DOI:
10.1039/D3QM00523B
(Review Article)
Mater. Chem. Front., 2024,
8, 248-264
Recent progress on MRI probes for metal ion detection and biological applications
Received
5th May 2023
, Accepted 23rd September 2023
First published on 3rd October 2023
Abstract
The disorder of metal ion levels can have a significant impact on the ecological environment and human health due to their crucial role in complex biological metabolism processes and the overall ecological balance. Traditional detection technologies for various metal ions have been successfully developed. However, these traditional methods have limitations when it comes to monitoring the physiological distribution of metal ions with high sensitivity. Magnetic resonance imaging (MRI), known for its ability to provide deep tissue imaging with excellent spatiotemporal resolution, has been widely adopted in biomedical and clinical diagnostics. Interestingly, numerous studies have focused on designing and developing MRI nanoprobes specifically for the detection of metal ions. In this review, we highlight the research progress, limitations, and remaining challenges in the development of metal ion detection probes based on MRI technology.
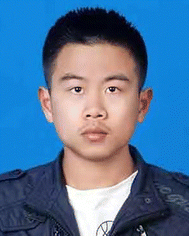
Zhiming Deng
| Zhiming Deng received his degrees (PhD in 2022) in physics from the Key Laboratory of Low-dimensional Quantum Structures and Quantum Control of Ministry of Education, Hunan Normal University. He is currently doing postdoctoral research in the College of Chemistry and Chemical Engineering at Hunan University under the supervision of Prof. Guosheng Song. His research is focused on the design and synthesis of functional nanomaterials for biomedical applications. |
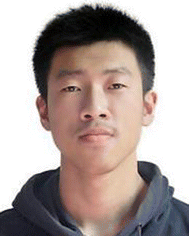
Dingyou Lu
| Dingyou Lu is currently a postgraduate in the College of Chemistry and Chemical Engineering at Hunan University under the supervision of Prof. Guosheng Song. He received his BS degree from Hunan University in 2022. His current research focuses on designing MRI nanomaterials for bioimaging and cancer therapy. |
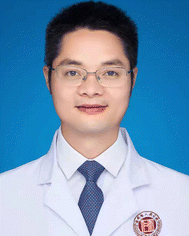
Sulai Liu
| Sulai Liu is an associate chief physician at Hunan Provincial People's Hospital (The First Affiliated Hospital of Hunan Normal University) in China. He received his PhD degree from Central South University in 2016. His research focuses on hepatocellular carcinoma, cholangiocarcinoma, and immunotherapy. |
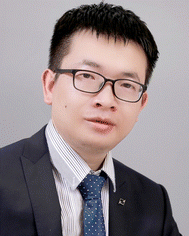
Xianzheng Tan
| Dr Xian-Zheng Tan is an associate chief physician of radiology at Hunan Provincial People's Hospital, the First Affiliated Hospital of Hunan Normal University in China. His research interests are focused on artificial intelligence applications in hepatobiliary-pancreas imaging. |
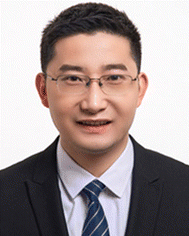
Guosheng Song
| Guosheng Song is a professor at Hunan University in China. He received his PhD degree from Donghua University in 2014. He carried out postdoctoral research at Soochow University and then at Stanford University from 2014 to 2018. In 2018, he joined the College of Chemistry and Chemical Engineering, Hunan University. His research mainly focuses on designing activatable nanoplatforms for multimode imaging and cancer specific therapy. |
1. Introduction
Metal ions, such as Zn2+, Ca2+, K+, Mg2+, Cu2+, Pb2+, Mn2+, Fe2+/3+, and Cd2+, play a crucial role in numerous biological processes, including cellular functions, osmotic regulation, molecular structures, and maintaining water and electrolyte balance in organisms.1 It is essential to maintain appropriate concentrations of metal ions for the appropriate functioning of biological systems. Additionally, metal ions are distributed unevenly across various organs,2 further emphasizing the importance of tightly controlling their levels to maintain normal physiological processes. Consequently, there is growing interest in non-invasive quantitative analysis of metal ion levels in vivo.
Recent advancements in life sciences have revealed associations between metabolic disorders of metal ion levels and conditions such as Wilson's disease,3 diabetes,4 and malignant tumors.5 For instance, Wilson's disease is a congenital disorder of copper metabolism, characterized by the presence of excessive free copper ions in the patient's urine. Metal-mediated oxidative stress also contributes to tumor growth and abnormal angiogenesis in malignant tumors.6 These findings highlight the urgent need for a novel imaging tool capable of quantitatively monitoring and spatially detecting metal ion levels in vivo. While there are various technologies available for metal ion detection, such as photoacoustic imaging,7 optical imaging,8–10 and ICP-MS detection,11 these methods can be costly and involve complex operational processes. Therefore, there is a pressing demand for the development of novel imaging technology specifically designed for non-invasive monitoring of metal ion levels in deep tissues.
Magnetic resonance imaging (MRI)12–19 is a non-invasive imaging method commonly used in clinical diagnostics for detecting disorders and monitoring treatment outcomes. It has proven particularly useful in high-resolution imaging of soft tissues, making it a valuable tool in biomedical research and clinical diagnostics. In recent years, the field of MRI has made significant progress, offering capabilities for high-resolution anatomical imaging and monitoring. Several MRI contrast strategies have been developed, including well-designed probes for various purposes, such as tracking therapeutic cells, imaging enzyme activity, and regulating gene expression. Numerous MRI nanoprobes have also been designed for the detection of metal ions, including Zn2+,20,21 Ca2+,22 K+,23 Mg2+,23 Cu2+,24–27 and heavy metal ions28 such as Pb2+ and Cd2+.
While previous reviews on metal ion monitoring have focused on optical imaging,29 colorimetric techniques,30 and photoacoustic imaging,31 there is limited research summarizing the use of MRI for systematic and comprehensive metal ion detection. This review aims to provide a detailed introduction to various metal ion detection methods based on MRI imaging technology (Scheme 1). It will cover recent progress in metal ion detection and highlight the key challenges for future applications.
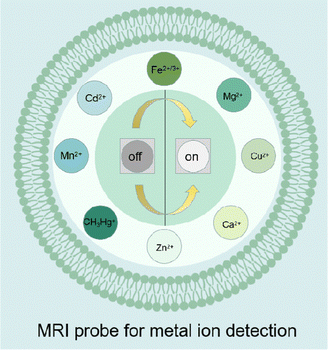 |
| Scheme 1 Schematic illustration of MRI probes for the detection of various metal ions in vitro and in vivo. | |
2. Design of MRI-based probes for metal ion detection
Metal ion-responsive MRI probes are developed to detect specific metal ions by altering MRI signals through the interaction between the targets and the probes. There are four main types of metal ion-responsive MRI probes.32
1.
Metal ion-identification moiety and MRI contrast agent
This approach combines a metal ion-identification moiety with an MRI contrast agent for ion-specific detection. The contrast agent interacts with water molecules when the ion receptor binds to the metal ion, leading to changes in relaxation times (Fig. 1A).
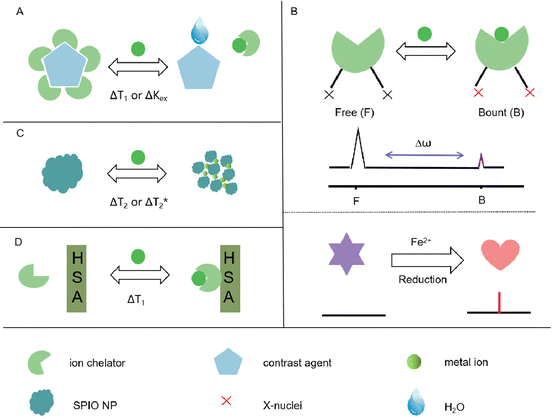 |
| Fig. 1 Schematic illustration of MRI probes for metal ion detection. (A) The mechanism for metal ion detection based on T1 contrast agents. (B) Sensing metal ions using a heteronuclear sensor. (C) The mechanism for metal ion detection based on SPIO NP. (D) The mechanism of MRI metal ion detection based on HSA. | |
2.
19F-MRI probes
Small-molecule-based 19F-MRI probes can be classified into two types based on their 19F signal readout: off–on and chemical shift change. Off–on probes use paramagnetic metal ions as quenchers to switch off the 19F signal, which can be de-quenched by cleavage or changes in the redox states of the quenchers in response to the analytes of interest (Fig. 1B).
3. Fe3O4 nanoparticles
Fe3O4 nanoparticles, also known as superparamagnetic iron oxide (SPIO) nanoparticles, can influence T1/T2 signal changes through a self-assembly strategy (Fig. 1C).
4. Contrast agents with increased relaxivity
Some contrast agents exhibit increased relaxivity in the presence of metal ions by forming a ternary complex with human serum albumin (HSA) (Fig. 1D).
These different approaches offer diverse mechanisms for metal ion detection and enable label-free MRI probes when non-1H nuclei are involved. By utilizing these strategies, researchers can design metal ion-responsive MRI probes for specific detection and imaging applications.
3. Detection of metal ions
3.1. MRI probe for Zn2+ ion monitoring
With its involvement in metalloenzyme catalysis, signal transduction, DNA binding, and gene expression, Zn2+ is crucial for the survival of organisms. The majority of Zn2+ ions in biological systems are firmly bound by proteins.33 However, Zn2+ abnormalities have been linked to various physical growth retardations and neurological conditions, including Alzheimer's disease and cerebral ischemia. To detect Zn2+ levels using MR imaging techniques, several MRI probes have been designed.
For instance, Sherry and co-workers34 pioneered the design of a novel probe based on GdDOTA-diBPEN (Fig. 2A). This probe has a high detection limit (about 30 μM) for Zn2+ ion detection in the presence of HSA. The probe's relaxivity (r1) increased from 5.0 ± 0.1 mM−1 s−1 to 6.0 ± 0.1 mM−1 s−1 in the presence of Zn2+, making it suitable for detecting free Zn2+ ions in vivo without interfering with other biological processes involving Zn2+. In this case, GdDOTA-diBPEN's relaxivity rose by around three times, and the agent's binding to HSA was extremely strong. Next, in order to maximize the r1 of the Zn2+-responsive probes, a series of MRI probes35 (Fig. 2B) were designed to fine-tune the rate of water exchange from the inner sphere of Gd. Especially for Gd-4 and Gd-5, which were up to 50 mM−1 s−1 for r1 relaxivity values upon binding to Zn(ii) and HSA.
