvement of Higher Education Personnel (CAPES). This award recognized his doctoral thesis as the best within the Materials field in Brazil in 2021. Dr Paulin's expertise extends to the domains of Materials Science and Applied Physics. Currently, his research interests are at the intersection of biomaterials, sustainability, and organic (bio)electronics. |
Sustainability spotlight
Technological progress needs to be aligned with sustainable practices as it can have a negative impact on the environment. Using natural materials can reduce plastic pollution, develop biodegradable medical electronics to improve patient outcomes and reduce healthcare costs, as well as enable the development of energy-harvesting and energy-storage devices that can power implantable and wearable health devices. This approach is, therefore, related to SDG 3 (Good Health and Well-being), SDG 7 (Affordable and Clean Energy), SDG 12 (Responsible Consumption and Production) and SDG 14 (Life Below Water).
|
Introduction
In today's world, technological progress and environmental degradation can be viewed as two sides of the same coin. As much as the former is celebrated, we cannot close our eyes to the warning signs of the adverse impact of human-driven activities on the environment.1,2
From smartphones and smart homes to self-driving cars and artificial intelligence, the fast pace at which electronic technology is evolving is shaping our lives and how we approach healthcare. Take, for example, the recent coronavirus disease (COVID-19) outbreak. Biosensors, in particular, have shown immense promise for real-time health monitoring and disease control.3 Nonetheless, their uninterrupted use further increased the amount of single-use medical items and non-biodegradable plastics.2,4,5 As the demand for healthcare electronics grows, its development must be aligned with sustainable practices to mitigate the adverse impact on the environment.
Nature and healthcare
In order to achieve sustainable healthcare technology, Nature itself can have a solution. Through the years of evolution and adaptations, Nature has provided us with an extensive range of organic materials derived from animals, plants, fungi, and bacteria. These natural organic materials (NM) possess mechanical, physical, and chemical properties that align with current healthcare needs.6–8 They are also biologically active, biocompatible, biodegradable, renewable, and cost-effective.6–8
One significant advantage of NM is its biodegradability, which offers a low carbon footprint solution to address the problem of plastic pollution and replace conventional fossil-fuel-based plastics.9–11 The feasibility of such a substitution is becoming increasingly evident to the extent that the US government has set an admirable goal of ensuring that 90% of all plastic will be from a bio-based origin within the next two decades.12 For example, natural polymers such as cellulose (found in the cell walls of plants) and chitin (found in the exoskeletons of crustaceans and insects) provide solutions to combat plastic pollution due to their biodegradability and impressive mechanical properties.8,13 Cellulose, for instance, exhibits exceptional strength and durability comparable to traditional plastics, along with resilience to weather and chemicals.14,15 Similarly, chitin demonstrates biocompatibility, biodegradability, and remarkable resistance to microbial degradation,9,16 making it valuable in medical and environmental applications.
The NM used in bioplastics can be sourced from renewable plant biomass and natural sources, promoting environmental sustainability and reducing dependence on non-renewable resources.17,18 Bioplastics can also be designed to be biodegradable or compostable, facilitating reuse through recycling or organic waste management systems.10,11,19 Ongoing advancements in extraction techniques aim to enhance efficiency and minimize environmental impact.10,17 Enhancing their mechanical properties and extending their lifespan through formulation optimization is also a research theme.17,18 These attributes underscore their role as a sustainable alternative to conventional plastics. It is worth noting that, while more environmentally friendly than traditional petroleum-based plastics, bioplastics can still contribute to undesirable land use and greenhouse gas emissions.9
Another interesting consequence of using nature in healthcare is developing new medicines from plants and microorganisms, the so-called bioprospecting. A good example is Taxol, a plant-based chemotherapeutic agent obtained from yew trees. U.S. Food and Drug Administration (FDA) approved this compound for treating different types of cancers because it inhibits cell proliferation.20
However, Nature is capable of much more. The core of the earliest evidence from humankind implants, back to the Neolithic period, was based on ivory, nacre, various types of animal tissues, silk, and wood. Nowadays, silk fibers are re-emerging as promising candidates for use in sutures, ligaments, and tendons, as they are flexible and incredibly strong.8 Silk offers a slower degradation rate than absorbable fiber sutures like polyglycolic acid (PGA). While PGA dissolves in about 60–90 days, silk can take up to two years to completely degrade in vivo,21,22 providing prolonged support for wound healing and causing less inflammatory response. In ligament and tendon repair, silk supports cell attachment and proliferation, promoting better healing and integration with native tissues than synthetic materials like polyethylene terephthalate.8,21,23 However, the scalability of silk production and its standardization for medical use still require further research and development.22
To add on, natural rubber latex (NRL) has shown multiple applications in enhancing tissue repair in critical bone defects and chronic wounds, as well as enabling a sustained and controlled local drug release.24,25 As an example, the antioxidant activity of curcumin has been preserved after incorporation into NRL, providing anti-aging benefits with promising applications in the cosmetics industry.26 Additionally, the treatment of inflammatory diseases and candida spp. infected burn wounds have been achieved by combining NRL with synthetic drugs like ibuprofen,27 organic materials such as silver sulfadiazine,28 and composites made with natural resins (red propolis) and metallic copper ions.29
In reality, NM is witnessing an enormous blossoming in healthcare in terms of exploration and applications. These materials are being considered for various healthcare settings, such as biomedical implants, bioprosthetics, bioadhesives, sealants, cardiovascular therapy, cosmetics, surgical sutures, sensors, tissue grafts and engineering, and wound healing (Fig. 1).8 Therefore, the economic impact of these materials in the biomedical field can be impressive.
