DOI:
10.1039/D4PY00101J
(Paper)
Polym. Chem., 2024,
15, 1962-1974
Design of functional isocyanate-free poly(oxazolidone)s under mild conditions†
Received
26th January 2024
, Accepted 13th April 2024
First published on 15th April 2024
Abstract
Polyoxazolidones, i.e. high-performance polymers bearing cyclic carbamate linkages, were recently obtained by a non-isocyanate route under mild conditions. Herein, we report the preparation of polyoxazolidones bearing thioether linkages, which offer multiple opportunities for facile chain functionalization. The process consists in the chemical upcycling of CO2-based poly(oxo-carbonate)s by aminolysis with allylamines. At room temperature, a poly(oxo-carbonate) is completely decomposed into an allyl-functionalized bis(oxazolidone) which is then copolymerized with dithiols by UV-initiated thiol–ene polymerization. The allyl-functionalized bis(oxazolidone) monomer is also quantitatively obtained by reacting an allylamine with a CO2-based bis(alkylidene cyclic carbonate). A library of poly(oxazolidone-co-thioether) copolymers is easily accessible by varying the nature of dithiol and a Mw of up to 101
000 g mol−1 is reached. All polymers are quantitatively dehydrated by simple thermal treatment at a temperature ranging from 120 °C to 140 °C in the solid state, furnishing poly(oxazolidone-co-thioether) copolymers bearing exocyclic vinylene moieties and presenting a high thermal stability (Tdeg10% up to 360 °C) and various glass transition temperatures. Post-polymerization modifications by thiol oxidation to sulfoxides or sulfones, or through S-alkylation of the thioether linkages, are realized to deliver unprecedented functional polyoxazolidones. Notably, the introduction of sulfonium groups enables the production of the first example of water-soluble polyoxazolidones. This work describes a simple platform to produce a large panel of functional polyoxazolidones that are not accessible by the current isocyanate-based methods, moreover under mild operating conditions by exploiting CO2-based monomers.
Introduction
Polyurethanes (PUs), ranking 6th among all polymers, are found in multiple applications such as foams, adhesives, coatings, and elastomers.1–3 However, most PUs still present poor resistance to heat which is considered as a serious disadvantage for some high-performance applications requiring high thermal stability.4–6 In response to this challenge, the solution was found in the development of polyoxazolidones (POxa), a specialized class of PUs distinguished by their exceptional thermal stability thanks to the presence of thermally and chemically resistant five-membered cyclic urethane linkages. Their exceptional heat resistance renders them highly promising for industrial applications that demand elevated thermal durability, e.g., in the automotive, aerospace, electrical engineering, and electronics sectors.7–12 POxa are most often produced by copolymerization of diisocyanates with polyepoxides at high temperature (typically 160 °C) in the presence of suitable catalysts.13–15 Nevertheless, diisocyanates are toxic compounds and numerous epoxides are classified as CMR (carcinogenic, mutagenic and reprotoxic) products, both causing serious harm to the environment and the human body.16,17 The high reactivity of the reagents as well as the harsh reaction conditions are also not compatible with the introduction of many functionalities (e.g., ketones and alcohols) in the polymer chains, restricting the possibilities to tune POxa properties. Although POxa are highly appealing for the development of high-performance materials, more versatile, greener, and safer synthetic approaches are searched for their production. To address this challenge, only a few studies succeeded in finding alternative pathways toward these elusive polymers, such as the polycondensation of diurethanes with diepoxides at 90 °C employing a tertiary amine as a catalyst,18 or the copper-catalyzed 4-component reaction of a dialkyne with a diamine, an aldehyde, and carbon dioxide at temperatures ranging from 75 °C to 80 °C.19,20 Some of us pioneered the utilization of novel CO2-sourced activated cyclic carbonates, i.e., α-alkylidene carbonates (αCC), for preparing isocyanate-free POxa by copolymerization of bifunctional monomers (BisαCC) with diamines under mild conditions, i.e., at room temperature (RT) and under catalyst-free conditions.21–23 The scope of POxa was also extended by Schaub's24 and Lamb's25 groups by varying the structure of BisαCC. The functional group tolerance of the reaction was proved to be feasible through copolymerization with mixtures of diamines and dithiols, providing elusive poly(oxazolidone-co-monothiocarbonate)s.26 Recently, circular POxa networks embedding dynamic N,S-acetal bonds were produced starting from αCC, diamines, and polythiols.27
BisαCC are also used to prepare poly(oxo-carbonate)s by organocatalyzed copolymerization with (biorenewable) diols,28–33 and some of them have found applications as solid electrolytes for lithium batteries.34 Interestingly, this class of polycarbonates, also prepared at RT, exhibited easy recyclability. By aminolysis with propylamine, the polymer was totally decomposed into the starting diol and an elusive bis(oxazolidone) at RT under catalyst-free conditions. The process was shown to be tolerant to alcohol functional groups and by utilizing aminopropanol, a hydroxyl functional bis(oxazolidone) was collected with high yield. The building block further served as a co-monomer for polymerization with BisαCC to deliver a polymer containing oxazolidone and oxo-carbonate linkages (thus, poly(oxazolidone-co-carbonate))35 (Scheme 1A). The facile depolymerization of poly(oxo-carbonate) involved the nucleophilic attack of a primary amine on the pendant ketone groups, leading to an hemiaminal intermediate that could undergo intramolecular cyclization to deliver the five-membered oxazolidone and the leaving alcohol. This approach of polycarbonate upcycling utilizing simple commercially available functional amines thus offered an appealing route to easily produce functional bis(oxazolidone)s re-entering the production of hybrid POxa.
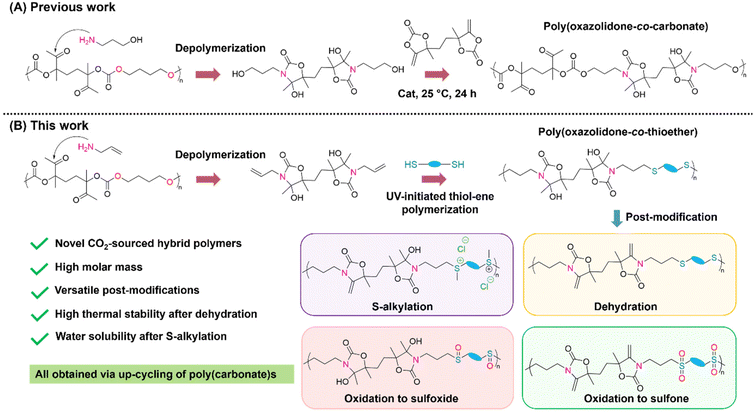 |
| Scheme 1 (A) Depolymerization of PC by aminolysis with propanolamine and its upcycling into a hybrid polyoxazolidone containing carbonate linkages;35 (B) depolymerization of PC by aminolysis with allylamine and upcycling into a polyoxazolidone containing thioether bonds via thiol–ene photopolymerization, and further modifications of the polymer. | |
In this work, we exploited this poly(oxo-carbonate) upcycling strategy to deliver an allyl functionalized bis(oxazolidone) by facile aminolysis with an allylamine. The monomer was then upcycled into hybrid POxa containing thioether linkages by radical thiol–ene photopolymerization with various dithiols (Scheme 1B). We showed that various functionalities are easily introduced into the polymer chains, i.e., pendant olefins by dehydration, sulfoxides or sulfones by partial or total oxidation of thioethers, or sulfonium by their S-alkylation. The successful polymer modifications were demonstrated by NMR and IR spectroscopies, and the thermal properties of the polymers were assessed by thermogravimetric analysis (TGA) and differential scanning calorimetry (DSC). This work enlarged the upcycling possibilities of poly(oxo-carbonate)s by utilizing commercially available reagents while giving access to new functional POxa-type polymers with tunable properties. Another alternative route was also proposed to quantitatively deliver an allyl-functionalized bis(oxazolidone) needed for the construction of hybrid POxa by direct aminolysis of a CO2-based bis(alkylidene cyclic carbonate) with an allylamine at RT.