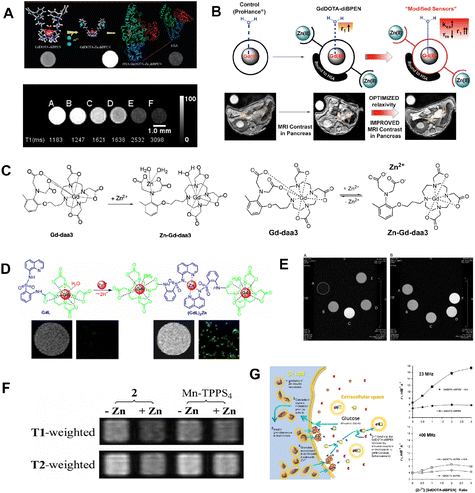 |
| Fig. 2 (A) Hypothetical structures and T1-weighted phantom MR images of GdDOTA-diBPEN in the presence and absence of Zn2+. Reproduced from ref. 34 with permission from American Chemical Society, copyright 2009. (B) Increasing Zinc(ii) sensitivity MRI contrast agents with responsive water exchange rates. Reproduced from ref. 35 with permission from American Chemical Society, copyright 2015. (C) Proposed mechanism of a Zn2+-activated Gd-daa3 contrast agent. Reproduced from ref. 36 with permission from American Chemical Society, copyright 2008. Reproduced from ref. 37 with permission from NATL ACAD SCIENCES, copyright 2007. (D) GdL binding mode to Zn2+ and confocal fluorescence pictures in MDA-MB-231 cells after incubation with Zn2+. Reproduced from ref. 38 with permission from American Chemical Society, copyright 2012. (E) Optimized geometry of the SAP isomers of GdZnL2+ and GdHL+. Reproduced from ref. 39 with permission from American Chemical Society, copyright 2015. (F) Zn2+-induced T1/T2 signal change in solution. Reproduced from ref. 40 with permission from NATL ACAD SCIENCES, copyright 2007. (G) The steps involved in insulin and Zn2+ release from-cells during glucose-stimulated insulin secretion are depicted schematically. Reproduced from ref. 41 with permission from NATL ACAD SCIENCES, copyright 2011. | |
For other Zn2+ detection probes, Meade and co-workers36,37(Fig. 2C) designed double kinds of Zn2+-responsive GdDO3A-based agents containing either an iminodiacetate or a pyridine substituent for specific Zn2+ binding. The advantage of those probes relies on the fact that upon Zn2+ binding, the Gd3+ center becomes accessible for water molecule exchange, resulting in increased relativity and a high detection limit for Zn2+ detection. To further verify the biosafety of the designed probe, two kinds of cells were treated with 0.5 mM Gd-daa3 probes, which showed no observable cell apoptosis. Cells proliferated exponentially at the expected rate, indicating low toxicity from the agent. These results provide support for potential in vivo studies in the future. Therefore, dual imaging probes are urgently being developed to improve the specificity of Zn2+ ion detection. Following these efforts to develop MRI based probes to monitor Zn2+ in biology, a Zn2+-responsive bimodal probe GdL based on Gd-DO3A bound to 8-sulfonamidoquinoline was proposed.38 As the fluorescence increases, the r1 of GdL also increases from 3.8 mM−1 s−1 to 5.9 mM−1 s−1 in the presence of Zn2+(Fig. 2D). In the physiological pH range, the Zn2+ responsive imaging probe exhibits favorable selectivity and tolerance over a range of biologically significant anions and metal ions for both relaxivity and luminescence. In order to improve the contrast performance of Zn2+ detection, a DO3A unit for Gd3+ chelate was linked to an NO2A moiety for Zn2+ binding.39 Obviously, the relativity of the obtained sensor was increased by 150% at a high magnetic field B0 (7.0 T) in the presence of Zn2+ (Fig. 2E). Although other strategies for improving Zn2+ detection sensitivity were proposed, none of the mentioned probes have been further used in vivo.
For further in vivo detection of Zn2+, Lippard and coworkers designed an alternative scaffold called Mn-(DPA-C2)2-TPPS3 that uses Zn2+-selective binding units coupled to a water-soluble porphyrin template to make it easier to utilize probes to detect fluctuating Zn2+ levels in biological systems.40 As shown in Fig. 2F, the zinc-containing solution presented a weaker signal than the zinc-free sample, and the reference Mn-TPPS4 showed no zinc-induced T2 change. This Mn-porpyrin probe has several advantages over the Gd-based probes stated above, including the ability to be detected by both MRI and optical modalities. Recently, the high-affinity Zn2+ agent, GdL1, and the low-affinity Zn2+ agent, GdL2, were described as improved Zn2+-responsive MRI contrast agents41 for visualization of beta-cell function (Fig. 2G). Deep tissue monitoring of beta-cell activity in vivo during type 2 diabetes progression or following islet implantation in type I diabetic patients is an attractive prospective use for these MRI probes.
Recently, in order to distinguish Zn2+ from other abundant endogenous metal ions, a highly effective MRI contrast agent based on paramagnetic Gd3+ has been created.42 Its longitudinal relaxivity significantly increases as a result of the interaction with Zn2+, which modifies the hydration of the complex. An experiment using tube phantoms indicated the potential of this compound to function as an MRI contrast agent. Six tubes containing GdL alone or with various metal ions were photographed. As demonstrated in Fig. 3A, tubes containing GdL and Zn2+ present a significant increase in MR signal strength, but tubes containing Ca2+ or Mg2+ had no discernible change. They designed a novel responsive platform that is ideal for exploring Zn-related physiological processes in high magnetic fields. Bar-Shir et al. recently developed innovative probes for the detection of Zn2+ in the brain.43 A strong 19FiCEST effect was achieved, which was depicted as a Zn2+ map superimposed on a 1H-MRI anatomical image, as expected from a labile-zinc-rich ROI. In contrast, no discernible difference between the two 19F-MRI data sets could be shown, and the resulting 19F-iCEST exhibited no signal when the probe was administered to an area that was not anticipated to contain large levels of labile Zn2+ (Fig. 3B). As we all know, a healthy prostate has the highest concentration of Zn2+. Due to the fact that this level drops dramatically during the early stages of prostate cancer, in vivo detection of prostate zinc content may be used for prostate cancer diagnosis.44 There was a distinct difference in the 19F-iCEST signal before and after D-glucose injection, indicating the presence of a normal prostate. In the future, in order to achieve more accurate Zn2+ ion detection, the sensitivity and specificity can be further improved. Achieving good biocompatibility is a primary goal for clinical applications of materials. Most probes are mainly organic and face issues such as poor stability. It is necessary to develop stable and specific probes that are not affected by other metal ions.
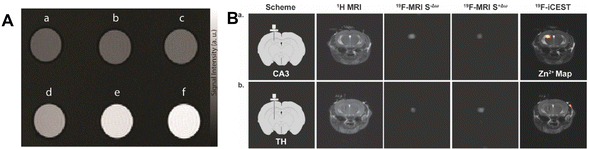 |
| Fig. 3 (A) The T1-weighted MR images of GdL treated with different metal ions. Reproduced from ref. 42 with permission from Willey VCH, copyright 2021. (B) In vivo19F-iCEST maps of Zn2+ in the mouse brain. Reproduced from ref. 43 with permission from American Chemical Society, copyright 2021. | |
It should be pointed out that MRI probes for Zn2+ detection often lack the required sensitivity to detect low concentrations of Zn2+. This can limit their usefulness in applications where high sensitivity is crucial, such as in the detection of Zn2+ related diseases. Meanwhile, MRI probes for Zn2+ detection may also lack specificity, meaning they can interact with other metal ions. This can lead to false-positive results and hinder accurate Zn2+ detection. And some MRI organic molecule probes for Zn2+ detection present poor stability, especially in complex biological environments. This can result in probe degradation or loss of signal over time, reducing their reliability and longevity. Moreover, for Zn2+ detection, crossing cell membranes and accessing intracellular Zn2+ pools is another challenge in studying Zn2+ dynamics within cells. To address these limitations, modifying the probe structure or incorporating specific binding moieties that enhance sensitivity and specificity for Zn2+ detection is feasible. The development of cell-penetrating peptides or nanoparticle-based delivery systems to facilitate probe entry into cells is able to improve the cell permeability of MRI probes for Zn2+ detection. By addressing these challenges and implementing potential solutions, the limitations of MRI probes for Zn2+ detection can be overcome, leading to improved sensitivity, specificity, stability, and cell permeability for a wide range of applications.
3.2. MRI probe for Ca2+ ion monitoring
All types of cells use Ca2+ signaling as a second messenger in signaling pathways to convey information and control many biochemical processes. One of the main focuses of life science research has been to create methods to dynamically analyze the Ca2+ concentration in vivo.45 The dynamic evaluation of Ca2+ is mainly done through calcium optical imaging, which also sparked the creation of numerous synthetic and genetically engineered calcium markers.46,47 Although this technique is capable of imaging Ca2+ signals in vivo, penetration of deep tissues by photon scattering is a major obstacle to its use in large animals and humans. Due to the importance of Ca2+ in the setting of cellular action potentials and its critical role in neuronal activity, researchers developed a non-invasive method to monitor changes in Ca2+ levels in deep neural tissue of living humans. The general comprehension of neuronal activity has greatly benefited from functional MRI investigations,48 but the indirect observation of neuronal function necessitates a direct and quantitative MRI-based method to spatially detect Ca2+ alternations. Therefore, it is highly important to develop an MRI probe for direct observation of Ca2+ levels in vivo.
As mentioned above, designing an MRI probe for monitoring Ca2+ levels could aid in the diagnosis of Ca2+-related diseases. As shown in Fig. 4A, Dhingra et al. designed a Ca2+-sensitive MRI contrast agent that was blocked from inner-sphere water molecules by two APTRA iminoacetates that interacted with the Gd(III) centers.49 As a consequence, the Gd-DOPTRA presents a low relaxivity down to 3.5 mM−1 s−1, and the probe presents a high detection specificity for Ca2+. Next, the group also designed a series of MRI probes (GdL1–GdL4) by combining the DO3A-Gd(III) complex with various chelators (Fig. 4B).50 In these designed DO3A-Gd(III) complexes, the GdL1 complexes were found to be insensitive to Ca2+. When similar r1 alterations were produced by the addition of other metal ions, it was discovered that GdL2 and GdL3 were responsive to Ca2+ in a variety of concentration ranges with poor selectivity. Interestingly, in a relaxometric titration experiment, the r1 of GdL4 was dramatically raised by 63% upon binding with Ca2+.
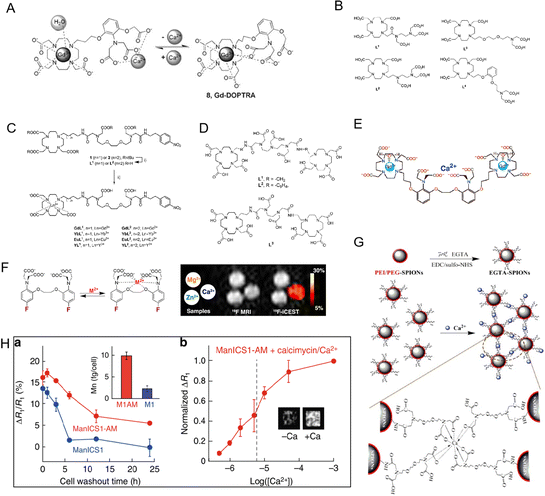 |
| Fig. 4 (A) The process in equilibrium with Ca2+ of Gd-DOPTRA. Reproduced from ref. 49 with permission from The Royal Society of Chemistry, copyright 2008. (B) The structures of the investigated ligand L1–L4. Reproduced from ref. 50 with permission from The Royal Society of Chemistry, copyright 2011.(C) Chemical structures of L1 and L2. Reproduced from ref. 51 with permission from The Royal Society of Chemistry, copyright 2019. (D) Chemical structures of L1, L2 and L3. Reproduced from ref. 53 with permission from American Chemical Society, copyright 2008. (E)–(G) Chemical structures of Ca2+-sensitive MRI contrast agents. Reproduced from ref. 56, 57 and 58 with permission from American Chemical Society, American Chemical Society and Elsevier, copyright 2002, 2013 and 2016, respectively. (H) The Ca2+-dependent MRI signal changes in cells. Reproduced from ref. 59 with permission from The Springer Nature, copyright 2019. | |
Recently, other Ca2+-responsive MRI probes (Fig. 4C, named GdL1 and GdL2) with different linker lengths between the MRI signaling unit and Ca2+-responsive moieties were developed by Angelovski et al.51 The total r1 and r2 relaxivity changes for GdL2 were measured by relaxometric titrations of both probes and were determined to be 131 and 138%, respectively. Greater r1 and r2 relaxivity modifications in GdL2 showed that even small structural variations between the acyclic Ca2+ binding unit and the macrocyclic Gd(III) ligand might result in significantly different relaxation characteristics of the Gd(III) complex. Then, a dual frequency response probe (SCA) to detect Ca2+ by recording 1H and 19F NMR signals based on GdL was developed, in which –NO2 was replaced by the –CF3 group of the benzyl moiety.52 After the addition of Ca2+, the 1H relaxometry measurement of SCAs showed a 2.89-fold increase in r1 relaxivity from 3.18 to 9.20 mM−1 s−1 in contrast to GdL. Interestingly, the signal-to-noise ratio of the 19F NMR signal decreased from 46 to 20 in the presence of Ca2+. Both 1H and 19F NMR signal changes allowed SCAs for dual-frequency Ca2+ responses. The results show that these double-frequency tracers are extremely promising for the creation of new functional MRI techniques.