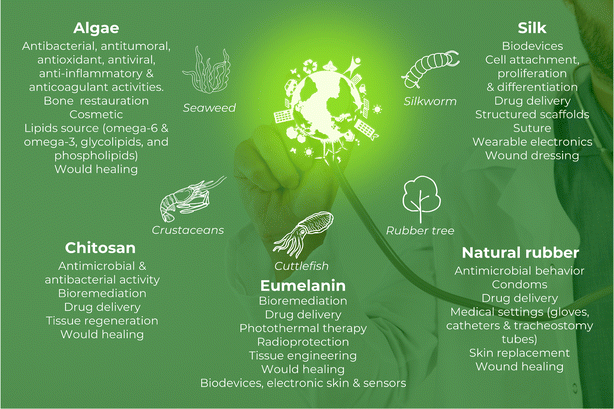 |
| Fig. 1 Typical healthcare applications of a few natural organic materials. | |
The development of implantable sensors and diagnostic tools is sparking a notable sense of excitement due to the potential to provoke a new era in healthcare through unprecedented precision and efficacy.6,8,30 Although these biodevices are electronic systems designed to interact with biological and living tissue safely, they are generally built upon non-renewable plastics and metal sources, which may lead to inflammation, rejection, or even toxic effects in the body. By contrast, implementing the inherent biocompatibility of NM into the system would make them less likely to cause any adverse reaction.6,8 Indeed, electronic devices based on hyaluronic acid (HA) showed no cell cytotoxic response,31 in contrast to poly(3,4-ethylenedioxythiophene) polystyrene sulfonate (PEDOT:PSS), a well-known biocompatible synthetic polymer.32 In this case, fluorescence-activated cell sorting revealed that the PEDOT:PSS device can have a damaged cell ratio higher than 41%, which is significantly higher than the HA-based device with 5.7% cell damage.31
Similarly, the ability of NM to naturally degrade over time and be metabolized by the body can improve patient outcomes by reducing the need to remove the implanted devices, thus avoiding potential surgical complications.6,8,30 Also, reduced healthcare costs would be expected since there will be no need for additional surgeries or treatments. Consequently, such medical electronics could be more accessible to low-income communities, improving their quality of life.
NM also possesses electrical, optical, and magnetically active features essential for developing high-performance and accurate biodevices to better interact with living tissue.30,33 These devices could monitor the patient's health and provide real-time data, enabling the detection and treatment of complications at an early stage.
Another exciting area for health electronics relies on energy-harvesting devices that can convert body heat, biofluids, and human-body motions into electricity.1,6,34,35 These systems would have the efficacy to power implantable and wearable health devices without the need for batteries or constant charging. As an emerging demonstration, a flexible and transparent triboelectric nanogenerator capable of harvesting energy from water, wind, and human motion was developed using silver nanowires and a cellulose-based material.35 Combining the above characteristics with the renewability of NM can assist in reducing the carbon footprint associated with resource extraction (by minimizing the use of toxic chemicals and pollutants) and use.
Challenges and opportunities
There are several benefits to using NM, but there are also challenges associated with their technological development. For instance, NM's performance and durability may limit their use in long-term applications compared to traditional inorganic materials. Additionally, the harsh conditions of standard nano and microfabrication techniques need to be adapted to NM's soft and gentle traits to implement them into the devices.36
The main challenge is producing NM on a large scale, which may require specialized equipment or expertise to separate it from its natural source and remove any impurities or contaminants.7 These factors can increase production costs and create additional obstacles to commercialization and widespread adoption. Furthermore, large-scale production of NM can have a significant environmental impact, particularly if energy-intensive processes are used. Reducing this impact will require innovative methods and more sustainable practices.37
Noteworthily, adding financial value to what is typically seen as waste from the agricultural and food industries can be a viable possibility to alleviate such a rise in cost.7,38 This means that the “waste” products such as corn stover, sugarcane bagasse, coffee grounds, and fruit peels can be processed to isolate carbon-rich material and natural extracts with all the above-mentioned properties. In addition, this approach has the advantage of reducing environmental pollution by avoiding the burning or disposing of such products in unplanned landfills, thereby lowering the carbon footprint of the production process.
The advances in chemistry and materials science also offer a promising alternative to develop materials that have not only the unique properties of the NM but also the chemical flexibility to tailor their properties to any specific needs.7,33 Thus, greater versatility and customization in material design can improve performance and efficiency. Additionally, adopting a synthetic approach can assist in mitigating ethical concerns associated with animal-based materials by avoiding harmful and exploitative practices.7
The use of NM in healthcare is a developing concept with great potential to revolutionize the field, but it's not a one-size-fits-all solution. As research in this area advances, input from scientists, engineers, and healthcare professionals will lead to innovative nature-based biodevices with a real impact on patient outcomes. Whether or not to use NM will depend on the specific application, environmental impact, and economic feasibility. While inorganic materials have been viewed as the best option for their superior durability, we must also consider the environmental impact of their production and disposal.2 NM, on the other side, offers a sustainable alternative to reduce the strain on non-renewable resources and promote a healthier ecosystem. However, it is crucial to manage the production of NM to keep costs feasible and minimize environmental impact, including reducing carbon emissions through energy-efficient methods.37
Combining the best of these two worlds could further positively impact healthcare by maximizing its advantages. This connection can result in a medical setting with enhanced durability, cost-effectiveness, and biodegradability while still addressing the medical needs of biocompatibility and mechanical softness that inorganic materials do not match. J. A. Rogers's group showed a good representation of a direct couple of the brain tissue and rigid and inflexible metallic electrodes using NM. They demonstrated that silk (from Bombyx mori silkworms cocoons) is dissolved and resorbed by the biological tissue, allowing a spontaneous and conformal wrapping process of the ultrathin electronics at the biotic/abiotic interface.39 Accordingly, the mechanical mismatch of this interface is reduced without losing the implanted electrode performance.