Experimental
Synthesis of poly(oxo-carbonate) (PC)
PC was synthesized according to a procedure described before.28BisαCC (2 g, 7.86 mmol, 1 equiv.), 1,4-butanediol (0.708 g, 7.86 mmol, 1 equiv.) and DBU (60 μL, 0.401 mmol, 0.05 equiv.) were added to a reaction tube with dry DMSO (10 mL) and the mixture was stirred at 25 °C for 24 h. Then, the polymer was precipitated in diethyl ether followed by centrifugation at 10
000 rpm for 7 min and then dried under vacuum at 25 °C for 8 h. The solid was then solubilized in THF and dialyzed against THF for 24 h, followed by precipitation in diethyl ether and filtration. Finally, the polymer was dried under vacuum at 40 °C for 8 h and characterized by 1H, 13C, and HSQC NMR spectroscopy (Fig. S1–S3†) and SEC (Fig. S4†).
Depolymerization of poly(oxo-carbonate) (PC) by allylamine into bis(oxazolidone) (1)
PC (400 mg) was added in a reaction tube and dissolved in dry THF (1.2 mL). Then, 1,3,5-trimethoxybenzene (TMB) (25 mg) was added as an internal standard, followed by the addition of allylamine (395 mg, 3 equiv. vs. each ketone functionality of the polymer repeating unit). The mixture was stirred at 25 °C and depolymerization was monitored by 1H-NMR spectroscopy and SEC by sampling over time. Bis(oxazolidone) (1) was isolated by precipitation of the reaction mixture in diethyl ether followed by filtration. The solid was dried under vacuum at 40 °C for 24 h (isolated yield = 63%, 215 mg). The isolated monomer was characterized by 1H, 13C, COSY, HSQC, and HMBC NMR spectroscopy (Fig. S7–S11†).
Synthesis of bis(oxazolidone)s (1) by aminolysis of BisαCC
BisαCC (2 g, 7.86 mmol, 1 equiv.) was added to an ice bath-cooled glass vial followed by the addition of allylamine (2.24 g, 39.2 mmol, 5 equiv.). The reaction was monitored by 1H-NMR. After a 30 min reaction, the monomer was simply purified by removing the solvent under vacuum at 40 °C (isolated yield > 99%). The isolated monomer was characterized by 1H, 13C, COSY, HSQC, and HMBC NMR spectroscopy (see Fig. S7–S11†).
General procedure for the synthesis of polymers by radical thiol–ene
Bis(oxazolidone) 1 (400 mg, 1.08 mmol, 1 equiv.), dithiol 2 (1.08 mmol, 1 equiv.) and 2,2-dimethoxy-2-phenyl acetophenone (DMPA) (13.9 mg, 0.054 mmol, 0.05 equiv.) were added in a glass flask, followed by the addition of DMF (600 μL). The reaction mixture was stirred under irradiation of 365 nm UV light (Omnicure series 2000, 200 W) for 24 h. The distance of the light source to the reaction mixture was kept constant at 10 cm for each polymerization reaction. After 24 h, an aliquot of the crude product was sampled for 1H-NMR and SEC characterization. The polymer was diluted in a minimum amount of THF, precipitated in diethyl ether, and centrifuged at 10
000 rpm for 7 min. After 4 h of vacuum drying at RT, the solid was solubilized and dialyzed in THF for 24 h, followed by precipitation in diethyl ether and filtration. The pure polymer was dried under vacuum at 40 °C for 24 h and characterized by 1H, 13C, COSY, HSQC, and HMBC NMR spectroscopy (see Fig. 1, and Fig. S26–S50†) and SEC (see Table 2).
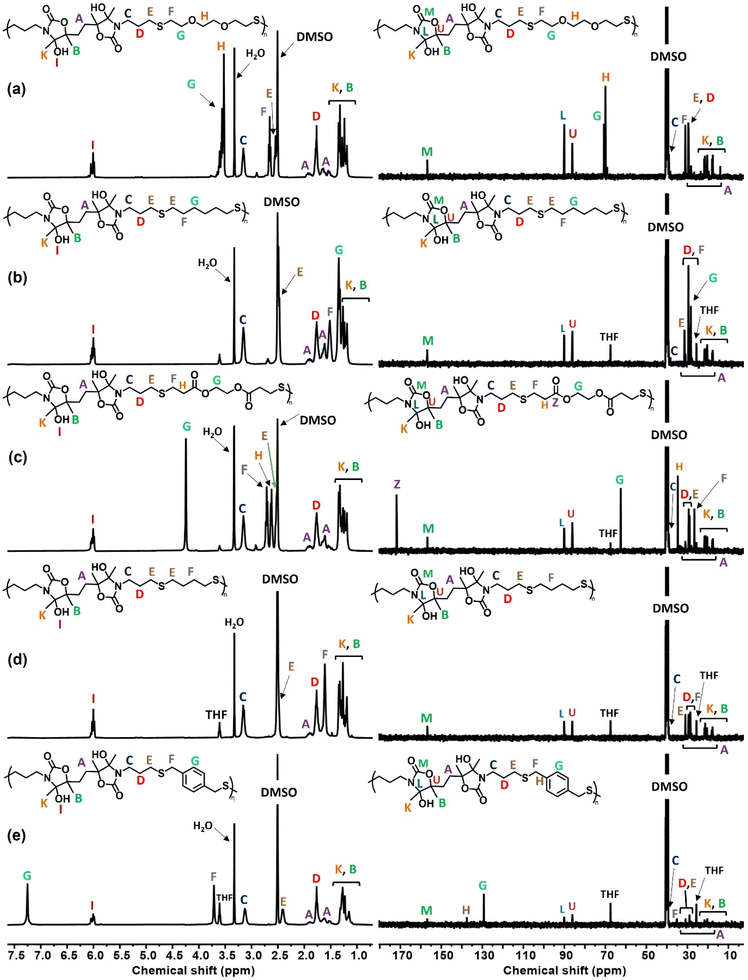 |
| Fig. 1
1H-NMR spectra (left, 400 MHz, DMSO-d6) and 13C-NMR spectra (right, 100 MHz, DMSO-d6) of purified polymers (a) P2a, (b) P2b, (c) P2c, (d) P2d, and (e) P2e. | |
Representative procedure for the dehydration of polymers
All the dehydration temperatures of polymers were determined by TGA and are presented in Table 3. The dehydration of polymers was performed according to a procedure described before.26 An oven was pre-heated to the dehydration temperature and then 100 mg of the corresponding polymer was poured onto an aluminum plate and placed in the oven for 2 h under air. The dehydrated polymers were used for characterization without any further purification. The dehydrated polymers were characterized by 1H, 13C, COSY, HSQC, and HMBC NMR spectroscopy (see Fig. S62–S86†) and SEC (see Fig. S87–S91†).