It should be noted that it was shown to be difficult to track the fluctuation of Ca2+ concentration in the millimolar range using conventional nanoprobes for free Ca2+ concentration in the micromolar range. In view of this, Mishra et al. developed three Ca2+-responsive Ln3+ complex MRI probes (L1, L2, and L3) (Fig. 4D).53 In order to expand the detection range to higher Ca2+ values, the Ca2+ recognition moiety in the center of these ligands has a low affinity for Ca2+. These MRI probes were developed by adding Gd(III)-DO3A-type bismacrocyclic complexes to Ca2+ recognition molecules such as ethylenediaminetetraacetic acid (EDTA) and diethylenetriamine pentaacetic acid (DTPA) bisamides, respectively. Measurements of relaxation titration revealed that the ligand architecture affected how sensitive their relaxivity was toward Ca2+. After chelation with Ca2+, the relaxivity of L1 and L3 increased by 15% and 32%, respectively. Due to the significant distance between the Ca2+ binding site and Gd(III)-DO3A, the poor Ca2+ sensitivity of L2 was attributed to a poorer Ca2+ coordination capacity.
To measure Ca2+ fluctuations, Angelovski and coworkers also designed two calcium-activated MRI probes (Gd2L1 and Gd2L2). The probes Gd2L1 and Gd2L2 were created by combining two DO3A-Gd(III) complexes with the Ca2+-specific chelator.54 In order to lower the Ca2+-association constant of EGTA while maintaining its selectivity for other competing metals, two of its carboxylate groups were converted to amides. Due to the modification of EGTA, the Ca2+ chelating unit demonstrated high flexibility, which is advantageous for Ca2+ detection. It was discovered that the initial relativity was 4.05 and 3.44 mM−1 s−1.
Recently, two monomeric bifunctional chelators that can act as Ca2+-responsive contrast agents were described by Angelovski et al.55 According to the results of the relaxometric nuclear magnetic resonance titrations, there were no detectable changes in the r1 signals of the probe in the presence of Ca2+. Due to the interaction of GdL2 with extracellular Ca2+, the T1-weighted signal intensity of MRI rapidly dropped, reaching up to 5% of the difference in the detrended signal. As shown in Fig. 4E, the Ca2+-sensitive MRI contrast agent, DOPTA-Gd (Gd-1), was designed by Meade and colleagues.56 Two DO3A-Gd chelates are combined to form DOPTA-Gd, which is connected by a modified BAPTA moiety, a well-known selective Ca2+ binder. In the absence of Ca2+, the iminodiacetate groups of the BAPTA linker bind to the Gd3+ centers, producing a relaxivity of 3.26 mM−1 s−1. These groups preferentially bind Ca2+ upon the addition of Ca2+, opening up the coordination sites around the Gd3+ centers to solvent water and causing about 80% rise in relaxivity to 5.76 mM−1 s−1. The selectivity and Ca2+ binding affinity of DOPTA-Gd make it potentially useful for monitoring intracellular Ca2+ in a biological setting. McMahon et al. used M2+-5F-BAPTA probes to detect the signal of Ca2+ even at low Ca2+ concentrations with high sensitivity (Fig. 4F).57 With the simple Ca2+ sensing substance EGTA (ethylene glycol tetraacetic acid), Wu et al. developed a Ca2+-responsive poly (ethylene glycol) and polyethylenimine coated iron oxide nanoparticle (PEI/PEG-SPIONs) MRI contrast agents.58 As shown in Fig. 4G, the Ca2+-induced aggregation of EGTA-SPIONs reduced the T2 weighted MRI signal intensity. When the Ca2+ concentration dropped from 1.2 to 0.8 mM, the T2 values dropped by 25%. Furthermore, Ca2+ is required for signal transduction in almost all cells, where it coordinates activities ranging from embryogenesis to brain function. To solve this issue, Jasanoff et al. designed a novel contrast agent, ManICS1-AM, for monitoring calcium levels in cells by MRI.59 As shown in Fig. 4H, when stimulated with pharmacological reagents or photogenetic tools, ManICS1-AM loaded cells showed changes in MRI contrast. The response ability is consistent with the signal obtained using a fluorescent calcium indicator. Furthermore, depending on the repeated brain activation in response to various stimuli in vivo, Okada et al. developed a specific MRI nanoprobe for endogenous Ca2+ detection,60 which is suitable for tracking extracellular Ca2+ signaling processes. The created probes can diagnose AD quickly in response to changes in Ca2+ level with a high signal-to-noise ratio.
Furthermore, depending on the repeated brain activation in response to various stimuli in vivo, Okada et al. developed a specific MRI nanoprobe for endogenous Ca2+ detection,60 which is suitable for tracking extracellular Ca2+ signaling processes. The created probes can diagnose AD quickly in response to changes in Ca2+ level with a high signal-to-noise ratio. Researchers employed the MaCaReNas in combination with medial forebrain bundle electrical stimulation, a neural activation paradigm often used in addiction research, to investigate MaCaReNas’ capacity to detect more physiological neuronal activation on a quicker timeline. MaCaReNas were injected into the dorsal striatum and subsequently scanned utilizing a block-design paradigm in combination with T2-weighted spin-echo echo-planar imaging. Image contrast alterations were found only when functional MaCaReNas were administered ipsilaterally to the activated medial forebrain bundle, not when contralateral stimulation or calcium-insensitive LCIOs were utilized. All these well-designed MRI probes provided a promising method for Ca2+ monitoring with high specificity. In order to directly monitor neuronal networks and activity, manganese-enhanced MRI was proposed as an alternative or complementary method to fMRI, in which manganese ions (Mn2+) mimic Ca2+ and can be used as MRI contrast agents. However, high Mn2+ concentrations were associated with some toxic side effects. Therefore, a robust, routinely used molecular MR imaging methodology for direct observation of Ca2+ levels is of interest, and because of this several strategies to rationally design and synthesize molecular probes for this purpose are meaningful. Manganese-enhanced MRI has been proposed as an alternate or supplementary technique to MRI for directly monitoring neural networks and activity, in which manganese ions imitate Ca2+ and can be utilized as an MRI contrast agent. High Mn2+ concentrations, on the other hand, were linked to several hazardous adverse effects. As a result, a robust, frequently used molecular MR imaging technology for direct observation of Ca2+ levels is of interest, and numerous methodologies for rationally designing and synthesizing molecular probes for this purpose are being investigated.
3.3. MRI probe for Cu2+ ion monitoring
The copper ions are the third transition metal that is absolutely necessary for human health.61,62 Additionally, copper is necessary for the growth of connective tissue, cellular respiration, and the production of bones.63 However, unusual Cu2+ concentrations may lead to Menkes disease,64 Wilson disease65 and Alzheimer's disease.66 Therefore, it is important to explore highly sensitive probes for Cu2+ level detection in vivo. Instrument-based analytical techniques, such as optical approaches and graphite furnace atomic absorption spectroscopy, are the standard for Cu2+ detection. However, due to their low sensitivity, expensive equipment costs, and need for trained staff, their use is limited during routine detection. Based on this, it is meaningful to design an MRI probe for Cu2+ detection.67,68
Thus, Chang and colleagues designed a Cu2+-sensitive MRI probe with remarkable selectivity.69 A Gd(III) complex was coupled to a pendant iminodiacetate Cu2+ binding site to create the probe. The entry of inner-sphere water was hampered by the interaction of carboxylates with the Gd(III) ion. In order to facilitate the access of inner-sphere water to the Gd(III) center, the pendant carboxylate receptor reduced steric constriction by chelating Cu2+. With the binding of Cu2+, the relaxivity of copper-gad-1 rose from 3.76 to 5.29 mM−1 s−1. Moreover, it was discovered that the Cu2+ binding to the probe was reversible, with the relaxivity returning once the probe-Cu2+ was treated with too much EDTA with super Cu2+ selectivity.
Interestingly, Chang et al. designed a series of copper-activated MRI probes (CG2–CG6) for the detection of copper at different oxidation states.70 The probes were created by joining several thioether-based copper receptors with the Gd(III)-DO3A scaffold through a 2,6-dimethylpyridine linker (Fig. 5A). These probes demonstrated strong Cu2+ or Cu+ selectivity over competing metal ions. Due to the coordination of the pyridyl linker with Gd(III), in the absence of Cu2+, these probes displayed poor proton relaxivity (r1 = 1.2–2.2 mM−1 s−1). As shown in Fig. 5B, the CG2–CG5 responded to Cu+ only, but probe CG6 responded to both Cu+ and Cu2+, leading to a striking increase in relaxivity (Dr1 = 2.3–6.9 mM−1 s−1). The largest “off–on” responses (360% increasement) were seen for these probes (CG2 and CG3) after Cu+ binding. The Cu+ concentrations ranged from 0 to 100 mM, and the T1-weighted image clearly displayed an increase in MRI signals. In order to improve the charge and hydrophilicity of the platform, the probe was created by swapping out the acetate arms with hexanedioate arms. The 2-(ethylthio)-N-(2-(ethylthio)ethyl)ethana mine substituted pyridyl copper recognition unit was joined to a Gd(III)-DO3A complex. With Cu+ binding, the r1 of copper-Gad-7 increased from 2.6 to 11.4 mM−1 s−1 (about 340% enhancement), and this rise in relaxivity is equivalent to the r1 increase of probe CG2. Over competing cations and anions, such as Cu2+, citrate, bicarbonate, phosphate, and lactate anions, the copper-gad-7 probe demonstrated remarkable specificity for Cu+. The probes might be utilized to capture MRI pictures and track changes in Cu+ levels, according to T1-weighted images.