Eumelanin in focus
Eumelanin is a key natural pigment found throughout nature. In humans, it is present in the skin, hair and eyes, as well as several internal regions of the body, like the locus coeruleus and substantia nigra regions of the brain, inner ear (cochlea) and melanoma cells.7,40 Eumelanin draws attention not only for its biological role in photochemical reactions, antioxidant protection, and neurological disorders but also for its unique properties and applications in healthcare and biotechnology.7
As part of the broader exploration into the use of natural organic materials in medical technology, eumelanin presents intriguing characteristics such as biocompatibility,41,42 biodegradability,43,44 and photoprotection,7,40 which offer new avenues for developing sustainable and biodegradable medical settings. Indeed, in the medical realm, eumelanin has already been explored as thermo- and/or photostabilizers,45–47 bioremediation,48–50 matrix for bactericidal systems,51 controlled drug delivery,52,53 photothermal therapeutic agent,54–56 and reduced inflammation.57,58
Eumelanin also showed photoconversion59 and energy storage capabilities.60–63 For instance, using an eumelanin anode (0.6 g of active material) along with a manganese oxide cathode could sustain 5 mW of power for 20 hours.61 Meanwhile, an eumelanin cathode (0.6 g of active material) with a sodium-titanium phosphate anode generated 18 mW of power for 16 hours.60 These power outputs are sufficient to satisfy the power needs of several current medical devices.64 Additionally, eumelanin systems exhibit stable specific capacities (over 60 mA h g−1) after 500 cycles, with coulombic efficiencies maintained above 99.2%.61 In supercapacitor configuration, eumelanin-based electrodes also show coulombic efficiencies close to 100% even after 5000 cycles.59,65 Thus, these systems can be rechargeable. Besides, active electrode material in these configurations fits the standard sizes typically used in wireless ingestible devices.64
NM has an intrinsic ability to generate electrical charges, but it has been notoriously hard to obtain electrically conductive variants over the years. Unlike solid-state electronics, where electron and hole transfer are common, most biological activity is driven by cation and anion fluidic motions.66 This fundamental difference creates challenges in selecting suitable materials for biotic–abiotic interfaces. Here, eumelanin can be a good material candidate, as it shows electroactivity7,67–69 with the ability to transduce ionic signals into electronic ones.70–72 Aligning eumelanin's processability into self-healing73 and flexible substrates62,72 with the aforementioned feature makes it a versatile material for the fabrication of advanced wound dressing systems. Indeed, eumelanin derivatives have been tested as pH sensors after contact with human plasma72 and in bacterial cultures74 without compromising their effectiveness.
Not only can eumelanin be integrated into bioelectronic systems, but it can also play an active role in the wound-healing process itself.75–77 Eumelanin is known for its antioxidant, antibacterial, and tissue-regeneration properties, offering significant potential for improving wound treatment outcomes. By incorporating eumelanin into formulations for dressings, it is possible to harness its properties to protect tissues against oxidative stress, prevent infections, and modulate the inflammatory response. Also, eumelanin can promote tissue regeneration, thus accelerating the healing process. The possibility of developing eumelanin-based drug delivery systems also presents an exciting opportunity to deliver therapeutic agents directly to the wound site, providing targeted and effective treatment. This innovative approach holds the potential to significantly enhance wound care outcomes, offering a promising solution to the ongoing challenge of wound healing.
Given eumelanin's hydration-based conductive properties,68,78–80 it could also be integrated into wearable transient to assess skin hydration, monitoring human respiration patterns and speech recognition by tracking alterations in water molecules concentration through air exhalation.81,82
Despite its numerous advantages, eumelanin is constrained by challenges that should not be overlooked. The production process can be complex and time-consuming and is not yet fully optimized for large-scale industrial production.7,83 Costly extraction and purification procedures further restrict its viability in commercial sectors. Additionally, scalability issues persist, with current manufacturing techniques needing refinement to ensure yield and consistency. Nonetheless, promising cost-effective methodologies are emerging as researchers are exploring innovative methods to overcome these obstacles and enhance the feasibility of eumelanin for broader industrial use.84,85
Moreover, natural variations in eumelanin sources contribute to inconsistencies in quality and properties,7,80 making it difficult to produce reliable, cost-effective product development. Potential solutions lie in in vitro synthesis, promising controlled and consistent production conditions.
Furthermore, achieving precise structural control during eumelanin synthesis remains a significant challenge. Structural integrity dictates its physical and chemical properties, including electrical conductivity and optical characteristics, which depend heavily on its molecular arrangement.63,86–88 Advances in chemistry and material science are being explored to manipulate eumelanin's molecular structure,89–93 leading to predictable properties essential for any specific application. Continued research and development efforts are imperative to make eumelanin viable for various commercial industries.
Conclusion
With the science community embracing different perspectives and new and innovative approaches in healthcare, the promises to uncover opportunities for growth and success are limitless. A holistic approach is essential for comprehending the positive effects of NM that could emerge on the environment, economy, and social spheres. As always, there are multiple solutions to this problem, and considering NM is just one of them. Nevertheless, the outlined approach is not only beautiful but also incredibly effective. By working together to put the power of Nature to good use, a better world for ourselves and future generations can be mastered.
Data availability
Data sharing does not apply to this article as no datasets were generated or analyzed during the current study.
Conflicts of interest
There are no conflicts to declare.
Acknowledgements
J. V. P. thanks the support of the FUNDUNESP and São Paulo Research Foundation (FAPESP, grant 2020/15869-6).
References
- J. Zhu, H. Wen, H. Zhang, P. Huang, L. Liu and H. Hu, Recent Advances in Biodegradable Electronics- from Fundament to the next-Generation Multi-Functional, Medical and Environmental Device, Sustainable Mater. Technol., 2023, 35, e00530, DOI:10.1016/j.susmat.2022.e00530
.
- M. P. Cenci, T. Scarazzato, D. D. Munchen, P. C. Dartora, H. M. Veit, A. M. Bernardes and P. R. Dias, Eco-Friendly Electronics – A Comprehensive Review, Adv. Mater. Technol., 2022, 7, 2001263, DOI:10.1002/admt.202001263
.
- L. M. Cerdeira Ferreira, D. Lima, L. H. Marcolino-Junior, M. F. Bergamini, S. Kuss and F. Campanhã Vicentini, Cutting-Edge Biorecognition Strategies to Boost the Detection Performance of COVID-19 Electrochemical Biosensors: A Review, Bioelectrochemistry, 2024, 157, 108632, DOI:10.1016/j.bioelechem.2023.108632
.
- S. Bhattacharjee, P. Bahl, A. A. Chughtai, D. Heslop and C. R. MacIntyre, Face Masks and Respirators: Towards Sustainable Materials and Technologies to Overcome the Shortcomings and Challenges, Nano Sel., 2022, 3, 1355–1381, DOI:10.1002/nano.202200101
.
- P. K. Rai, C. Sonne, H. Song and K. H. Kim, Plastic Wastes in the Time of COVID-19: Their Environmental Hazards and Implications for Sustainable Energy Resilience and Circular Bio-Economies, Sci. Total Environ., 2023, 858, 159880, DOI:10.1016/j.scitotenv.2022.159880
.