Procedures for polymer post-modification
Oxidation of thioether to sulfoxide.
Selective oxidation of P2b to sulfoxide was performed following modification of a previous procedure.36 In a glass vial, polymer P2b (100 mg, 0.19 mmol) was dissolved in 2 mL DMF. 395 μL of a 30% (w/w) H2O2 aqueous solution (10 equiv. vs. thioether, 3.84 mmol) was added, and the mixture was stirred for 72 h. The polymer was precipitated in diethyl ether followed by centrifugation at 10
000 rpm for 7 min. The solid was dried under vacuum at 25 °C for 24 h (isolated yield = 90%). The polymer was characterized by 1H, 13C, COSY, HSQC, HMBC NMR (see Fig. S115–S120†), and FTIR spectroscopy (see Fig. S121†) and SEC (see Fig. S122†).
Oxidation of thioether to sulfone.
Selective oxidation of P2b to sulfone was performed following modification of previous procedures.37,38 In a glass vial, polymer P2b (200 mg, 0.384 mmol) was dissolved in 2 mL DMF. Then, 237 μL (3 equiv. vs. thioether, 2.3 mmol) of a 30% (w/w) H2O2 aqueous solution and 6 mg (0.025 equiv.) of diphenyl diselenide (DPDS) were added and the mixture was stirred for 48 h. The polymer was precipitated in diethyl ether followed by centrifugation at 10
000 rpm for 7 min and dried under vacuum at 40 °C for 24 h and then at 60 °C for 16 h (isolated yield = 92%). The polymer was characterized by 1H, 13C, COSY, HSQC, HMBC NMR (see Fig. S124–S129†), and FTIR spectroscopy (Fig. S130†) and SEC (see Fig. S131†).
S-Alkylation of thioether.
In a glass vial, polymer P2b (200 mg, 0.384 mmol) was dissolved in 3 mL DMF. Then, methyl iodide (10 equiv. vs. thioether function, 3.84 mmol) was added, and the reaction mixture was stirred for 48 h. The resulting solution was dialyzed in a solution of 0.1 M NaCl for 2 days to exchange the counterion I− to Cl−, and then dialyzed in water for 1 day. The solution was lyophilized to obtain the polymer as a white solid (isolated yield = 70%). The polymer was characterized by 1H, 13C, COSY, HSQC, and HMBC NMR spectroscopy (see Fig. S133–S138†).
Results and discussion
Monomer synthesis
We first studied the aminolysis of a CO2-sourced poly(oxo-carbonate) (PC) using allylamine to provide a new bis(oxazolidone) with the allyl functionality. To this aim, PC was synthesized via step-growth copolymerization of BisαCC with 1,4-butanediol at 25 °C in the presence of DBU as an organocatalyst (5 mol% vs.BisαCC), following a previously described procedure28 (Scheme 2). The microstructure of PC was confirmed by 1H, 13C and HSQC NMR spectroscopy (Fig. S1–S3†) and the molar mass was determined by size exclusion chromatography (SEC; Mw = 22
900 g mol−1, Fig. S4†). The aminolysis of PC was conducted at RT in THF using allylamine (3 equiv. vs. each ketone functionality of the polymer repeating unit). The formation of the products and the extent of depolymerization were monitored by 1H-NMR spectroscopy (Fig. S5†) and SEC (Fig. S6†). After only 1 h, 1H-NMR revealed that the resonance relative to the methyl adjacent to the ketone group of PC (δ = 2.10 ppm) drastically decreased (90% of degradation). This was translated in a huge decrease of molar mass as determined by SEC, reaching a low Mw value of 850 g mol−1. 1H-NMR also revealed characteristic resonances corresponding to the expected products: the released alcohol (HO-![[C with combining low line]](https://www.rsc.org/images/entities/char_0043_0332.gif)
2– at 3.39 ppm), the oxazolidone (N-![[C with combining low line]](https://www.rsc.org/images/entities/char_0043_0332.gif)
2– at 3.76 ppm) and the imine side-product (N-![[C with combining low line]](https://www.rsc.org/images/entities/char_0043_0332.gif)
2– at 3.95 ppm; see our previous work for the mechanism of formation of this side-product35). After 24 h of reaction, the characteristic signals of PC totally disappeared, indicating complete polymer degradation (Fig. S5†). Interestingly, the determined molar composition of the products alcohol/oxazolidone/imine was 0.5/0.4/0.1. The selectivity of the oxazolidone product was thus higher compared to the depolymerization performed with propylamine in our previous work (alcohol/oxazolidone/imine was 0.5/0.33/0.17).35 The formed bis(oxazolidone) was isolated by precipitation in diethyl ether (isolated yield 63%) (Fig. S7–S11†).
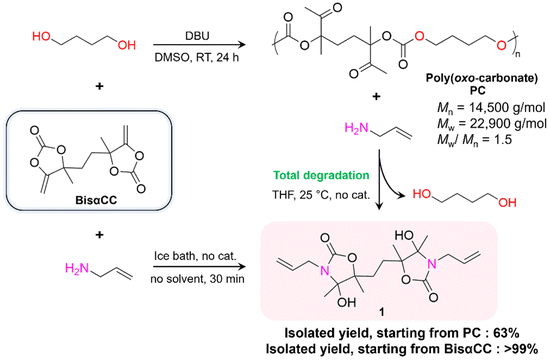 |
| Scheme 2 Aminolysis of PC into 1 and direct synthesis of 1 by aminolysis of BisαCC. | |
An alternative route to monomer 1 was the direct aminolysis of BisαCC by allylamine (Scheme 2). The reaction was monitored by 1H-NMR using 5 equiv. of allylamine as both the reagent and solvent in an ice bath under catalyst-free conditions (Fig. S12†). The results evidenced a fast aminolysis, reaching completion within 30 min, with the selective and quantitative production of bis(oxazolidone) 1 (isolated yield of >99%).