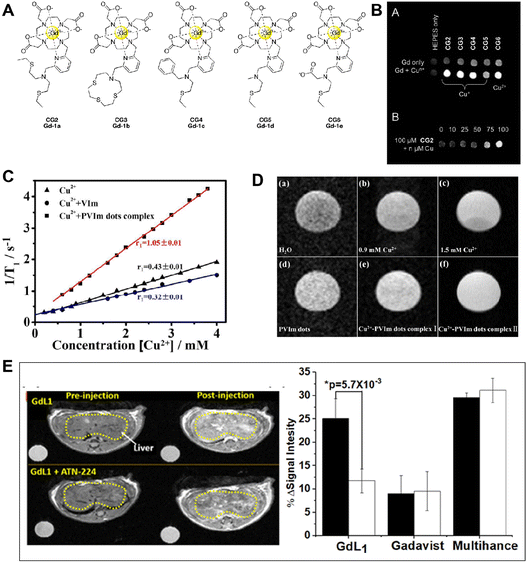 |
| Fig. 5 (A) Molecular structure of CG2–CG6. Reproduced from ref. 70 with permission from The Royal Society of Chemistry, copyright 2009. (B) T1-weighted phantom images of CG2–CG6 with and without Cun+. Reproduced from ref. 70 with permission from The Royal Society of Chemistry, copyright 2009. (C) Plots of 1/T1vs. concentration of Cu2+ added to different probes to form complexes. Reproduced from ref. 72 with permission from American Chemical Society, copyright 2019. (D) MRI T1-weighted images of PVIm dots aqueous solution with different concentrations of Cu2+. Reproduced from ref. 72 with permission from American Chemical Society, copyright 2019. (E) Imaging of free Cu2+in vivo. Reproduced from ref. 73 with permission from The Royal Society of Chemistry, copyright 2019. | |
Recently, Aoki et al. investigated a novel probe based on the nido-carborane derivative 4 bearing N,N,N′-trimethylethylenediamine for the ultrasensitive detection of Cu2+.71 It observed changes in the 11B NMR spectra and MRI images of 4 bearing N,N,N′-trimethylethylenediamine after different concentrations of Cu2+ incubation. In addition, prolonged clearance times are highly expressed on the detection and treatment of serious diseases. For instance, in a single step, Hong Bi and coauthors created PVIm dots with good biocompatibility, hydrophilic properties, and a strong affinity for Cu2+ (Fig. 5C and D).72 This makes it simple to create Cu2+-PVIm dots complexes. It has been effectively shown in vitro that the Cu2+-PVIm dots complexes work well as MRI contrast agents. A novel approach for MRI testing and prolonged renal clearance time can most likely be provided by the PVIm dots. The Cu2+/+-activatable MRI probe discussed here may serve as a promising tool for Cu2+/+-related disease diagnosis. Interestingly, Sherry and co-workers designed a probe named GdL1, which presents high selectivity for Cu2+ with a 43% increase in r1 relaxivity. As shown in Fig. 5E, the T1-weighted imaging demonstrates that GdL1 can detect Cu2+in vivo.73 This is an unprecedented opportunity to investigate the function of Cu2+ in the genesis and progression of neurological illnesses like Wilson's disease.
MRI probes for Cu2+ detection, like Zn2+ probes, may lack the sensitivity necessary to detect low amounts of Cu2+. This can restrict their utility in applications requiring high sensitivity, such as the detection of Cu2+ in biological samples. Background interference from endogenous Cu2+ found in biological samples can make MRI probes for Cu2+ detection vulnerable. This can make distinguishing between external and endogenous Cu2+ signals difficult. Similar to Zn2+ detection, signal amplification methods may be used to improve the sensitivity of Cu2+ detection in MRI probes. To boost the probe's capacity to detect low concentrations of Cu2+, amplification agents or signal enhancement techniques may be used. Methods for suppressing background interference from endogenous Cu2+ in biological samples can be investigated by researchers. This may entail the use of chelating or masking agents that bind to endogenous Cu2+ while minimizing interference with external Cu2+ signals. The limits of MRI probes for Cu2+ detection can be solved by addressing these issues and applying alternative solutions, resulting in enhanced sensitivity, specificity, stability, and reduced background interference for a wide range of applications.
3.4. MRI probe for K+ and Mg2+ monitoring
Potassium (K+) is widely distributed in the natural world in the form of salt and is also an important component in human muscle and nerve tissue. K+ is able to maintain nerve health and a normal heartbeat, can prevent strokes, and helps muscles contract normally. When high sodium intake leads to hypertension, K+ has the effect of lowering blood pressure.74 Suzuki's group75 (Fig. 6A and B) designed a Gd3+ contrast agent whose relaxivity relies on K+ concentration (KMR-K1, Gd-7). After adding K+, the r1 decreases from 5.05 to 4.78 mM−1 s−1. The Mg2+, an electrolyte found in significant amounts all over the body, is a crucial cofactor in more than 300 enzyme activities. In biology, interactions between Mg2+ and phosphate anions are common and important for the stability of DNA and RNA biomolecules.76 And Mg2+ has been linked to a number of cardiovascular diseases. A Gd-DTPA derivative modified with one charged b-diketone functionality (KMR-Mg, Gd-8) (Fig. 6A and B)75 responds to variations in Mg2+ concentrations. When 1 equivalent of Mg2+ is supplied, the KMR-r1 of Mg falls from 4.98 to 3.95 mM−1 s−1. Interestingly, Ling and colleague designed a K+-sensitive dual-mode nanoprobe for non-invasive identification of tumor malignancy. The probes presented high-performance T2 MRI capability. As illustrated in Fig. 6C, the invention of a K+-sensitive dual-mode nanoprobe improves the imaging performance from dual MRI and fluorescence imaging modalities, allowing for more accurate imaging of tumor malignancies based on ion homeostasis.77
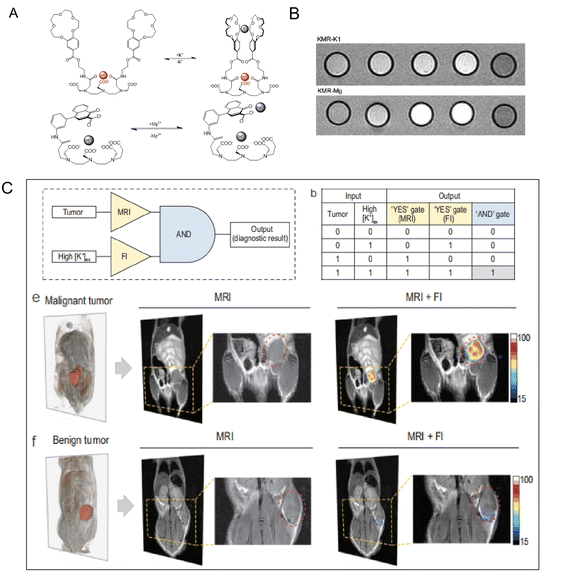 |
| Fig. 6 (A) Diagrammatic representation of the suggested conformational shift of KMR-K1 and KMR-Mg in the presence and absence of K+ or Mg2+, respectively. Reproduced from ref. 75 with permission from The Royal Society of Chemistry, copyright 2007. (B) T1-weighted MR images of KMR-K and KMR-Mg in the presence of various amounts of K+ and Mg2+. Reproduced from ref. 75 with permission from The Royal Society of Chemistry, copyright 2007. (C) Logic operation of tumor imaging in vivo after being injected with K+-sensitive dual-mode nanoprobe. Reproduced from ref. 77 with permission from Oxford university Press, copyright 2022. | |
3.5. MRI probe for Fe2+/3+ ion monitoring
The most prevalent transition metal in humans is iron. Iron can only exist in its stable redox states of ferrous ion (Fe2+) and ferric ion (Fe3+) under physiological conditions. The majority of the iron in cells is tightly attached to enzymes and certain transport and storage proteins, while the labile iron is only present in trace amounts and is only weakly bound to anions, which are multifunctional ligands. Based on its powerful redox capability, iron plays crucial roles in many biological processes, including DNA synthesis, electron transport, and oxygen metabolism. On the other hand, iron overload results in aberrant ROS generation that leads to severe cell damage and organ malfunction. A range of conditions, including cancer, hepatitis, and neurodegenerative illnesses, have also been connected to disruption in iron homeostasis. Age-related increases in iron levels in the brain are associated with Alzheimer's.78,79
In order to selectively detect Fe in aqueous solutions, Nagasawa and colleagues designed the F-Nox-1 19F-MRI probe as a Fe(ii)-selective chemical switch for the chemical shift and signal intensity of 19F signals in MR spectroscopy.80 There was no crosstalk between the 19F-MR signals of the N-oxide and its deoxygenated constituent. They attempted to detect Fe in cells using 19F-NMR for biological purposes. However, no discernible 19F signal was seen in the cells. F-Nox-1 cytotoxicity was not seen, indicating that the absence of the 19F signal was not attributable to cell death. As a result, the sensitivity of 19F-NMR spectroscopy, rather than cell-membrane permeability, would make the detection of cellular Fe significantly more challenging. The current work suggested that more efforts should be made to improve 19F signals for 19F-MR imaging of cellular labile Fe in cells and in vivo. F-Nox-1's extraordinarily low cytotoxicity and water solubility are both intriguing qualities for in vivo application. Next, double representative examples were designed.81,82 One Fen+ center links several Gd3+-chelates to build larger complexes with correspondingly longer rotational correlation periods and greater relaxivity values. These super-molecular assemblies were developed as high-relaxivity contrast agents. Even though these substances have not yet generally been described as iron-responsive MRI indications, they still serve as a suitable foundation for future sensor developments. For instance, 2,20-bipyridine (H8L) and phenanthroline (DTPA-5-amido-1,10-phenanthroline) have been developed for Fe2+ chelation. When 1/3 equiv. of Fe2+ is added to the bipyridine compound H8L, its effective mass relaxivity increases by 59% (r1 = 20.2 to 32.2 mM−1 s−1). Localized hyperintensity was seen in the right liver lobe on a T2-weighted MRI, showing that there were reperfused liver lobes after ischemia damage and focal dorsal muscle necrosis. The infarcted liver lobe was somewhat less intense or isointense on T1-weighted MRI when compared to the healthy liver, making the diagnosis difficult. The Fe homeostasis is essential for ferroptosis, as well as macrophage differentiation and polarization, which are linked to tumor development and malignant behavior.83 An activatable MR imaging nanoprobe for controlling and monitoring Fe3+ release was designed by Gao et al.84 The nanoprobes may release Fe3+ into the weak acidic environment of tumors, allowing the T1 MRI effect to be activated, providing a unique platform for detecting the release of exogenous Fe3+ for tumor treatment (Fig. 8D). MRI is an emerging technique for studying the spatial distribution of dominant iron sources in vivo. However, it is still challenging to distinguish between Fe(ii) and Fe(iii) using MRI probes regardless of the convoluted data acquisition and processing, giving prominence to develop molecular imaging probes enabling mapping of the Fe(ii) in living individuals. MRI probes for Fe2+/Fe3+ detection may indeed face challenges in terms of sensitivity, oxidation state discrimination, and interference from endogenous iron or other paramagnetic species. However, researchers can explore various strategies to overcome these limitations:
1. Enhancing sensitivity.
Amplification agents or signal enhancement methods can be incorporated into the probe design to improve the detection of low concentrations of Fe2+/Fe3+.
2. Selectivity improvement.
Modifying the probe structure or incorporating specific binding moieties can enhance the selectivity of the probe towards Fe2+/Fe3+.