- V. Slabov, S. Kopyl, M. P. Soares dos Santos and A. L. Kholkin, Natural and Eco-Friendly Materials for Triboelectric Energy Harvesting, Nano-Micro Lett., 2020, 12, 42, DOI:10.1007/s40820-020-0373-y
.
- J. V. Paulin and C. F. O. Graeff, From Nature to Organic (Bio)Electronics: A Review on a Melanin-Inspired Material, J. Mater. Chem. C, 2021, 9, 14514–14531, 10.1039/D1TC03029A
.
- E. Insuasti-Cruz, V. Suárez-Jaramillo, K. A. Mena Urresta, K. O. Pila-Varela, X. Fiallos-Ayala, S. A. Dahoumane and F. Alexis, Natural Biomaterials from Biodiversity for Healthcare Applications, Adv. Healthcare Mater., 2022, 11, 2101389, DOI:10.1002/adhm.202101389
.
- G. Atiwesh, A. Mikhael, C. C. Parrish, J. Banoub and T. A. T. Le, Environmental Impact of Bioplastic Use: A Review, Heliyon, 2021, 7, e07918, DOI:10.1016/j.heliyon.2021.e07918
.
- J. G. Rosenboom, R. Langer and G. Traverso, Bioplastics for a Circular Economy, Nat. Rev. Mater., 2022, 7, 117–137, DOI:10.1038/s41578-021-00407-8
.
- W. A. Ahsan, A. Hussain, C. Lin and M. K. Nguyen, Biodegradation of Different Types of Bioplastics through Composting—A Recent Trend in Green Recycling, Catalysts, 2023, 13, 294, DOI:10.3390/catal13020294
.
-
Bold Goals for U.S., Biotechnology and Biomanufacturing: Harnessing Research and Development to Further Societal Goals, 2023, https://www.whitehouse.gov/wp-content/uploads/2023/03/Bold-Goals-for-U.S.-Biotechnology-and-Biomanufacturing-Harnessing-Research-and-Development-To-Further-Societal-Goals-FINAL.pdf.
- J. W. Roy Chong, X. Tan, K. S. Khoo, H. S. Ng, W. Jonglertjunya, G. Y. Yew and P. L. Show, Microalgae-Based Bioplastics: Future Solution towards Mitigation of Plastic Wastes, Environ. Res., 2022, 206, 112620, DOI:10.1016/j.envres.2021.112620
.
- Q. Xia, C. Chen, Y. Yao, J. Li, S. He, Y. Zhou, T. Li, X. Pan, Y. Yao and L. Hu, A Strong, Biodegradable and Recyclable Lignocellulosic Bioplastic, Nat. Sustain., 2021, 4, 627–635, DOI:10.1038/s41893-021-00702-w
.
- S. Zhang, Q. Fu, H. Li, P. Wu, G. I. N. Waterhouse, Y. Li and S. Ai, A Pectocellulosic Bioplastic from Fruit Processing Waste: Robust, Biodegradable, and Recyclable, Chem. Eng. J., 2023, 463, 142452, DOI:10.1016/j.cej.2023.142452
.
- Y. Zhou, Y. He, X. Lin, Y. Feng and M. Liu, Sustainable, High-Performance, and Biodegradable Plastics Made from Chitin, ACS Appl. Mater. Interfaces, 2022, 14, 46980–46993, DOI:10.1021/acsami.2c12764
.
- P. Rai, S. Mehrotra, S. Priya, E. Gnansounou and S. K. Sharma, Recent Advances in the Sustainable Design and Applications of Biodegradable Polymers, Bioresour. Technol., 2021, 325, 124739, DOI:10.1016/j.biortech.2021.124739
.
- A. S. Khandeparkar, R. Paul, A. Sridhar, V. V. Lakshmaiah and P. Nagella, Eco-Friendly Innovations in Food Packaging: A Sustainable Revolution, Sustainable Chem. Pharm., 2024, 39, 101579, DOI:10.1016/j.scp.2024.101579
.
- A. L. Merchan, T. Fischöder, J. Hee, M. S. Lehnertz, O. Osterthun, S. Pielsticker, J. Schleier, T. Tiso, L. M. Blank, J. Klankermayer, R. Kneer, P. Quicker, G. Walther and R. Palkovits, Chemical Recycling of Bioplastics: Technical Opportunities to Preserve Chemical Functionality as Path towards a Circular Economy, Green Chem., 2022, 24, 9428–9449, 10.1039/d2gc02244c
.
- A. Sharma, S. K. Bhatia, A. Banyal, I. Chanana, A. Kumar, D. Chand, S. Kulshrestha and P. Kumar, An Overview on Taxol Production Technology and Its Applications as Anticancer Agent, Biotechnol. Bioprocess Eng., 2022, 27, 680–702, DOI:10.1007/s12257-022-0063-3
.
- Y. Li, Q. Meng, S. Chen, P. Ling, M. A. Kuss, B. Duan and S. Wu, Advances, Challenges, and Prospects for Surgical Suture Materials, Acta Biomater., 2023, 168, 78–112, DOI:10.1016/j.actbio.2023.07.041
.
- M. Rahman, T. M. Dip, M. G. Nur, R. Padhye and S. Houshyar, Fabrication of Silk Fibroin-Derived Fibrous Scaffold for Biomedical Frontiers, Macromol. Mater. Eng., 2024, 309, 2300422, DOI:10.1002/mame.202300422
.
- M. Xiao, J. Yao, Z. Shao and X. Chen, Silk-Based 3D Porous Scaffolds for Tissue Engineering, ACS Biomater. Sci. Eng., 2024, 10, 2827–2840, DOI:10.1021/acsbiomaterials.4c00373
.
- N. B. Guerra, G. Sant'Ana Pegorin, M. H. Boratto, N. R. de Barros, C. F. de Oliveira Graeff and R. D. Herculano, Biomedical Applications of Natural Rubber Latex from the Rubber Tree Hevea Brasiliensis, Mater. Sci. Eng., C, 2021, 126, 112126, DOI:10.1016/j.msec.2021.112126
.