Synthesis of poly(oxazolidone-co-thioether)s
The allyl functionalized bis(oxazolidone) 1 was copolymerized with dithiol 2a by a UV-initiated radical thiol–ene reaction in the presence of a photoinitiator (Scheme 3). The experimental setup is shown in Fig. S13 and S14.† An equimolar ratio between the two co-monomers was used and a series of reaction parameters were explored to determine the optimal conditions to reach high monomer conversions and molar masses (solvent, concentration, nature of the photoinitiator). All the polymerizations were carried out at RT using a 365 nm UV lamp for 24 h. Crude reaction mixtures were analyzed by 1H-NMR spectroscopy (to determine the monomer conversion, Fig. S15, eqn (S1)†) and SEC (to determine Mw of the relative polymer). The results are summarized in Table 1.
 |
| Scheme 3 Reaction scheme for the photoinitiated thiol–ene polymerization of 1 with 2a. | |
Table 1 Optimization of the reaction conditions for the preparation of poly(oxazolidone-co-thioether)s
Entry |
Solvent |
Concentration (M) |
Initiatora (5 mol% vs. monomer 1) |
M
n b (g mol−1) |
M
w b (g mol−1) |
Dispersityb |
Conv.c (%) |
The structure of free-radical initiators is shown in Scheme S1.†
determined by SEC in DMF/LiBr calibrated with PS standards on a crude reaction mixture.
Conversion of monomer 1 calculated by 1H-NMR spectroscopy on a crude reaction mixture. Conditions: 365 nm UV light, 24 h, RT.
|
1 |
DMF |
0.5 |
DMPA |
4500 |
10 000 |
2.3 |
90 |
2 |
DMF |
1.5 |
DMPA |
16 500 |
74 000 |
4.5 |
>99 |
3 |
DMF |
1.8 |
DMPA |
27 000 |
116 000 |
4.2 |
>99 |
4 |
THF |
1.8 |
DMPA |
4500 |
7700 |
1.7 |
72 |
5 |
Acetonitrile |
1.8 |
DMPA |
5000 |
12 600 |
2.4 |
80 |
6 |
DMF |
1.8 |
Omnirad 2100 |
13 000 |
38 500 |
2.9 |
92 |
7 |
DMF |
1.8 |
Omnirad 2022 |
15 000 |
38 000 |
2.5 |
97 |
As shown in Table 1, the monomer conversion and polymer molar mass were strongly dependent on the nature of the solvent. At the same concentration (1.8 M) and with the same photoinitiator (2,2-dimethoxy-2-phenyl acetophenone; DMPA), the conversion and polymer Mw were higher in DMF (>98%; 116
000 g mol−1; entry 3) compared to those obtained in THF (72%; 7700 g mol−1; entry 4) or acetonitrile (80%; 12
600 g mol−1; entry 5). Hence, DMF proved to be the optimal solvent for this polymerization process. Decreasing the monomer concentration from 1.8 to 1.5 and 0.5 M was detrimental for the reaction (entries 1–3). Replacing DMPA by alternative photoinitiators such as Omnirad 2100 or Omnirad 2022 had adverse effects on both the conversions and the polymer molar mass (entries 6 and 7). It is worth noting that high monomer conversion resulted in highly viscous reaction mixtures, thereby impeding growing chain diffusion and efficient stirring. This phenomenon led to broad molecular weight distributions with dispersity values growing over the theoretical maximum value of 2.
Having the optimized reaction conditions in hand (DMF as the solvent, a monomer concentration of 1.8 M, DMPA as the photoinitiator), the time of UV light exposure required to reach complete monomer conversion was determined by monitoring the photopolymerization process over time using monomer 1 and dithiol 2b (Fig. S16a†) by 1H-NMR spectroscopy and SEC. The dithiol 2b was selected to simplify the 1H-NMR spectrum of the reaction compared to dithiol 2a. As shown in Fig. S16b,† the resonance associated with the allyl moiety of 1 at δ = 5.06–5.22 ppm was not detectable after 30 min reaction, confirming very high monomer consumption. After 24 h, the viscosity of the reaction medium was high, which prevented any further stirring. The time evolution of the SEC chromatogram, and thus polymer molar mass and dispersity, upon exposure to UV light is shown in Fig. S17 and S18.† After 5 h of photopolymerization, a moderate Mw of 19
000 g mol−1 was obtained (Đ = 1.9), which was significantly increased to 53
000 g mol−1 (Đ = 2.6) after 24 h of reaction.
Various dithiols 2a–2e (Scheme 4) were then evaluated for photopolymerization to illustrate the versatility of the process and to prepare a large scope of polymers (Table 2). All the polymerizations were conducted under the optimized reaction conditions at RT for 24 h. A conversion of more than 98% was obtained for P2a–d as determined by 1H-NMR on crude mixtures, evidenced by the almost complete disappearance of the olefinic peak (δ = 5.06–5.22 ppm) of monomer 1 (Fig. S19†). It is worth noting that a lower conversion of 80% was obtained for polymer P2e probably due to the low solubility of dithiol 2e in DMF. The crude polymers had moderate to high relative Mw, with values ranging from 19
000 (for P2e) to 116
000 g mol−1 (for P2a) (Table 2). The molecular characteristics of polymers after purification and their SEC chromatograms are depicted in Table S1 and Fig. S20–S25.†
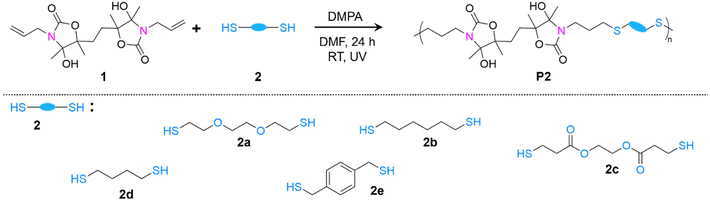 |
| Scheme 4 Synthesis of poly(oxazolidone-co-thioether)s P2a–P2e. | |
Table 2 Scope of the photoinitiated thiol–ene click polymerization of monomer 1 and different dithiols
Entry |
Polymer |
Dithiol |
M
n a (g mol−1) |
M
w
(g mol−1) |
Dispersitya |
Conv.b (%) |
Conditions: DMF (1.8 M), 2,2-dimethoxy-2-phenyl acetophenone (DMPA) 5 mol% vs. monomer 1, 365 nm UV light, 24 h, RT. Determined on crude products by SEC in DMF/LiBr calibrated with PS standards. Determined by 1H-NMR spectroscopy on crude reaction mixtures. The calculations are detailed in the ESI.† |
1 |
P2a
|
2a
|
27 000 |
116 000 |
4.2 |
>99 |
2 |
P2b
|
2b
|
19 000 |
71 000 |
3.7 |
>99 |
3 |
P2c
|
2c
|
18 000 |
54 000 |
2.9 |
>99 |
4 |
P2d
|
2d
|
22 000 |
55 000 |
2.5 |
>99 |
5 |
P2e
|
2e
|
7000 |
19 000 |
2.7 |
80 |
The polymers’ microstructures were fully characterized by NMR spectroscopy, and all 1H- and 13C-NMR spectra are depicted in Fig. 1 and Fig. S26–S50.†1H-NMR spectra demonstrated the anticipated repeating units consisting of thioether and oxazolidone linkages, with their typical resonances, e.g., S-![[C with combining low line]](https://www.rsc.org/images/entities/char_0043_0332.gif)
2 of thioethers at δ = 2.44–2.50 ppm, and N-![[C with combining low line]](https://www.rsc.org/images/entities/char_0043_0332.gif)
2 of oxazolidones at δ = 3.08–3.19 ppm as well as the resonance of the hydroxyl moiety at δ = 6.01 ppm (Fig. 1). The presence of ether linkages in polymer P2a was confirmed by the resonance at δ = 3.55 ppm of the methylene group adjacent to the ether bond (![[C with combining low line]](https://www.rsc.org/images/entities/char_0043_0332.gif)
2-O). For P2c, the presence of the ester linkages was confirmed by the adjacent methylene peak (![[C with combining low line]](https://www.rsc.org/images/entities/char_0043_0332.gif)
2-COO, δ = 2.61 ppm). The presence of the oxazolidone group was further confirmed by 13C-NMR spectroscopy, with the typical resonance of the carbonyl group at 157 ppm in all synthesized polymers. For P2c, in addition to the carbonyl group of oxazolidone, one of the ester moieties was also present at 171.85 ppm. All the polymers were soluble in DMSO, DMF and THF, and were insoluble in water (Table S2†).