3. Oxidation state discrimination.
Developing probes with different affinities for Fe2+ and Fe3+ or utilizing redox-sensitive probes can enable tracking changes in the oxidation state of iron.
4. Suppression of background interference.
Chelating agents or masking agents can be used to selectively bind to endogenous iron or interfering species, minimizing their impact on the detection of exogenous Fe2+/Fe3+.
By addressing these challenges and implementing appropriate solutions, the limitations of MRI probes for Fe2+/Fe3+ detection can be overcome, leading to improved sensitivity, selectivity, and oxidation state discrimination. This would have significant implications for applications involving tracking iron metabolism and studying iron-related processes in biological systems.
3.6. MRI probe for heavy metal ion monitoring
The Cd2+, as heavy metal ions, can harm human health as well as enter organs and tissues through the blood.85 For the requirement of high sensitivity detection, most Cd2+ responsive probes have been explored.86 Due to the buildup of Cd2+ in organisms, the ability to detect Cd2+ in living cells or tissues is essential. While the only frequently used way to detect it in vivo is optical imaging.87 Researchers have paid close attention to MRI probes, which can be used throughout the tissue since they are not constrained by light scattering or photobleaching. Recently, numerous MRI sensors have been created for biological purposes. However, few MRI sensors have been reported for metal ion detection in biological systems. To date, many magnetic nanoprobes for Pb2+ detection have been reported. As shown in Fig. 7A, the iron oxide nanoparticles modified with ethyl 1-(2-(3′,4′-dihydroxyphenyl)-2-oxoethyl)-1H-1,2,3-triazole-4 carboxylate (ETC) for Cd2+ detection were created by Yang's teams.88 The ETC-(Zn, Mn)Fe3O4 NPs were able to efficiently collect Cd2+ and demonstrate the sensitive and selective detection of Cd2+ in live cells. This technique demonstrates a straightforward, quick, and effective approach for detecting Cd2+, which has a wide range of potential applications in biological systems. In order to increase specificity, Yang's groups have further created 3-(3,4-dihydroxyphenyl) propionic acid (DHCA)-modified Fe/Fe3O4 nanoparticles (DHCA-Fe/Fe3O4) for the detection of Pb2+ with outstanding selectivity over other metal ions (Fig. 7B).89 As shown in Fig. 7C, Xu et al. designed novel MRI DNAzyme probes for the highly sensitive detection of Pb2+.90 With an increase in Pb2+ concentration, a decrease in the T2 relaxation time was present. This method employs functionalized magnetic nanoparticles to detect Pb2+ in an easy, quick, and effective manner that can also be broadly used to detect other species. One further hazardous metal that poses a major risk to human health and the environment is mercury.91,92 The three different types of mercury are organic compounds, inorganic salts, and elemental mercury. The most prevalent species in the environment are organic mercury compounds and inorganic mercury salts.93 As shown in Fig. 7D, the mercury ion caused the T–T coupling to assemble into the T-Hg-T structure after it was initially incorporated into the water solution containing the probe.94 Following the reaction, the scattered probes came together to form aggregates that altered the magnetic relaxation characteristics of nearby water protons and shortened the T2 relaxation time. Organic forms of mercury, primarily methylmercury species (CH3Hg+), are far more dangerous than their inorganic counterparts (Hg0, Hg2+) because of their high cellular membrane permeability and potential to accumulate in the brain, kidneys, and endocrine system. Singh et al. presented a Gd(III) complex MRI probe named o-MeHgGad95 for non-invasive visualization of CH3Hg+, which was motivated by the particular interaction of mercury with the sulphur ether. This “off–on” MRI probe was created using the dithioacetal groups’ removal as the response mechanism. Water molecules were not able to interact with the Gd(III) ion. At three equivalents of CH3Hg+, the relaxivity increased from 2.3 to 5.9 mM−1 s−1 due to the increased water molecule access to the Gd(III) ion. However, a minor relaxivity increase was also achieved in the presence of Cu2+. As shown in Fig. 8E, the T1-weighted MR images showed a considerable contrasting increase in the kidney, liver, and gut of mice exposed to CH3Hg+. Furthermore, the relaxivity increase was found to be highly selective to CH3Hg+ over other species.
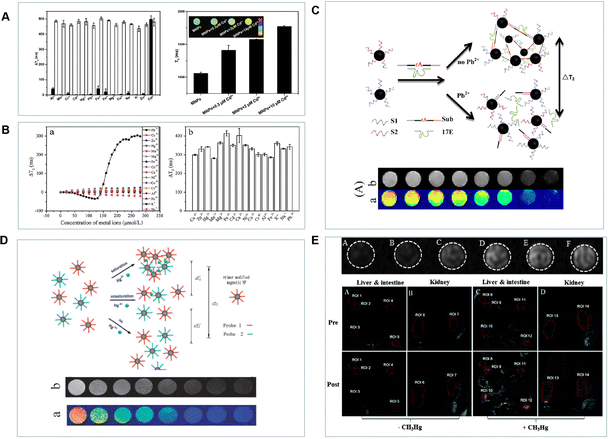 |
| Fig. 7 (A) The T2 values of ETC-(Zn, Mn)Fe3O4 NPs in the presence of different metal ions. Reproduced from ref. 88 with permission from Elsevier, copyright 2015. (B) The T2 intensity of DHCA-Fe/Fe3O4 NPs in the presence of Pb2+ and other metal ions. Reproduced from ref. 89 with permission from Elsevier, copyright 2016. (C) and (D) A diagram of the MRI probes for the detection of Pb2+ and Hg2+. Reproduced from ref. 90 and 94 with permission from American Chemical Society and The Royal Society of Chemistry, copyright 2013 and 2011. (E) The MR images of CH3Hg+-mediated probes and T1-weighted MR images of C57BL/6JNarl mice after injection of o-MeHgGad. Reproduced from ref. 95 with permission from The Royal Society of Chemistry, copyright 2015. | |
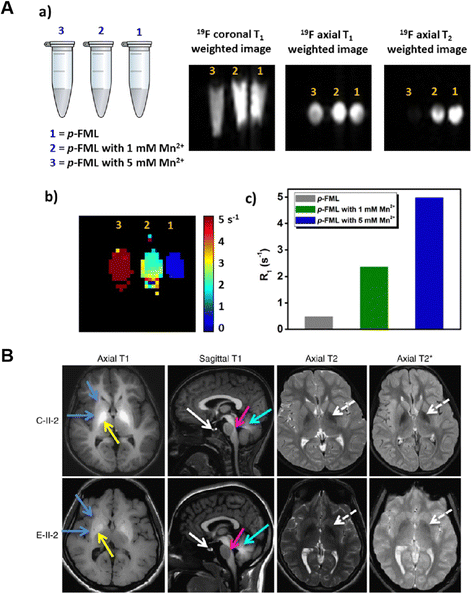 |
| Fig. 8 (A) MRI signal of the Mn2+ sensitive probe after treatment with 0 mM, 1 mM and 5 mM Mn2+. Reproduced from ref. 98 with permission from Elsevier, copyright 2017. (B) MRI brain images of patients overexposed to environmental Mn. Reproduced from ref. 99 with permission from Springer Nature, copyright 2016. | |
MRI probes for heavy metal ion detection should be designed with a focus on minimizing potential toxicity and improving selectivity and sensitivity. Researchers can consider the following strategies:
1. Designing selective probes.
Incorporate specific binding moieties or functional groups that selectively interact with the target heavy metal ions, reducing cross-reactivity with other substances in the sample matrix.
2. Signal amplification.
Use amplification agents or signal enhancement methods to improve the probe's ability to detect low concentrations of heavy metal ions, enhancing sensitivity.
3. Minimizing toxicity.
Pay careful attention to the potential toxicity of the probes by exploring the use of biocompatible materials or encapsulation strategies to minimize the release of heavy metal ions and reduce potential toxicity concerns.
By addressing these challenges and implementing potential solutions, the limitations of MRI probes for heavy metal ion detection can be overcome, leading to improved selectivity, sensitivity, interference mitigation, and safety for various applications involving heavy metal ion detection.
3.7. MRI probe for Mn2+ ion monitoring
Manganese (Mn) is crucial for controlling a wide range of biological processes. Several enzymes use Mn2+ as a structural and catalytic cofactor.96,97 According to estimates of Mn concentrations in pathological settings, the average concentration is about 160 μM in the brain, while striatal local concentrations can reach 1–5 mM. In this situation, high sensitivity detection methods are crucial for detecting Mn2+. For patho-physiologically significant Mn2+ concentrations, Datta et al.98 designed a fluorinated macrocyclic ligand (Fig. 8A) that allows for a linear rise in 19F relaxation rates. Importantly, even at mM levels of Mn2+, the impacts of line widening could be seen in the 19F NMR signal intensities. For Mn2+ detection in the biological milieu, water solubility is an important factor. To obtain a water-soluble Mn2+ probe, they added arms in the macrocycle that contained an anionic oxygen donor. The 19F-MR probe may also be useful for visualizing Mn2+ distributions in biological material, according to T1 and T2 weighted 19F-MR images of phantoms. Following the anterior pituitary gland, the midbrain, pontine tegmentum, and corpus callosum, high Mn accumulation was found in the globus pallidus region of the basal ganglia, putamen, caudate, subthalamic, and dentate nuclei in the MRI images of the brains of manganism patients (Fig. 8B).99 Moreover, because 1H-MRI is based on NMR, it has poor sensitivity by nature and is only now helpful for highlighting areas with high mM to M levels of Mn buildup.
MRI probes for Mn2+ detection may face challenges due to background interference from endogenous Mn2+ or other paramagnetic species, as well as potential toxicity concerns associated with high Mn2+ concentrations. To overcome these limitations, researchers can consider the following solutions:
1. Minimizing interference.
Carefully design the probe structure or incorporate specific binding moieties that enhance selectivity for Mn2+, reducing interference from other paramagnetic species or endogenous Mn2+ in biological samples.
2. Enhancing sensitivity.
Implement signal amplification techniques or signal enhancement methods to improve the probe's ability to detect low concentrations of Mn2+, increasing sensitivity in Mn2+ detection.
3. Addressing potential toxicity.
Pay attention to the potential toxicity of the probe, especially in applications involving in vivo imaging. Modify the probe's design or explore the use of biocompatible materials or encapsulation strategies to minimize any release of Mn2+ and reduce potential toxicity concerns.