- R. D. Herculano, C. U. Mussagy, N. B. Guerra, G. Sant'Ana Pegorin Brasil, J. F. Floriano, B. S. Burd, Y. Su, J. C. da Silva Sasaki, P. A. C. Marques, M. Scontri, M. C. R. Miranda, E. S. Ferreira, F. L. Primo, M. A. Fernandes, S. He, S. Forster, C. Ma, P. E. de Lima Lopes Filho, L. S. dos Santos, G. R. Silva, A. E. M. Crotti, N. R. de Barros, B. Li and R. J. de Mendonça, Recent Advances and Perspectives on Natural Latex Serum and Its Fractions for Biomedical Applications, Biomater. Adv., 2024, 157, 213739, DOI:10.1016/j.bioadv.2023.213739
.
- F. K. de Souza Silva, C. B. Costa-Orlandi, M. A. Fernandes, G. S. A. Pegorin Brasil, C. U. Mussagy, M. Scontri, J. C. da S. Sasaki, A. P. de Sousa Abreu, N. B. Guerra, J. F. Floriano, R. J. de Mendonça, G. F. Caetano, N. Farhadi, A. Gómez, S. Huang, A. M. Farias, F. L. Primo, B. Li, A. M. Fusco-Almeida, M. R. Dokmeci, V. Jucaud, M. J. S. Mendes-Giannini, M. R. Cardoso and R. D. Herculano, Biocompatible Anti-Aging Face Mask Prepared with Curcumin and Natural Rubber with Antioxidant Properties, Int. J. Biol. Macromol., 2023, 242, 124778, DOI:10.1016/j.ijbiomac.2023.124778
.
- A. de F. Lima, G. S. Pegorin, M. C. R. Miranda, J. P. Cachaneski-Lopes, W. de M. Silva, F. A. Borges, N. B. Guerra, R. D. Herculano and A. Batagin-Neto, Ibuprofen-Loaded Biocompatible Latex Membrane for Drug Release: Characterization and Molecular Modeling, J. Appl. Biomater. Funct. Mater., 2021, 19, 1–13, DOI:10.1177/22808000211005383
.
- J. L. Tanaka, C. B. Costa-Orlandi, B. S. Burd, G. S. A. Pegorin, T. V. da Silva, N. B. Guerra, M. J. S. Mendes-Giannini, A. M. Fusco-Almeida, R. D. Herculano and N. R. de Barros, Natural Rubber Dressing Loaded with Silver Sulfadiazine for the Treatment of Burn Wounds Infected with Candida Spp, Int. J. Biol. Macromol., 2021, 189, 597–606, DOI:10.1016/j.ijbiomac.2021.08.102
.
- A. R. Bystronski, N. B. Guerra, C. Aguzzoli, J. S. Crespo and M. Giovanela, Preparation and Characterization of Natural Rubber Latex Films Containing Red Propolis and Copper for Biomedical Applications, MRS Adv., 2024, 9, 90–95, DOI:10.1557/s43580-023-00651-w
.
- H. C. Ates, P. Q. Nguyen, L. Gonzalez-Macia, E. Morales-Narváez, F. Güder, J. J. Collins and C. Dincer, End-to-End Design of Wearable Sensors, Nat. Rev. Mater., 2022, 7, 887–907, DOI:10.1038/s41578-022-00460-x
.
- J. H. Lee, Y. S. Rim, W. K. Min, K. Park, H. T. Kim, G. Hwang, J. Song and H. J. Kim, Biocompatible and Biodegradable Neuromorphic Device Based on Hyaluronic Acid for Implantable Bioelectronics, Adv. Funct. Mater., 2021, 31, 2107074, DOI:10.1002/adfm.202107074
.
- M. Berggren and A. Richter-Dahlfors, Organic Bioelectronics, Adv. Mater., 2007, 19, 3201–3213, DOI:10.1002/adma.200700419
.
- X. Ma, Z. Jiang, L. Xiang and F. Zhang, Natural Material Inspired Organic Thin-Film Transistors for Biosensing: Properties and Applications, ACS Mater. Lett., 2022, 4, 918–937, DOI:10.1021/acsmaterialslett.2c00095
.
- J. Lv, J. Chen and P. S. Lee, Sustainable Wearable Energy Storage Devices Self-charged by Human-body Bioenergy, SusMat, 2021, 1, 285–302, DOI:10.1002/sus2.14
.
- J. Xiong, Y. Li, D. Gao, J. Chen, K. Parida and P. S. Lee, Reconfigurable Origami Transparent Cellulose Triboelectric Paper for Multi-Modal Energy Harvesting, ChemNanoMat, 2022, 8, e202200034, DOI:10.1002/cnma.202200034
.
- A. Grebenko, A. Bubis, K. Motovilov, V. Dremov, E. Korostylev, I. Kindiak, F. S. Fedorov, S. Luchkin, Y. Zhuikova, A. Trofimenko, G. Filkov, G. Sviridov, A. Ivanov, J. T. Dull, R. Mozhchil, A. Ionov, V. Varlamov, B. P. Rand, V. Podzorov and A. G. Nasibulin, Green Lithography for Delicate Materials, Adv. Funct. Mater., 2021, 31, 2101533, DOI:10.1002/adfm.202101533
.
- D. S. Bajwa, G. Pourhashem, A. H. Ullah and S. G. Bajwa, A Concise Review of Current Lignin Production, Applications, Products and Their Environment Impact, Ind. Crops Prod., 2019, 139, 111526, DOI:10.1016/j.indcrop.2019.111526
.
- N. A. Sagar, S. Pareek, S. Sharma, E. M. Yahia and M. G. Lobo, Fruit and Vegetable Waste: Bioactive Compounds, Their Extraction, and Possible Utilization, Compr. Rev. Food Sci. Food Saf., 2018, 17, 512–531, DOI:10.1111/1541-4337.12330
.
- D. H. Kim, J. Viventi, J. J. Amsden, J. Xiao, L. Vigeland, Y. S. Kim, J. A. Blanco, B. Panilaitis, E. S. Frechette, D. Contreras, D. L. Kaplan, F. G. Omenetto, Y. Huang, K. C. Hwang, M. R. Zakin, B. Litt and J. A. Rogers, Dissolvable Films of Silk Fibroin for Ultrathin Conformal Bio-Integrated Electronics, Nat. Mater., 2010, 9, 511–517, DOI:10.1038/nmat2745
.