Polymers’ dehydration and thermal properties
The thermal stability of all polymers was investigated using thermogravimetric analysis (TGA, Fig. S51–S55†). A two-step thermal degradation behavior was observed (see Fig. 2b, TGA curve of P2b as a representative example), a typical feature of poly(hydroxyoxazolidone)s.26 The first weight loss corresponded to the release of volatile traces and most importantly to the dehydration of hydroxyoxazolidone units in the range of 120–140 °C, resulting in the formation of an exocyclic vinylene moiety (Fig. 2a). The dehydration temperature (Tdehy) was precisely determined by applying a slow heating ramp (2 K min−1; Fig. S56–S60†), as already reported for POxa of the hydroxyoxazolidone type.26 The second weight loss was attributed to the degradation of the polymer backbone. The degradation temperature at 10% mass loss (Tdeg,10%) of the polymers was determined after the dehydration event and was in the range of 320–365 °C (Table 3 and Fig. S51–S55†).
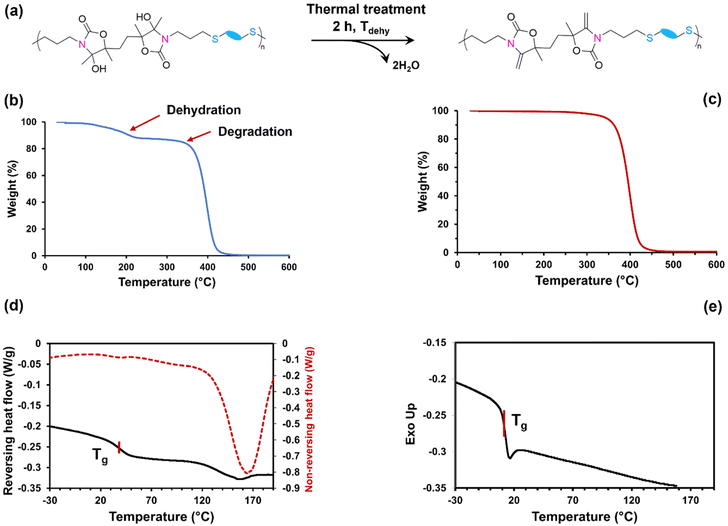 |
| Fig. 2 (a) Schematic representation of POxa dehydration; (b) TGA pattern of POxa (P2b); (c) TGA thermogram of POxa-dehydrated (P2b-dehydrated); (d) mDSC thermogram for POxa (P2b); (e) DSC thermogram of POxa-dehydrated (P2b-dehydrated). | |
Table 3 Thermal properties of POxa and POxa-dehydrated
POxa-hydrated |
POxa-dehydrated |
Entry |
Polymer |
T
deg,10% a (°C) |
T
dehy
(°C) |
T
g b (°C) |
T
deg,10%
(°C) |
T
g c (°C) |
The degradation and dehydration temperatures were determined by TGA. For POxa-hydrated, the Tdeg,10% of the polymers was determined after the first weight loss.
Glass transition temperatures were determined by modulated DSC (using the reversing heat flow curve).
Glass transition temperatures were determined by conventional DSC.
|
1 |
P2a
|
356 |
120 |
7 |
348 |
2 |
2 |
P2b
|
364 |
140 |
39 |
361 |
12 |
3 |
P2c
|
341 |
130 |
31 |
338 |
7 |
4 |
P2d
|
360 |
120 |
37 |
354 |
24 |
5 |
P2e
|
324 |
130 |
38 |
305 |
53 |
As dehydration affected the polymers’ functionality, their properties were studied and compared with polymers before dehydration. The quantitative dehydration of POxa was previously reported by treating the polymers with acetic acid23 or p-toluene sulfonic acid,25 and more recently by simple neat thermal treatment.26 Herein, we adopted the thermal treatment methodology as it permitted us to quantitatively produce the dehydrated polymers by heating POxa at their Tdehy for 2 h under air. As the dehydration process was carried out on neat POxa without requiring any dehydrating agent nor solvent, no purification step was needed after the thermal treatment to provide dehydrated POxa (coined POxa-dehydrated). The complete dehydration was confirmed by NMR spectroscopy (Fig. S61–S86†). The 1H-NMR spectra of P2b and P2b-dehydrated are depicted in Fig. S61a and S61b† as representative examples, which confirmed that the proton signal of tertiary alcohol at δ = 5.95–6.1 ppm completely disappeared, and the characteristic resonance of exocyclic olefinic protons appeared at δ = 4.07–4.36 ppm. The 13C-NMR spectra of P2b-dehydrated evidenced the typical olefinic resonance at around δ = 80 ppm and δ = 148 ppm. The SEC chromatograms for dehydrated polymers, displayed in Fig. S87–S91,† revealed a shift to a lower molar mass (Table S3†). Although chain scission may have occurred following dehydration, the variation in molecular weight observed before and after dehydration could also be attributed to changes in the hydrodynamic volume of the polymers in the solvent used for SEC analysis.