Conclusions
Due to its capacity to provide images with great spatial and temporal resolution from deep inside the body, MRI technology has been widely used in disease diagnosis and scientific research. There has been a lot of interest in developing responsive MRI probes for metal ion detection. The MRI probes respond to endogenous and external stimuli via a particular interaction, binding, or reaction with a targeted analyte and then produce distinctive changes in the MRI signal for the detection of metal ions. Various strategies have been suggested for the development of metal ion responsive probes, as outlined in Table 1. The most popular method for creating MRI probes is based on adjusting the amount of coordinated water molecules to modify relaxivity. Although these methods have received extensive research attention in the development of MRI probes, there is still a great need for the identification of novel response mechanisms. Future designs of more durable MRI probes for in vivo imaging will be possible thanks to the new processes. The MRI technology for metal ion detection is still limited by its sensitivity/selectivity, accurate quantification\imaging and biological safety. To speed-up the widespread adoption of MRI technology for metal ion detection in vivo and in vitro, we propose the following considerations:
Table 1 Performance of various probes in metal ion detection via MRI imaging
Metal ions |
Probe name |
Chelating agent |
Imaging modality |
Ref. |
Zn2+ |
GdDOTA-diBPEN |
N,N-bis-(2-pyridyl-methyl) ethylene diamine |
T
1
|
34, 35
|
Gd-daa3 |
Iminodiacetate or pyridine |
T
1
|
36, 37
|
GdL |
Sulfonamidoquinoline |
T
1
|
38, 39
|
Mn-(DPA-C2)2-TPPS3 |
Dipicolylamine |
T
1
|
40
|
Ca2+ |
Gd-DOPTRA |
APTRA (o-amino-phenol-N,N,O-triacetate) |
T
1
|
49
|
GdL1-GdL4 |
Polyaminocarboxylates and polyetheraminocarboxylates |
T
1
|
50
|
GdL1,GdL2 |
EGTA |
T
1,1H,19F |
51, 52
|
L1,L2,L3 |
Ethylenediaminetetraacetic acid (EDTA) and diethylenetriamine pentaacetic acid (DTPA) |
T
1
|
53
|
Gd2L1,Gd2L2 |
EGTA |
T
1
|
54
|
DOPTA-Gd (Gd-1) |
BAPTA |
T
1
|
56
|
M2+-5F-BAPTA |
BAPTA |
T
1
|
57
|
PEI/PEG- SPIONs |
EGTA |
T
2
|
58
|
ManICS1-AM |
BAPTA |
T
1
|
59
|
MaCaReNas |
C2 domains of synaptotagmin 1 |
T
2
|
60
|
Cu2+ |
copper-gad-1 |
Iminodiacetate |
T
1
|
69
|
CG2-CG6 |
Thioether-based copper receptors |
T
1
|
70
|
Copper-Gad-7 |
2-(Ethylthio)-N-(2-(ethylthio)ethyl)ethana |
T
1
|
70
|
Nido-carborane derivatives 4 |
N,N,N′,N′-Tetramethylethylenediamine |
11B |
71
|
Cu2+-PVIm |
poly-N-Vinylimidazole (PVIm) |
T
1
|
72
|
GdL1 |
Iminodiacetate |
T
1
|
73
|
K+,Mg2+ |
KMR-K1, Gd-7 |
Crown ether |
T
1
|
76
|
KMR-Mg Gd-8 |
b-diketone functionality |
T
1
|
76
|
Fe2+/3+ |
F-Nox-1 |
Tetrafluoro-p-phenylenediamine (TFPDA) |
19F |
80
|
H8L |
TTAHA (N-tris(2-aminoethyl)amine-N′,N′,N′′,N′′,N′′′,N′′′-hexaacetate) |
T
2
|
81, 82
|
UCPN-GA |
T
1
|
84
|
Heavy metal ions |
ETC-(Zn,Mn)Fe3O4 |
Ethyl 1-(2-(3′,4′-dihydroxyphenyl)-2-oxoethyl)-1H-1,2,3-triazole-4-carboxylate |
T
2
|
88
|
DHCA-Fe/Fe3O4 |
3-(3,4-Dihydroxyphenyl)propionic acid(DHCA) |
T
2
|
88
|
Fe3O4 MNPs |
DNAzyme |
T
2
|
90
|
Gold nanoparticle |
DNA |
T
2
|
94
|
o-MeHgGad |
Disulfide bond |
T
1
|
95
|
Mn2+ |
p-FML |
Penta-aza macrocyclic ligand |
19F |
98
|
Enhancing detection specificity
Traditional metal ion MRI detection probes can be interfered with by various metal ions, the biological microenvironment, enzymes, and other substances, leading to reduced detection specificity. To improve specificity, it is crucial to design novel probes or identify specific metal ion response targets that offer higher selectivity and minimize interference.
Ensuring probe safety
MRI probes for metal ion detection play a vital role in biomedical imaging and diagnostics. It is essential for these probes to have minimal toxicity to ensure safe in vivo use. Extensive toxicity testing is typically performed to assess the safety profile of MRI probes. Additionally, the probes should be efficiently cleared from the body after imaging to minimize any potential long-term effects. Stability during imaging is crucial, as the probe should not degrade or release toxic byproducts.
Understanding metabolic pathways and excretion mechanisms
Upon administration, MRI probes are taken up by cells through mechanisms like passive diffusion or active transport. They may undergo metabolic transformations or enzymatic reactions that modify their structure. The specific metabolic pathways can vary depending on the probe and the targeted metal ion. After imaging, the probe or its metabolites are eliminated from the body through routes such as renal excretion or metabolism by liver enzymes. It is important to conduct extensive research and preclinical studies to understand the bio-safety and metabolic pathways of MRI probes for metal ion detection.
By addressing these considerations, researchers can overcome sensitivity/selectivity limitations, ensure accurate quantification/imaging, and enhance the biological safety of MRI technology for metal ion detection. These efforts will contribute to the wider adoption and application of MRI in the field of metal ion detection in both in vivo and in vitro settings.
Conflicts of interest
The authors have declared no conflicts of interest for this article.
Acknowledgements
This review was supported by the National Natural Science Foundation of China (grants U21A20287, 51872088, 21890744, and 21974039), the National Key R&D Program of China (2019YFA0210100), the Science and Technology Project of Hunan Province (2020RC3022 and 2020SK2014), the National Natural Science Foundation of China (22374045), Youth medical key talents foundation of Hunan Province (20230508-1034) and China Postdoctoral Science Foundation (2023M731063).
Notes and references
- H. W. Mbatia and S. C. Burdette, Photochemical tools for studying metal ion signaling and momeostasis, Biochemistry, 2012, 51, 7212–7224 CrossRef CAS PubMed.
- K. P. Carter, A. M. Young and A. E. Palmer, Fluorescent sensors for measuring metal ions in living systems, Chem. Rev., 2014, 114, 4564–4601 CrossRef CAS PubMed.
- H. J. Feng, Q. R. Fu, W. Du, Q. Q. Li, L. C. Su and J. B. Song, Quantitative assessment of copper(II) in wilson's disease based on photoacoustic imaging and ratiometric surface-enhanced raman scattering, ACS Nano, 2021, 15, 3402–3414 CrossRef CAS PubMed.
- E. Mocchegiani, R. Giacconi and M. Malavolta, Zinc signalling and subcellular distribution: emerging targets in type 2 diabetes, Trends Mol. Med., 2008, 14, 419–428 CrossRef CAS PubMed.
- L. Fouani, S. V. Menezes, M. Paulson, D. R. Richardson and Z. Kovacevic, Metals and metastasis: exploiting the role of metals in cancer metastasis to develop novel anti-metastatic agents, Pharmacol. Res., 2017, 115, 275–287 CrossRef CAS.
- L. Zhang, Q. Cheng, C. X. Li, X. Zeng and X. Z. Zhang, Near infrared light-triggered metal ion and photodynamic therapy based on AgNPs/porphyrinic MOFs for tumors and pathogens elimination, Biomaterials, 2020, 248, 120029 CrossRef CAS PubMed.
- L. L. Zeng, G. C. Ma, J. Lin and P. Huan, Photoacoustic probes for molecular detection: recent advances and perspectives, Small, 2018, 14, 1800782 CrossRef.
- Q. Wang, K. N. Wei, S. Z. Huang, Q. Tang, Z. Tao and Y. Huang, “Turn-Off” supramolecular fluorescence array sensor for heavy metal ion identifification, ACS Omega, 2021, 6, 31229–31235 CrossRef CAS.
- Z. Y. Guo, H. Y. Yu, W. Ren and Q. S. Wang, Selective aqueous fluorescent probes for metal ions based on benzoyl hydrazone derivatives, Anal. Methods, 2015, 7, 8129–8137 RSC.
- D. Wu, L. Y. Chen, W. L. Lee, G. K. Ko, J. Yin and J. Y. Yoon, Recent progress in the development of organic dye based near-infrared fluorescence probes for metal ions, Coord. Chem. Rev., 2018, 354, 74–97 CrossRef CAS.
- C. Xu, M. He, B. B. Chen and B. Hu, Magnetic porous coordination networks for preconcentration of various metal ions from environmental water followed by inductively coupled plasma mass spectrometry detection, Talanta, 2022, 245, 123470 CrossRef CAS.
- Z. Dong, P. Liang, G. Q. Guan, B. L. Yin, Y. J. Wang and G. S. Song, Overcoming hypoxia-induced ferroptosis resistance via a 19F/1H-MRI traceable core-shell nanostructure, Angew. Chem., Int. Ed., 2022, 61, e202206074 CrossRef CAS.
- R. Y. Yue, C. Zhang, X. Li, Y. J. Wang, G. Q. Guan, X. B. Zhang and G. S. Song, Dual key co-activated nanoplatform for switchable MRI monitoring accurate ferroptosis-based synergistic therapy, Chem, 2022, 8, 1956–1981 CAS.
- C. Lu, L. B. Han, J. Wang, J. C. Wan, G. S. Song and J. H. Rao, Engineering of magnetic nanoparticles as magnetic particle imaging tracers, Chem. Soc. Rev., 2021, 50, 8102–8146 RSC.
- G. S. Song, X. C. Zheng, Y. J. Wang, X. Xia, S. Chu and J. H. Rao, A magneto-optical nanoplatform for multimodality imaging of tumors in mice, ACS Nano, 2019, 13, 7750–7758 CrossRef CAS PubMed.
- Y. D. Yang, T. X. Yang, F. F. Chen, C. Zhang, B. L. Yin, X. Yin, L. B. Han, Q. J. Xie, X. B. Zhang and G. S. Song, Degradable magnetic nanoplatform with hydroxide Ions triggered photoacoustic, MR imaging, and photothermal conversion for precise cancer theranostic, Nano Lett., 2022, 22, 3228–3235 CrossRef CAS PubMed.
- G. S. Song, M. Chen, Y. R. Zhang, L. Y. Cui, H. B. Qu, X. C. Zheng, M. Wintermark, Z. Liu and J. H. Rao, Janus iron oxides @ semiconducting polymer nanoparticle tracer for cell tracking by magnetic particle imaging, Nano Lett., 2018, 18, 182–189 CrossRef CAS PubMed.
- C. Zhang, J. Q. Li, C. Lu, T. X. Yang, Y. Zhao, L. L. Teng, Y. Yang, G. S. Song and X. B. Zhang, H2S-activated “one-Key triple-lock” bis-metal coordination network for visualizing precise therapy of colon cancer, CCS Chem., 2021, 3, 2126–2142 CrossRef CAS.
- C. Yang, G. S. Song, H. F. Yuan, Y. Yang, Y. Q. Wang, D. J. Ye, H. M. Meng, S. Y. Huan and X. B. Zhang, Manganese-fluorouracil metallodrug nanotheranostic for MRI-correlated drug release and enhanced chemoradiotherapy, CCS Chem., 2020, 2, 1116–1128 Search PubMed.
- K. Hanaoka, K. Kikuchi, Y. Urano and T. Nagano, Selective sensing of zinc ions with a novel magnetic resonance imaging contrast agent, J. Chem. Soc., Perkin Trans., 2001, 2, 1840–1843 RSC.
- J. L. Major, R. M. Boiteau and T. J. Meade, Mechanisms of ZnII-activated magnetic resonance imaging agents, Inorg. Chem., 2008, 47, 10788–10795 CrossRef CAS PubMed.