- S. Dubey and A. Roulin, Evolutionary and Biomedical Consequences of Internal Melanins, Pigm. Cell Melanoma Res., 2014, 27, 327–338, DOI:10.1111/pcmr.12231
.
- C. J. Bettinger, J. P. Bruggeman, A. Misra, J. T. Borenstein and R. Langer, Biocompatibility of Biodegradable Semiconducting Melanin Films for Nerve Tissue Engineering, Biomaterials, 2009, 30, 3050–3057, DOI:10.1016/j.biomaterials.2009.02.018
.
- M. Piacenti-Silva, A. A. Matos, J. V. Paulin, R. A. da S. Alavarce, R. C. de Oliveira and C. F. Graeff, Biocompatibility Investigations of Synthetic Melanin and Melanin Analogue for Application in Bioelectronics, Polym. Int., 2016, 65, 1347–1354, DOI:10.1002/pi.5192
.
- T. Eom, J. Jeon, S. Lee, K. Woo, J. E. Heo, D. C. Martin, J. J. Wie and B. S. Shim, Naturally Derived Melanin Nanoparticle Composites with High Electrical Conductivity and Biodegradability, Part. Part. Syst. Charact., 2019, 36, 1900166, DOI:10.1002/ppsc.201900166
.
- E. Di Mauro, D. Rho and C. Santato, Biodegradation of Bio-Sourced and Synthetic Organic Electronic Materials towards Green Organic Electronics, Nat. Commun., 2021, 12, 3167, DOI:10.1038/s41467-021-23227-4
.
- Y. Wang, T. Li, P. Ma, H. Bai, Y. Xie, M. Chen and W. Dong, Simultaneous Enhancements of UV-Shielding Properties and Photostability of Poly(Vinyl Alcohol) via Incorporation of Sepia Eumelanin, ACS Sustain. Chem. Eng., 2016, 4, 2252–2258, DOI:10.1021/acssuschemeng.5b01734
.
- Y. Liang, E. Pakdel, M. Zhang, L. Sun and X. Wang, Photoprotective Properties of Alpaca Fiber Melanin Reinforced by Rutile TiO2 Nanoparticles: A Study on Wool Fabric, Polym. Degrad. Stab., 2019, 160, 80–88, DOI:10.1016/j.polymdegradstab.2018.12.006
.
- E. Di Mauro, M. Camaggi, N. Vandooren, C. Bayard, J. De Angelis, A. Pezzella, B. Baloukas, R. Silverwood, A. Ajji, C. Pellerin and C. Santato, Eumelanin for Nature-Inspired UV-Absorption Enhancement of Plastics, Polym. Int., 2019, 68, 984–991, DOI:10.1002/pi.5790
.
- A. Tripathi and J. S. Melo, Synthesis of a Low-Density Biopolymeric Chitosan- Agarose Cryomatrix and Its Surface Functionalization with Bio-Transformed Melanin for the Enhanced Recovery of Uranium(VI) from Aqueous Subsurfaces, RSC Adv., 2016, 6, 37067–37078, 10.1039/c6ra04686j
.
- G. Panzarasa, A. Osypova, G. Consolati, F. Quasso, G. Soliveri, J. Ribera and F. W. M. R. Schwarze, Preparation of a Sepia Melanin and Poly(Ethylene-Alt-Maleic Anhydride) Hybrid Material as an Adsorbent for Water Purification, Nanomaterials, 2018, 8, 54, DOI:10.3390/nano8020054
.
- M. Lindroos, D. Hörnström, G. Larsson, M. Gustavsson and A. J. A. van Maris, Continuous Removal of the Model Pharmaceutical Chloroquine from Water Using Melanin-Covered Escherichia Coli in a Membrane Bioreactor, J. Hazard. Mater., 2019, 365, 74–80, DOI:10.1016/j.jhazmat.2018.10.081
.
- V. Ball, I. Nguyen, M. Haupt, C. Oehr, C. Arnoult, V. Toniazzo and D. Ruch, The Reduction of Ag+ in Metallic Silver on Pseudomelanin Films Allows for Antibacterial Activity but Does Not Imply Unpaired Electrons, J. Colloid Interface Sci., 2011, 364, 359–365, DOI:10.1016/j.jcis.2011.08.038
.
- M. Araújo, R. Viveiros, A. Philippart, M. Miola, S. Doumett, G. Baldi, J. Perez, A. R. Boccaccini, A. Aguiar-Ricardo and E. Verné, Bioactivity, Mechanical Properties and Drug Delivery Ability of Bioactive Glass-Ceramic Scaffolds Coated with a Natural-Derived Polymer, Mater. Sci. Eng., C, 2017, 77, 342–351, DOI:10.1016/j.msec.2017.03.169
.
- A. De Trizio, P. Srisuk, R. R. Costa, A. G. Fraga, T. Modena, I. Genta, R. Dorati, J. Pedrosa, B. Conti, V. M. Correlo and R. L. Reis, Natural Based Eumelanin Nanoparticles Functionalization and Preliminary Evaluation as Carrier for Gentamicin, React. Funct. Polym., 2017, 114, 38–48, DOI:10.1016/j.reactfunctpolym.2017.03.004
.
- M. Kim, H. S. Kim, M. A. Kim, H. Ryu, H. J. Jeong and C. M. Lee, Thermohydrogel Containing Melanin for Photothermal Cancer Therapy, Macromol. Biosci., 2017, 17, 1600371, DOI:10.1002/mabi.201600371
.
- M. A. Kim, S. Do Yoon, E. M. Kim, H. J. Jeong and C. M. Lee, Natural Melanin-Loaded Nanovesicles for near-Infrared Mediated Tumor Ablation by Photothermal Conversion, Nanotechnology, 2018, 29, 415101, DOI:10.1088/1361-6528/aad4da
.
- Q. Jiang, Y. Liu, R. Guo, X. Yao, S. Sung, Z. Pang and W. Yang, Erythrocyte-Cancer Hybrid Membrane-Camouflaged Melanin Nanoparticles for Enhancing Photothermal Therapy Efficacy in Tumors, Biomaterials, 2019, 192, 292–308, DOI:10.1016/j.biomaterials.2018.11.021
.