The TGA analyses of all dehydrated polymers showed a single-step degradation pattern with Tdeg,10% in the range of 305 to 361 °C (see Fig. 2c, TGA curve of P2b-dehydrated as a representative example and Fig. S92–S96† for the other polymers). The overlay of TGA thermograms for both hydrated and dehydrated P2b copolymers (Fig. S97†) further confirmed that the first degradation step of hydrated polymers was attributed to their dehydration. Overall, the TGA analyses demonstrated the high thermal stability of the dehydrated hybrid POxa, which is significantly higher than conventional non-isocyanate polyurethanes of the polyhydroxyurethane type (Tdeg,10% around 170–285 °C).39–43
POxa polymers were characterized by modulated DSC (mDSC) analyses as the Tg was difficult to detect using conventional DSC analyses (Fig. S98–S102†). This feature was already observed for other hydroxy-oxazolidone-containing polymers, i.e., poly(hydroxyoxazolidone-co-thiocarbonate)s26 and poly(oxazolidone-co-carbonate)s,35 which were characterized by two broad and weak Tgs. For the herein synthesized polymers, no second Tg could be determined due to possible overlapping with early dehydration. As observed in Fig. 2d, the reversing contribution of the heat flow allows the determination of the Tg of all polymers in the range of 7–39 °C, and the dehydration event is observed at a higher temperature in the non-reversing contribution of the heat flow. For POxa-dehydrated, one strong Tg could be detected by conventional DSC, ranging from 2 to 53 °C depending on the polymer structure (Fig. S103–S107†). Although a rational trend is not trivial between Tg and the chemical structure in hydrated polymers, the Tg of dehydrated analogs seemed directly related to the rigidity of the dithiol structures, i.e., a low Tg for P2a containing ether linkages bringing increased chain flexibility and the highest Tg for P2e containing an aromatic moiety.
Mechanical properties
Insights into the mechanical properties of poly(oxazolidone)s before and after dehydration were gained through tensile testing. P2a, selected for its high molecular weight as determined by SEC, underwent compression molding at 70 °C, a temperature below the dehydration threshold. Experiments were conducted on dogbone-shaped specimens that were fabricated using the die-cutting technique (Fig. S108†). Despite its low Tg (7 °C as determined by DSC), P2a exhibited elastic behavior with a Young's modulus value of 398 MPa and a yield strength of 11.4 MPa. The material then underwent plastic deformation until rupture, with a stress at break of 7.6 MPa and a strain at break of 314% (Fig. S109†). Interestingly, the same experiment conducted on the dehydrated polymer P2a-dehydrated revealed heat flow when exposed to stress, making tensile testing impossible. This striking difference between hydrated and dehydrated polymers might be ascribed to intermolecular hydrogen bonding exclusively present in the hydroxyl-containing material.
Chemical stability
The resistance of poly(oxazolidone)s to acidic and basic environments was assessed by immersing P2a in sulfuric acid and sodium hydroxide (NaOH) aqueous solutions of varying concentrations (0.1 M, 1 M and 5 M) at room temperature for a period of 48 h (Fig. S110†). The polymer has shown great stability under acidic conditions, with no apparent chain scission as determined by SEC (Fig. S111†). However, exposure to acid-triggered partial dehydration led to a conversion in hydroxyl groups of 23% at a concentration of 0.1 M and up to 71% of conversion accompanied by some Wagner–Meerwein rearrangement of the resulting alkene at a concentration of 5 M (Fig. S112†).23 In contrast, the polymer integrity was affected under basic conditions. In 0.1 M NaOH aqueous solution, the polymer remained intact as determined by SEC and NMR (Fig. S113 and Fig. S114†). Unexpectedly, the sample immersed in 1 M NaOH exhibited solubility, and subsequent SEC analysis of the solution revealed a shift toward a longer elution time, suggesting chain scission events. However, isolation of the product proved to be challenging and would necessitate further investigation on the stability of these polymers under alkaline conditions. Although the same result was expected when the polymer was introduced into the 5 M NaOH solution, SEC analysis didn't reveal any change in elution times and the 1H-NMR spectrum remained unchanged from that of the pure polymer. Overall, the poly(oxazolidone)s synthesized herein seemed stable under acidic conditions, although some dehydration of the hydroxyl group is expected with increasing acid content. However, the material integrity was found to be compromised in specific basic environments.
Post-polymerization modifications
All POxa were characterized by the presence of thioether linkages within their backbone, offering multiple possibilities for further modifications, notably by oxidation to sulfoxide or sulfone as well as by alkylation to sulfonium.36,44 The polymer P2b was selected as a representative polymer for all post-polymerization modifications.
Oxidation.
We first explored the oxidation of the thioether linkages into sulfoxides or sulfones. The experiments were conducted at room temperature in DMF as solvent. Aqueous H2O2 (10 equiv. vs. the thioether repeating unit) was used for the selective and quantitative mono-oxidation of P2b to sulfoxide (P2b-sulfoxide). Reaction completion was confirmed after 72 h by 1H-NMR, where the characteristic resonance of the methylene (![[C with combining low line]](https://www.rsc.org/images/entities/char_0043_0332.gif)
2-S–) of thioether at δ = 2.43–2.49 ppm totally disappeared. A new peak corresponding to the methylene protons adjacent to the sulfoxide groups (–SO-![[C with combining low line]](https://www.rsc.org/images/entities/char_0043_0332.gif)
2) at δ = 2.57–2.81 ppm appeared45,46 (Fig. S115† and Fig. 3a and b). To further confirm the quantitative sulfoxide formation, 1H-NMR was also conducted in DMF-d7 as typical signals of the thioether function overlapped with the peak attributed to DMSO-d6 (Fig. S120, see ESI† for more details). No peak attributed to sulfone groups were observed, confirming that overoxidation did not occur under these experimental conditions. As P2b-sulfoxide was only soluble in DMF and DMSO, complete removal of residual solvent was not possible. The IR spectrum of P2b-sulfoxide also revealed the appearance of sulfoxides’ (SO) stretching band at ∼998 cm−1 (Fig. S121†).45 The SEC chromatogram presented the same pattern as that of P2b with only a slight decrease in elution time, suggesting no chain scission events (Fig. S122†).