- R. G. Pautler, A. C. Silva and A. P. Koretsky, In vivo neuronal tract tracing using manganese-enhanced magnetic resonance imaging, Magn. Reson. Med., 1998, 40, 740–748 CrossRef CAS PubMed.
- H. Hifumi, A. Tanimoto, D. Citterio, H. Komatsua and K. Suzuki, Novel 15-crown-5 ether or β-diketone incorporated gadolinium complexes for the detection of potassium ions or magnesium and calcium ions, Analyst, 2007, 132, 1153–1160 RSC.
- D. Kasala, T. S. Lin, C. Y. Chen, G. C. Liu, C. L. Kao, T. L. Cheng and Y. M. Wang, [Gd(Try-TTDA)(H2O)]2−: A new MRI contrast agent for copper ion sensing, Dalton Trans., 2011, 40, 5018–5025 RSC.
- W. S. Li, J. Luo and Z. N. Chen, A gadolinium(III) complex with 8-amidequinoline based ligand as copper(II) ion responsive contrast agent, Dalton Trans., 2011, 40, 484–488 RSC.
- E. L. Que and C. J. Chang, A smart magnetic resonance contrast agent for selective copper sensing, J. Am. Chem. Soc., 2006, 128, 15942–15943 CrossRef CAS.
-
Y. M. Xiao, G. Y. Zhao, X. X. Fang, Y. X. Zhao, G. H. Wang, W. Yang and J. W. Xu, A smart copper(II)-responsive binuclear gadolinium(III) complex-based magnetic resonance imaging contrast agent, RSC. Adv., 2014, 4, 34421–34427 Search PubMed.
- N. Tassali, N. Kotera, C. Boutin, E. Dubost, F. Taran and P. Berthault, Smart detection of toxic metal ions, Pb2+ and Cd2+, using a 129Xe NMR-based sensor, Anal. Chem., 2014, 86, 1783–1788 CrossRef CAS.
- L. J. Li, J. H. Wang, S. H. Xu, C. X. Li and B. Dong, Recent progress in fluorescent probes for metal ion detection, Front. Chem., 2022, 10, 875241 CrossRef CAS.
- H. Yin, A. Truskewycz and I. S. Cole, Quantum dot (QD)-based probes for multiplexed determination of heavy metal ions, Microchim. Acta, 2020, 187, 336 CrossRef CAS PubMed.
- L. L. Zeng, G. C. Ma, J. Lin and P. Huan, Photoacoustic probes for molecular detection: recent advances and perspectives, Small, 2018, 14, 1800782 CrossRef.
- H. Allouche-Arnon, N. D. Tirukoti and A. Bar-Shir, MRI-based sensors for in vivo imaging of metal ions in biology, Isr. J. Chem., 2017, 57, 1–12 CrossRef.
- B. L. Vallee and D. S. Auld, Zinc coordination, function, and structure of zinc enzymes and other proteins, Biochemistry, 1990, 29, 5647–5659 CrossRef CAS PubMed.
- A. C. Esqueda, J. A. Lopez, J. Ratnakar, A. J. M. Lubag, A. D. Sherry and L. M. D. Rodrıguez, A new gadolinium-based MRI zinc sensor, J. Am. Chem. Soc., 2009, 131, 11387–11391 CrossRef CAS PubMed.
- J. Yu, A. F. Martins, C. Preihs, V. C. Jordan, S. Chirayil, P. Y. Zhao, Y. K. Wu, K. Nasr, G. E. Kiefer and A. D. Sherry, Amplifying the sensitivity of Zinc(II) responsive MRI contrast agents by altering water exchange rates, J. Am. Chem. Soc., 2015, 137, 14173–14179 CrossRef CAS PubMed.
- J. L. Major, R. M. Boiteau and T. J. Meade, Mechanisms of ZnII-activated magnetic resonance imaging agents, Inorg. Chem., 2008, 47, 10788–10795 CrossRef CAS PubMed.
- J. L. Major, G. Parigi, C. Luchinat and T. J. Meade, The synthesis and in vitrotesting of a zinc-activated MRI contrast agent, Proc. Natl. Acad. Sci. U. S. A., 2007, 104, 13881 CrossRef CAS.
- J. Luo, W. S. Li, P. Xu, L. Y. Zhang and Z. N. Chen, Zn2+ responsive bimodal magnetic resonance imaging and fluorescent imaging probe based on a gadolinium(III) complex, Inorg. Chem., 2012, 51, 9508–9516 CrossRef CAS.
- M. Regueiro-Figueroa, S. Gündüz, V. Patinec, N. K. Logothetis, D. Esteban-Gómez and C. Platas-Iglesias, Gd3+-based magnetic resonance imaging contrast agent responsive to Zn2+, Inorg. Chem., 2015, 54, 10342–10350 CrossRef CAS PubMed.
- X. A. Zhang, K. S. Lovejoy, A. Jasanoff and S. J. Lippard, Water-soluble porphyrins as a dual-function molecular imaging platform for MRI and fluorescencezinc sensing, Proc. Natl. Acad. Sci. U. S. A., 2007, 104, 10780–10785 CrossRef CAS.
- A. J. M. Lubaga, L. M. D. Rodriguez, S. C. Burgessa and A. D. Sherry, Noninvasive MRI of β-cell function using a Zn2+-responsive contrast agent, Proc. Natl. Acad. Sci. U. S. A., 2011, 108, 18400–18405 CrossRef PubMed.
- G. J. Wang and G. Angelovski, Highly potent MRI contrast agent displaying outstanding sensitivity to zinc ions, Angew. Chem., Int. Ed., 2021, 60, 5734–5738 CrossRef CAS.
- N. D. Tirukoti, L. Avram, T. Haris, B. Lerner, Y. Diskin-Posner, H. Allouche-Arnon and A. Bar-Shir, Fast ion-chelate dissociation rate for in vivo MRI of labile zinc with frequency-specific encodability, J. Am. Chem. Soc., 2021, 143, 11751–11758 CrossRef CAS PubMed.
- Y. Yuan, Z. L. Wei, C. Y. Chu, J. Zhang, X. L. Song, P. Walczak and J. W. M. Bulte, Developing Zinc-specific iCEST MRI as imaging biomarker for prostate cancer, Angew. Chem., Int. Ed., 2019, 58, 15512–15517 CrossRef CAS PubMed.
- M. J. Berridge, P. Lipp and M. D. Bootman, The versatility and universality of calcium signalling, Nat. Rev. Mol. Cell Biol., 2000, 1, 11–21 CrossRef CAS PubMed.
- D. W. Domaille, E. L. Que and C. J. Chang, Synthetic fluorescent sensors for studying the cell biology of metals, Nat. Chem. Biol., 2008, 4, 168–175 CrossRef CAS PubMed.
- A. Miyawaki, J. Llopis, R. Heim, J. M. McCaffery, J. A. Adams, M. Ikura and R. Y. Tsien, Fluorescent indicators for calcium based on green fluorescent proteins (GFPs) and calmodulin, Microsc. Microanal., 1997, 4, 446–447 CrossRef.
- S. Ogawa, T. M. Lee, A. R. Kay and D. W. Tank, Brain magnetic resonance imaging with contrast dependent on blood oxygenation, Proc. Natl. Acad. Sci. U. S. A., 1990, 87, 9868–9872 CrossRef CAS.
- K. Dhingra, M. E. Maier, M. Beyerlein, G. Angelovski and N. K. Logothetis, Synthesis and characterization of a smart contrast agent sensitive to calcium, Chem. Commun., 2008, 3444–3446 RSC.
- I. Mamedov, N. K. Logothetisa and G. Angelovski, Structure-related variable responses of calcium sensitive MRI probes, Org. Biomol. Chem., 2011, 9, 5816–5824 RSC.
- K. Connah, V. Truffault, C. Platas-Iglesias and G. Angelovski, Investigations into the effects of linker length elongation on the behaviour of calcium-responsive MRI probes, Dalton Trans., 2019, 48, 13546–13554 RSC.
- P. Kadjane, C. Platas-Iglesias, P. Boehm-Sturm, V. Truffault, G. E. Hagberg and G. Angelovski, Dual-frequency calcium-responsive MRI agents, Chem. – Eur. J., 2014, 20, 7351–7362 CrossRef CAS.
- A. Mishra, P. Fousková, G. Angelovski, E. Balogh, A. K. Mishra and É. Tóth, Facile synthesis and relaxation properties of novel bispolyazamacrocyclic Gd3+ complexes: an attempt towards calcium-sensitive MRI contrast agents, Inorg. Chem., 2008, 47, 1370–1381 CrossRef CAS.
- G. Angelovski, P. Fouskova, I. Mamedov, S. Canals, E. Toth and N. K. Logothetis, Smart magnetic resonance imaging agents that sense extracellular calcium fluctuations, Chem. Bio. Chem., 2008, 9, 1729–1734 CrossRef CAS PubMed.
- K. Connah, V. Truffault, C. Platas-Iglesias and G. Angelovski, Investigations into the effects of linker length elongation on the behaviour of calcium-responsive MRI probes, Dalton Trans., 2019, 48, 13546–13554 RSC.
- W. H. Li, G. Parigi, M. Fragai, C. Luchinat and T. J. Meade, Mechanistic studies of a calcium-dependent MRI contrast agent, Inorg. Chem., 2002, 41, 4018 CrossRef CAS.
- A. Bar-Shir, A. Assaf Gilad, K. W. Y. Chan, G. S. Liu, P. C. M. V. Zijl and M. T. McMahon, Metal ion sensing using ion chemical exchange saturation transfer 19F magnetic resonance imaging, J. Am. Chem. Soc., 2013, 135, 12164–12167 CrossRef CAS PubMed.
- P. F. Xua, Z. W. Shen, B. L. Zhang, J. Wang and R. H. Wu, Synthesis and characterization of superparamagnetic iron oxide nanoparticles as calcium-responsive MRI contrast agents, Appl. Surf. Sci., 2016, 389, 560–566 CrossRef.
- A. Barandov, B. B. Bartelle, C. G. Williamson, E. S. Loucks, S. J. Lippard and A. Jasanoff, Sensing intracellular calcium ions using a manganese-based MRI contrast agent, Nat. Commun., 2019, 10, 897 CrossRef.
- S. Okada, B. B. Bartelle, N. Li, V. Breton-Provencher, J. J. Lee, E. Rodriguez, J. Melican, M. Sur and A. Jasanoffff, Calcium-dependent molecular fMRI using a magnetic nanosensor, Nat. Nanotechnol., 2018, 13, 473–477 CrossRef CAS PubMed.
- Y. B. Shi, Q. Y. Liu, W. Yuan, M. Xue, W. Feng and F. Y. Li, Dye-assembled upconversion nanocomposite for luminescence ratiometric in vivo bioimaging of copper ions, ACS Appl. Mater. Interfaces, 2019, 11, 430–436 CrossRef CAS.
- C. Wang, X. Y. Bi, M. C. Wang, X. Zhao and Y. Q. Lin, Dual-Channel online optical detection platform integrated with a visible light absorption approach for continuous and simultaneous in vivo monitoring of ascorbic acid and copper(II) ions in a living rat brain, Anal. Chem., 2019, 91, 16010–16016 CrossRef CAS.