- Y. Yuan, F. Wu, F. Zhang, X. Li, X. Wu and J. Fu, Hepatoenteric Protective Effect of Melanin from Inonotus Hispidus on Acute Alcoholic Liver Injury in Mice, Mol. Nutr. Food Res., 2023, 67, 2200562, DOI:10.1002/mnfr.202200562
.
- X. Wang, J. Zhang, L. Yang, T. Wang, G. Duan, Z. Gu and Y. Li, Eumelanin-like Poly(Levodopa) Nanoscavengers for Inflammation Disease Therapy, Biomacromolecules, 2024, 25, 2563–2573, DOI:10.1021/acs.biomac.4c00092
.
- R. Xu, A. Gouda, M. F. Caso, F. Soavi and C. Santato, Melanin: A Greener Route To Enhance Energy Storage under Solar Light, ACS Omega, 2019, 4, 12244–12251, DOI:10.1021/acsomega.9b01039
.
- Y. J. Kim, W. Wu, S. Chun, J. F. Whitacre and C. J. Bettinger, Biologically Derived Melanin Electrodes in Aqueous Sodium-Ion Energy Storage Devices, Proc. Natl. Acad. Sci. U. S. A., 2013, 110, 20912–20917, DOI:10.1073/pnas.1314345110
.
- Y. J. Kim, W. Wu, S. E. Chun, J. F. Whitacre and C. J. Bettinger, Catechol-Mediated Reversible Binding of Multivalent Cations in Eumelanin Half-Cells, Adv. Mater., 2014, 26, 6572–6579, DOI:10.1002/adma.201402295
.
- P. Kumar, E. Di Mauro, S. Zhang, A. Pezzella, F. Soavi, C. Santato and F. Cicoira, Melanin-Based Flexible Supercapacitors, J. Mater. Chem. C, 2016, 4, 9516–9525, 10.1039/c6tc03739a
.
- J. V. Paulin, S. L. Fernandes and C. F. O. Graeff, Solid-State Electrochemical Energy Storage Based on Soluble Melanin, Electrochem, 2021, 2, 264–273, DOI:10.3390/electrochem2020019
.
- C. J. Bettinger, Materials Advances for Next-Generation Ingestible Electronic Medical Devices, Trends Biotechnol., 2015, 33, 575–585, DOI:10.1016/j.tibtech.2015.07.008
.
- A. Gouda, A. Masson, M. Hoseinizadeh, F. Soavi and C. Santato, Biosourced Quinones for High-Performance Environmentally Benign Electrochemical Capacitors via Interface Engineering, Commun. Chem., 2022, 5, 98, DOI:10.1038/s42004-022-00719-y
.
- M. Levin, J. Selberg and M. Rolandi, Endogenous Bioelectrics in Development, Cancer, and Regeneration: Drugs and Bioelectronic Devices as Electroceuticals for Regenerative Medicine, iScience, 2019, 22, 519–533, DOI:10.1016/j.isci.2019.11.023
.
- M. Reali, P. Saini and C. Santato, Electronic and Protonic Transport in Bio-Sourced Materials: A New Perspective on Semiconductivity, Mater. Adv., 2021, 2, 15–31, 10.1039/d0ma00579g
.
- J. V. Paulin, M. P. Pereira, B. A. Bregadiolli, J. P. Cachaneski-Lopes, C. F. O. Graeff and C. C. B. Bufon, Controlling Ions and Electrons in Aqueous Solution: An Alternative Point of View of the Charge-Transport Behavior of Eumelanin-Inspired Material, J. Mater. Chem. C, 2023, 11, 6107–6118, 10.1039/D3TC00490B
.
- E. Kim, Z. Wang, J. W. Phua, W. E. Bentley, E. Dadachova, A. Napolitano and G. F. Payne, Enlisting Electrochemistry to Reveal Melanin's Redox-Related Properties, Mater. Adv., 2024, 5, 3082–3093, 10.1039/d3ma01161e
.
- M. Piacenti-Silva, J. C. Fernandes, N. B. de Figueiredo, M. Congiu, M. Mulato and C. F. de O. Graeff, Melanin as an Active Layer in Biosensors, AIP Adv., 2014, 4, 037120, DOI:10.1063/1.4869638
.
- M. Sheliakina, A. B. Mostert and P. Meredith, An All-Solid-State Biocompatible Ion-to-Electron Transducer for Bioelectronics, Mater. Horiz., 2018, 5, 256–263, 10.1039/C7MH00831G
.
- Z. Tehrani, S. P. Whelan, B. Mostert, J. V. Paulin, M. M. Ali, E. D. Ahmadi, C. F. de O. Graeff, O. J. Guy and D. T. Gethin, Printable and Flexible Graphene PH Sensors Utilising Thin Film Melanin for Physiological Applications, 2D Mater., 2020, 7, 024008, DOI:10.1088/2053-1583/ab72d5
.
- A. Wahab, N. Gogurla, J. Y. Park and S. Kim, Architecting Silk Protein and Melanin for Photoresponsive and Self-Healable Optoelectronic Skins, Adv. Mater. Technol., 2022, 7, 2101271, DOI:10.1002/admt.202101271
.
- S. P. Whelan, Z. Tehrani, M. Peacock, J. V. Paulin, O. Guy and D. Gethin, Investigation into the Suitability of Screen Printed Graphene-Melanin PH Sensors for Use in Bacterial Culturing Applications, J. Electroanal. Chem., 2022, 904, 115868, DOI:10.1016/j.jelechem.2021.115868
.
- L. P. da Silva, S. Oliveira, R. P. Pirraco, T. C. Santos, R. L. Reis, A. P. Marques and V. M. Correlo, Eumelanin-Releasing Spongy-like Hydrogels for Skin Re-Epithelialization Purposes, Biomed. Mater., 2017, 12, 025010, DOI:10.1088/1748-605X/aa5f79
.