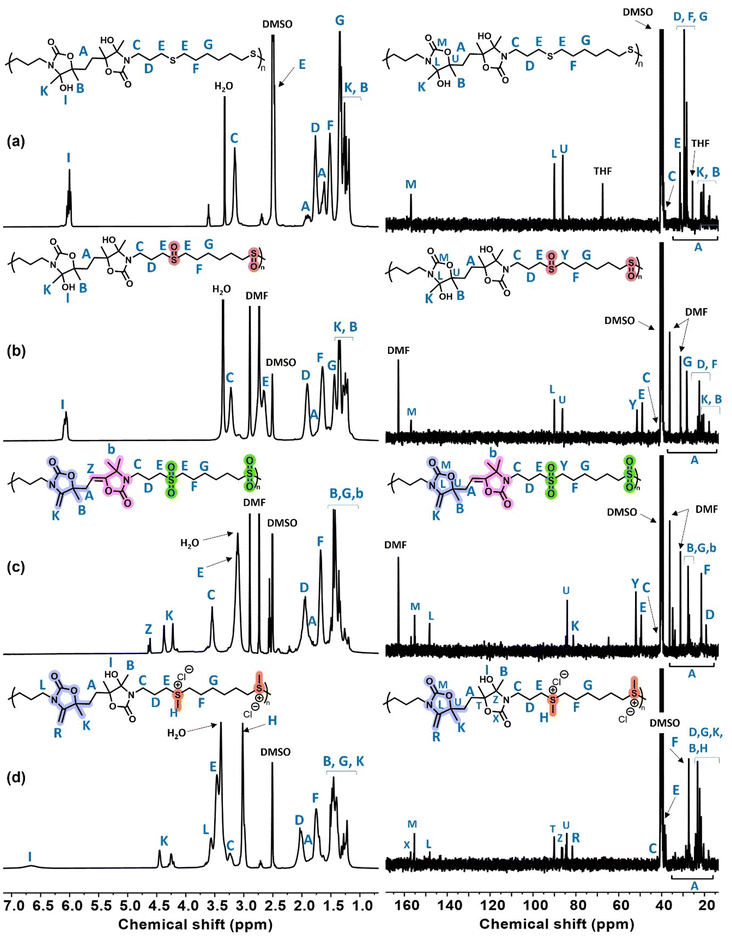 |
| Fig. 3
1H-NMR spectra (left, 400 MHz, DMSO-d6) and 13C-NMR spectra (right, 100 MHz, DMSO-d6) of (a) P2b, (b) P2b-sulfoxide, (c) P2b-sulfone and (d) P2b-sulfonium. | |
Tirelli et al. used a diphenyldiselenide (DPDS) catalyst in combination with H2O2 for the double oxidation of poly(propylene sulfide) to poly(propylene sulfone).37 Herein, we adopted the same methodology to oxidize the thioether linkages into sulfones. This was achieved by using 3 equiv. of H2O2 per thioether repeating unit and 0.025 equiv. of DPDS as the catalyst in DMF at RT. By monitoring the oxidation over time by 1H-NMR, it appeared that the thioethers were fully oxidized to sulfones after 48 h of reaction, and partial dehydration of the hydroxyoxazolidone moieties (10 mol% of functions) was observed (Fig. S123†).
P2b
-sulfone was purified by precipitation of the reaction mixture in diethyl ether, followed by drying under vacuum at 40 °C for 24 h. As the amount of residual DMF remaining in the polymer was high, the polymer was further dried at 60 °C under vacuum for 16 h. This mild thermal treatment was, however, enough to fully dehydrate the polymer. Moreover, a Wagner–Meerwein rearrangement was observed following the second thermal treatment resulting in the formation of β-alkylidene oxazolidone linkages (20 mol% of oxazolidone) (Fig. S124†). For further details and in-depth information regarding this rearrangement, we recommend referring to the article published by our group.23 The complete oxidation of P2b to P2b-sulfone was characterized similarly using NMR and FTIR. 1H-NMR in DMSO-d6 indicated that the thioether peak at δ = 2.43–2.49 ppm completely disappeared and a new downfield peak attributed to protons adjacent to sulfone at δ = 3.01–3.20 ppm appeared (Fig. 3c and S124†).47 The proton signals corresponding to the tertiary alcohol group, observed at δ = 5.95–6.1 ppm, vanished entirely, while the distinctive resonance of exocyclic olefinic protons emerged within the range of δ = 4.11–4.44 ppm, confirming the complete dehydration of the polymer. The olefinic proton associated with the β-alkylidene oxazolidone linkage was observed at δ = 4.59–4.66 ppm, in alignment with the chemical shift observed in analogous structures investigated in a prior study.23 To corroborate the quantitative formation of sulfone groups, an additional verification was conducted using 1H-NMR in DMF-d7 as the solvent (Fig. S129, see ESI† for more details). The two sulfone stretching frequencies could be observed by FTIR as two peaks at ∼1200 cm−1 and ∼1278 cm−1 as shown in Fig. S130.† No signal correlated with the sulfoxide was observed in 1H-NMR nor FTIR, indicating that double oxidation of the thioether to sulfones was selective. The SEC trace for P2b-sulfone showed a shift to a lower molar mass after oxidation (Fig. S131†). This might be the result of different hydrodynamic volumes of the polymers before and after modification (dehydration and oxidation of the thioether) or some chain scission.
TGA was also employed to assess the thermal stability of the two oxidized polymers, which were both characterized by a multi-step degradation pattern (Fig. S132†). The initial weight reduction observed between 100 and 200 °C was attributed to the evaporation of residual DMF and dehydration of the polymer. However, it is obvious that the thermal stability of P2b-sulfoxide is lower than that of P2b as already observed in other sulfoxide-containing polymers.36,45,47 While P2b and P2b-sulfoxide were completely decomposed at 600 °C, sample P2b-sulfone produced 15 wt% of char at that temperature. We did not perform DSC analyses as remaining DMF within the polymers might have a drastic plasticizing effect on chain mobility.
S-Alkylation
As thioethers are reactive towards alkyl halides and form hydrophilic sulfonium salts, we investigated this reaction for introducing sulfonium groups into POxa. We selected iodomethane (10 equiv. vs.P2b unit) for the S-alkylation of P2b in DMF at RT. After 48 h, the quantitative formation of the sulfonium group was confirmed by 1H-NMR with the characteristic resonance of the methylene (![[C with combining low line]](https://www.rsc.org/images/entities/char_0043_0332.gif)
2-S–) of thioether at δ = 2.43–2.49 ppm that disappeared at the benefit of two new resonances at δ = 3.12–3.29 ppm and 3 ppm for the methylene (CH2-S+) and the methyl (CH3-S+) of the sulfonium group, respectively (Fig. 3d and Fig. S133†). A partial dehydration (50 mol% of hydroxyoxazolidone) was also observed with the characteristic resonance of exocyclic olefinic protons at δ = 4.18–4.48 ppm. To our delight, the polymer was found to be soluble in water, being the first water-soluble POxa reported so far. Further confirmation of quantitative S-alkylation was performed using 1H-NMR characterization in D2O (Fig. S138, see ESI† for more details). A multistep degradation pattern for P2b-sulfonium was observed by TGA (Fig. S139†) that should correspond to dehydration (between 100 and 200 °C) and backbone degradation. However, a comprehensive elucidation of the degradation pathway falls beyond the scope of this study.
DSC characterization (Fig. S140†) showed Tg at 35 °C, as well as an endotherm at around 120 °C. The latter might be attributed to the dehydration of the remaining hydroxyoxazolidone moieties that occurred in this temperature range (Table 3).