- M. J. Berridge, P. Lipp and M. D. Bootman, The versatility and universality of calcium signalling, Nat. Rev. Mol. Cell Biol., 2000, 1, 11–21 CrossRef CAS.
- Q. Gao, L. Ji, Q. N. Wang, K. Yin, J. H. Li and L. X. Chen, Colorimetric sensor for highly sensitive and selective detection of copper ion, Anal. Methods, 2017, 9, 5094 RSC.
- A. Annu and B. Mohita, Wilson disease, Curr. Opin. Neurol., 2020, 33, 534–542 CrossRef.
- W. Qu, Y. Liu, D. Liu, Z. Wang and X. Jiang, Copper-mediated amplification allows readout of immunoassays by the naked eye, Angew. Chem., Int. Ed., 2011, 123, 3504–3507 CrossRef.
- A. C. Esqueda, J. A. Lopez, J. Ratnakar, A. J. M. Lubag, A. D. Sherry and L. M. D. Rodriguez, A new gadolinium-based MRI zinc sensor, J. Am. Chem. Soc., 2009, 131, 11387–11391 CrossRef CAS PubMed.
- L. N. Goswami, L. Ma, S. Chakravarty, Q. Cai, S. S. Jalisatgi and M. F. Hawthorne, Discrete nanomolecular polyhedral borane scaffold supporting multiple gadolinium(III) complexes as a high performance MRI contrast agent, Inorg. Chem., 2013, 52, 1694–1700 CrossRef CAS PubMed.
- E. L. Que and C. J. Chang, A smart magnetic resonance contrast agent for selective copper sensing, J. Am. Chem. Soc., 2006, 128, 15942–15943 CrossRef CAS.
- E. L. Que, E. Gianolio, S. L. Baker, A. P. Wong, S. Aime and C. J. Chang, Copper-responsive magnetic resonance imaging contrast agents, J. Am. Chem. Soc., 2009, 131, 8527–8536 CrossRef CAS.
- T. Tanaka, R. Araki, T. Saido, R. Abe and S. Aoki, 11B NMR/MRI sensing of copper(II) ions in vitro by the decomposition of a hybrid compound of anido-o-carboraneand a metal chelator, Eur. J. Inorg. Chem., 2016, 3330–3337 CrossRef CAS.
- M. Y. Hu, J. Chen, J. M. Wang, Y. Zhang, L. Liu, P. C. Moraisa and H. Bi, Cu2+-complex of hydrophilic nitrogen-rich polymer dots applied as a new MRI contrast agent, Biomater. Sci., 2017, 5, 2319–2327 RSC.
- N. N. Paranawithana, A. F. Martins, V. C. Jordan, P. Y. Zhao, S. Chirayil, G. Meloni and A. D. Sherry, A responsive magnetic resonance imaging contrast agent for detection of excess copper(II) in the liver in vivo, J. Am. Chem. Soc., 2019, 141, 11009–11018 CrossRef CAS PubMed.
- J. H. Youn and A. A. McDonough, Recent advances in understanding integrative control of potassium homeostasis, Annu. Rev. Physiol., 2009, 71, 381 CrossRef CAS.
- H. Hifumi, A. Tanimoto, D. Citterio, H. Komatsua and K. Suzuki, Novel 15-crown-5 ether or β-diketone incorporated gadolinium complexes for the detection of potassium ions or magnesium and calcium ions, Analyst, 2007, 132, 1153–1160 RSC.
- J. G. Gums, Magnesium in cardiovascular and other disorders, Am. J. Health Syst. Pharm., 2004, 61, 1569 CrossRef CAS PubMed.
- Q. Wang, F. Li, Z. Liang, H. Liao, B. Zhang, P. Lin, X. Liu, S. Hu, J. Lee and D. Ling, A K+-sensitive AND-gate dual-mode probe for simultaneous tumor imaging and malignancy identifification, Natl. Sci. Rev., 2022, 9, nwac080 CrossRef CAS PubMed.
- L. Zecca, M. B. H. Youdim, P. Riederer, J. R. Connor and R. R. Crichton, Iron, brain ageing and neurodegenerative disorders, Nat. Rev. Neurosci., 2004, 5, 863 CrossRef CAS.
- N. Solovyev, A. H. El-Khatib, M. Costas-Rodríguez, K. Schwab, E. Griffin, A. Raab, B. Platt, F. Theuring, J. Vogl and F. Vanhaecke, Cu, Fe, and Zn isotope ratios in murine Alzheimer's disease models suggest specific signatures of amyloidogenesis and tauopathy, J. Biol. Chem., 2021, 296, 100292 CrossRef CAS PubMed.
- R. Kakiuchi, T. Hirayama, D. Yanagisawa, I. Tooyama and H. Nagasawa, A 19F-MRI probe for the detection of Fe(II) ions in an aqueous system, Org. Biomol. Chem., 2020, 18, 5843–5849 RSC.
- J. B. Livramento, É. Tóth, A. Sour, A. Borel, A. E. Merbach and R. Ruloff, High relaxivity confined to a small molecular space: a metallostar-based, potential MRI contrast agent, Angew. Chem., 2005, 44, 1480 CrossRef CAS.
- T. N. Parac-Vogt, L. V. Elst, K. Kimpe, S. Laurent, C. Burtéa and K. Binnemans, Pharmacokinetic and in vivo evaluation of a self-assembled gadolinium(III)-iron(II) contrast agent with high
relaxivity, Contrast Media Mol. Imaging, 2006, 1, 267 CrossRef CAS PubMed.
- B. R. Stockwell, J. P. Friedmann Angeli, H. Bayir, A. I. Bush, M. Conrad, S. J. Dixon, S. Fulda, S. Gascon, S. K. Hatzios, V. E. Kagan, K. Noel, X. Jiang, A. Linkermann, M. E. Murphy, M. Overholtzer, A. Oyagi, G. C. Pagnussat, J. Park, Q. Ran, C. S. Rosenfeld, K. Salnikow, D. Tang, F. M. Torti, S. V. Torti, S. Toyokuni, K. A. Woerpel and D. D. Zhang, Ferroptosis: a regulated cell death nexus linking metabolism, redox biology, and disease, Cell, 2017, 171, 273–285 CrossRef CAS PubMed.
- P. Zhang, Y. Hou, J. Zeng, Y. Li, Z. Wang, R. Zhu, T. Ma and M. Gao, Coordinatively unsaturated Fe3+ based activatable probes for enhanced MRI and therapy of tumors, Angew. Chem., Int. Ed., 2019, 58, 11088–11096 CrossRef CAS.
- L. Jarup and A. Akesson, Current status of cadmium as an environmental health problem, Toxicol. Appl. Pharmacol., 2009, 238, 201–208 CrossRef PubMed.
-
H. Li, Y. Yao, C. Han and J. Zhan, Triazole-ester modified silver nanoparticles: click synthesis and Cd2+ colorimetric sensing, Chem. Commun., 2009, 4812–4814 Search PubMed.
- T. Cheng, Y. Xu, S. Zhang, W. Zhu, X. Qian and L. Duan, A highly sensitive and selective OFF-ON fluorescent sensor for cadmium in aqueous solution and living cell, J. Am. Chem. Soc., 2008, 130, 16160–16161 CrossRef CAS.
- Y. Zhang, J. C. Shen, H. Yang, Y. Yang, Z. G. Zhou and S. P. Yang, A highly selective magnetic sensor for Cd2+ in living cells with (Zn, Mn)-doped iron oxide nanoparticles, Sens. Actuators, B, 2015, 207, 887–892 CrossRef CAS.
- Y. Yang, Y. Zhang, J. C. Shen, H. Yang, Z. G. Zhou and S. P. Yang, A highly selective magnetic sensor with functionalized Fe/Fe3O4 nanoparticles for detection of Pb2+, Chin. Chem. Lett., 2016, 27, 891–895 CrossRef CAS.
- L. G. Xu, H. H. Yin, W. Ma, L. B. Wang, H. Kuang and C. L. Xu, MRI biosensor for lead detection based on the DNAzyme-induced catalytic reaction, J. Phys. Chem. B, 2013, 117, 14367–14371 CrossRef CAS.
- O. P. Ajsuvakova, A. A. Tinkov, M. Aschner, J. B. T. Rocha, B. Michalke and G. Bjørklund, Sulfhydryl groups as targets of mercury toxicity, Coord. Chem. Rev., 2020, 417, 213343 CrossRef CAS.
- J. Li, S. T. Wang, X. H. Cao, H. N. Huang and D. P. Cao, Sulfur-modified porous covalent organic polymers as bifunctional materials for efficient fluorescence detection and fast removal of heavy metal ions, Mater. Chem. Front., 2021, 5, 3428 RSC.
- T. W. Clarkson and L. Magos, The toxicology of mercury and its chemical compounds, Crit. Rev. Toxicol., 2006, 36, 609–662 CrossRef CAS PubMed.
- W. W. Ma, C. L. Hao, W. Ma, C. R. Xing, W. J. Yan, H. Kuang, L. B. Wang and C. L. Xu, Wash-free magnetic oligonucleotide probes-based NMR sensor for detecting the Hg ion, Chem. Commun., 2011, 47, 12503–12505 RSC.
- G. Singh, K. M. Hsu, Y. J. Chen, S. C. Wu, C. Y. Chen and Y. M. Wang, A switch-on MRI contrast agent for noninvasive visualization of methylmercury, Chem. Commun., 2015, 51, 12032–12035 RSC.
- Y. Sheng, I. A. Abreu, D. E. Cabelli, M. J. Maroney, A. F. Miller and J. S. Valentine, Superoxide dismutases
and superoxide reductases, Chem. Rev., 2014, 114, 3854–3918 CrossRef CAS.
- S. Bakthavatsalam, S. Das Sharma, M. Sonawane, V. Thirumalai and A. Datta, A zebrafish model of manganism reveals reversible and treatable symptoms that are independent of neurotoxicity, Dis. Model. Mech., 2014, 7, 1239–1251 CAS.
- A. Sarkar, I. E. Biton, M. Neeman and A. Datta, A macrocyclic 19F-MR based probe for Mn2+ sensing, Inorg. Chem. Commun., 2017, 78, 21–24 CrossRef CAS.
- K. Tuschl, E. Meyer, L. E. Valdivia, N. Zhao, C. Dadswell, A. Abdul-Sada, C. Y. Hung, M. A. Simpson, W. K. Chong, T. S. Jacques, R. L. Woltjer, S. Eaton, A. Gregory, L. Sanford, E. Kara, H. Houlden, S. M. Cuno, H. Prokisch, L. Valletta, V. Tiranti, R. Younis, E. R. Maher, J. Spencer, A. Straatman-Iwanowska, P. Gissen, L. A. Selim, G. PintosMorell, W. Coroleu-Lletget, S. S. Mohammad, S. Yoganathan, R. C. Dale, M. Thomas, J. Rihel, O. A. Bodamer, C. A. Enns, S. J. Hayflick, P. T. Clayton, P. B. Mills, M. A. Kurian and S. W. Wilson, Mutations in SLC39A14 disrupt manganese homeostasis and cause childhood-onset parkinsonism-dystonia, Nat. Commun., 2016, 7, 11601–11616 CrossRef CAS.
Footnote |
† Both these authors contributed equally to this work. |
|
This journal is © the Partner Organisations 2024 |
Click here to see how this site uses Cookies. View our privacy policy here.