- Z. Lin, L. Liu, W. Wang, L. Jia, Y. Shen, X. Zhang, D. Ge, W. Shi and Y. Sun, The Role and Mechanism of Polydopamine and Cuttlefish Ink Melanin Carrying Copper Ion Nanoparticles in Antibacterial Properties and Promoting Wound Healing, Biomater. Sci., 2021, 9, 5951–5964, 10.1039/d1bm00622c
.
- D. Biyashev, Z. E. Siwicka, U. V. Onay, M. Demczuk, D. Xu, M. K. Ernst, S. T. Evans, C. V. Nguyen, F. A. Son, N. K. Paul, N. C. McCallum, O. K. Farha, S. D. Miller, N. C. Gianneschi and K. Q. Lu, Topical Application of Synthetic Melanin Promotes Tissue Repair, npj Regener. Med., 2023, 8, 61, DOI:10.1038/s41536-023-00331-1
.
- M. Sheliakina, A. B. Mostert and P. Meredith, Decoupling Ionic and Electronic Currents in Melanin, Adv. Funct. Mater., 2018, 28, 1805514, DOI:10.1002/adfm.201805514
.
- M. Reali, A. Gouda, J. Bellemare, D. Ménard, J.-M. Nunzi, F. Soavi and C. Santato, Electronic Transport in the Biopigment Sepia Melanin, ACS Appl. Bio Mater., 2020, 3, 5244–5252, DOI:10.1021/acsabm.0c00373
.
- J. V. Paulin, S. Bayram, C. F. O. Graeff and C. C. B. Bufon, Exploring the Charge Transport of a Natural Eumelanin for Sustainable Technologies, ACS Appl. Bio Mater., 2023, 6, 3633–3637, DOI:10.1021/acsabm.3c00469
.
- L. Lu, C. Jiang, G. Hu, J. Liu and B. Yang, Flexible Noncontact Sensing for Human–Machine Interaction, Adv. Mater., 2021, 33, 2100218, DOI:10.1002/adma.202100218
.
- J. V. Paulin, L. G. S. Albano, D. H. S. Camargo, M. P. Pereira, B. A. Bregadiolli, C. F. O. Graeff and C. C. B. Bufon, Eumelanin-Based Multisensory Platform: A Case of Study for Photolithographic Patterning, Appl. Mater. Today, 2022, 28, 101525, DOI:10.1016/j.apmt.2022.101525
.
- A. B. M. Mostert, the What, the Why and the How: An Introductory Review for Materials Scientists Interested in Flexible and Versatile Polymers, Polymers, 2021, 13, 1670, DOI:10.3390/polym13101670
.
- S. Bayram, C. Dengiz, Y. C. Gerçek, I. Cetin and M. R. Topcul, Bioproduction, Structure Elucidation and in Vitro Antiproliferative Effect of Eumelanin Pigment from Streptomyces Parvus BSB49, Arch. Microbiol., 2020, 202, 2401–2409, DOI:10.1007/s00203-020-01956-2
.
-
J. W. Phua and C. J. H. Ottenheim, A Method for Obtaining Melanin from Invertebrate Biomass and the Product Obtained Therefrom, US Pat. 20230127563A1, 2023 Search PubMed
.
- J. V. Paulin, A. G. Veiga, Y. Garcia-Basabe, M. L. M. Rocco and C. F. Graeff, Structural and Optical Properties of Soluble Melanin Analogues with Enhanced Photoluminescence Quantum Efficiency, Polym. Int., 2018, 67, 550–556, DOI:10.1002/pi.5543
.
- J. V. Paulin, A. P. Coleone, A. Batagin-Neto, G. Burwell, P. Meredith, C. F. O. Graeff and A. B. Mostert, Melanin Thin-Films: A Perspective on Optical and Electrical Properties, J. Mater. Chem. C, 2021, 9, 8345–8358, 10.1039/D1TC01440D
.
- B. A. Bregadiolli, J. V. Paulin, L. G. S. Albano, L. M. Martins, D. H. S. de Camargo, L. C. da S. Filho, C. C. B. Bufon and C. F. de O. Graeff, A Strategy towards Melanin-Based Functional Material: RGO and Sulfonated Melanin Composites, J. Mater. Chem. C, 2021, 9, 16991–17002, 10.1039/D1TC02749B
.
- S. N. Dezidério, C. A. Brunello, M. I. N. da Silva, M. A. Cotta and C. F. O. Graeff, Thin Films of Synthetic Melanin, J. Non-Cryst. Solids, 2004, 338–340, 634–638, DOI:10.1016/j.jnoncrysol.2004.03.058
.
- S. R. Cicco, M. Ambrico, P. F. Ambrico, M. M. Talamo, A. Cardone, T. Ligonzo, R. Di Mundo, C. Giannini, T. Sibillano, G. M. Farinola, P. Manini, A. Napolitano, V. Criscuolo and M. D'Ischia, A Water-Soluble Eumelanin Polymer with Typical Polyelectrolyte Behaviour by Triethyleneglycol N-Functionalization, J. Mater. Chem. C, 2015, 3, 2810–2816, 10.1039/C4TC01997K
.
- A. Pezzella, M. Barra, A. Musto, A. Navarra, M. Alfè, P. Manini, S. Parisi, A. Cassinese, V. Criscuolo and M. D'Ischia, Stem Cell-Compatible Eumelanin Biointerface Fabricated by Chemically Controlled Solid State Polymerization, Mater. Horiz., 2015, 2, 212–220, 10.1039/c4mh00097h
.
- E. S. Bronze-Uhle, J. V. Paulin, M. Piacenti-Silva, C. Battocchio, M. L. M. Rocco and C. F. de O. Graeff, Melanin Synthesis under Oxygen Pressure, Polym. Int., 2016, 65, 1339–1346, DOI:10.1002/pi.5185
.
- R. Micillo, M. Iacomino, M. Perfetti, L. Panzella, K. Koike, G. D'Errico, M. D'Ischia and A. Napolitano, Unexpected Impact of Esterification on the Antioxidant Activity and (Photo)Stability of a Eumelanin from 5,6-Dihydroxyindole-2-Carboxylic Acid, Pigm. Cell Melanoma Res., 2018, 31, 475–483, DOI:10.1111/pcmr.12689
.
|
This journal is © The Royal Society of Chemistry 2024 |
Click here to see how this site uses Cookies. View our privacy policy here.