Conclusion
In this study, the chemical recycling of CO2-sourced poly(oxo-carbonate)s was achieved under mild conditions through aminolysis with allylamines, resulting in the recovery of a new bis(hydroxyoxazolidone) monomer featuring allyl functional groups. This monomer was copolymerized with various commercially available dithiols via a photoinitiated thiol–ene click reaction at room temperature, delivering elusive poly(oxazolidone)-type polymers containing thioether functions, i.e., poly(oxazolidone-co-thioether)s. Optimal reaction conditions were investigated through solvent, initiator, and reaction time adjustments, leading to poly(oxazolidone)-type copolymers with a Mw of up to 101
000 g mol−1. All the polymers exhibited an amorphous nature with a Tg spanning from 7 °C to 39 °C. These polymers displayed a two-step thermal decomposition pattern, with the first step associated with polymer dehydration in the temperature range of 120–140 °C and the second step attributed to the degradation of the polymer backbone within the range of 324–364 °C. Following a straightforward thermal treatment at the dehydration temperature for 2 h, the polymers underwent complete dehydration, resulting in the formation of polyoxazolidones enriched with exocyclic olefin moieties. This dehydration occurred in the solid state and did not require any solvent nor dehydrating agent, making the process facile and easily upscalable. The dehydrated polymers presented a high degradation temperature (305–361 °C) and a single Tg spanning from 2 °C to 53 °C. The post-modification of poly(oxazolidone-co-thioether)s was further assessed, focusing on the selective single or double oxidation of thioether linkages into sulfoxides or sulfones, as well as the alkylation of thioether to yield sulfonium derivatives. The introduction of sulfonium moieties delivered the first example of a water-soluble poly(oxazolidone). This research exemplifies the strategic utilization of functional monomers derived from the degradation of polycarbonates for the synthesis of a diverse range of novel functional poly(oxazolidone)-type polymers, whose properties have to be investigated in a forthcoming work. Importantly, the allyl-functional bis(hydroxyoxazolidone) needed for their synthesis can also be quantitatively produced by reacting allylamines with the CO2-sourced bis(alkylidene cyclic carbonate), offering perspective for facile upscaling.
Conflicts of interest
The authors declare no competing financial interest.
Acknowledgements
The authors acknowledge the financial support provided by the NIPU-EJD project; this project has received funding from the European Union's Horizon 2020 research and innovation program under the Marie Skłodowska-Curie grant agreement no 955700. The authors of Liege thank FNRS for financial support in the frame of the CO2 Switch project under grant T.0075.20. They also thank FNRS and FWO for funding the EOS project “BIOFACT” no O019618F (ID EOS: 30902231). C. D. is a F.R.S.-FNRS Research Director. H. S. acknowledges the financial support from el Ministerio de Ciencia e Innovación from TED2021-129852B-C22 funded by MCIU/AEI/10.13039/501100011033 and by the European Union NextGeneration EU/PRTR and the grant PID2022-138199NB-I00 funded by MCIU/AEI/10.13039/501100011033.
References
- Y. Deng, R. Dewil, L. Appels, R. Ansart, J. Baeyens and Q. Kang, Reviewing the thermo-chemical recycling of waste polyurethane foam, J. Environ. Manage., 2021, 278, 111527 CrossRef CAS PubMed
.
- J. O. Akindoyo, M. Beg, S. Ghazali, M. Islam, N. Jeyaratnam and A. Yuvaraj, Polyurethane types, synthesis and applications–a review, RSC Adv., 2016, 6(115), 114453–114482 RSC
.
- H. W. Engels, H. G. Pirkl, R. Albers, R. W. Albach, J. Krause, A. Hoffmann, H. Casselmann and J. Dormish, Polyurethanes: Versatile materials and sustainable problem solvers for today's challenges, Angew. Chem., Int. Ed., 2013, 52(36), 9422–9441 CrossRef CAS PubMed
.
- S.-H. Liu, M.-Y. Shen, C.-F. Kuan, H.-C. Kuan, C.-Y. Ke and C.-L. Chiang, Improving thermal stability of polyurethane through the addition of hyperbranched polysiloxane, Polymers, 2019, 11(4), 697 CrossRef CAS PubMed
.
- E. Delebecq, J.-P. Pascault, B. Boutevin and F. Ganachaud, On the versatility of urethane/urea bonds: reversibility, blocked isocyanate, and non-isocyanate polyurethane, Chem. Rev., 2013, 113(1), 80–118 CrossRef CAS PubMed
.
-
M. Szycher, Szycher's handbook of polyurethanes, CRC press, 1999 Search PubMed
.
- V. Sendijarevic, A. Sendijarevic, H. Lekovic, H. Lekovic and K. C. Frisch, Polyoxazolidones for high temperature applications, J. Elastomers Plastics, 1996, 28(1), 63–83 CrossRef CAS
.
- V. Sendijarevic, A. Sendijarevic, K. C. Frisch and P. Reulen, Novel isocyanate–based matrix resins for high temperature composite applications, Polym. Compos., 1996, 17(2), 180–186 CrossRef CAS
.
- V. A. Pankratov, T. M. Frenkel and A. Fainleib, 2-Oxazolidinones, Russ. Chem. Rev., 1983, 52(6), 576 CrossRef
.
- N. Kinjo, S. I. Numata, T. Koyama and T. Narahara, Synthesis and viscoelastic properties of new thermosetting resins having isocyanurate and oxazolidone rings in their molecular structures, J. Appl. Polym. Sci., 1983, 28(5), 1729–1741 CrossRef CAS
.
- T. Pelzer, B. Eling, H.-J. Thomas and G. A. Luinstra, Toward polymers with oxazolidin-2-one building blocks through tetra-n-butyl-ammonium halides (Cl, Br, I) catalyzed coupling of epoxides with isocyanates, Eur. Polym. J., 2018, 107, 1–8 CrossRef CAS
.
- M. Kitayama, Y. Isda, F. Odaka, S. Anzai and K. Irako, Synthesis and properties of polyoxazolidone elastomers from diepoxides and diisocyanates, Rubber Chem. Technol., 1980, 53(1), 1–13 CrossRef CAS
.
- A. Bakry, R. Aversano, L. D’Ilario, V. Di Lisio, I. Francolini, A. Piozzi and A. Martinelli, Flexible aliphatic poly (isocyanurate–oxazolidone) resins based on poly (ethylene glycol) diglycidyl ether and 4, 4′–methylene dicyclohexyl diisocyanate, J. Appl. Polym. Sci., 2016, 133(19), 43404 CrossRef
.
- M. Azechi and T. Endo, Synthesis and property of polyoxazolidone having fluorene moiety by polyaddition of diisocyanate and diepoxide, J. Polym. Sci., Part A: Polym. Chem., 2014, 52(12), 1755–1760 CrossRef CAS
.
- H. J. Altmann, M. Clauss, S. König, E. Frick-Delaittre, C. Koopmans, A. Wolf, C. Guertler, S. Naumann and M. R. Buchmeiser, Synthesis of Linear Poly (oxazolidin-2-one) s by Cooperative Catalysis Based on N-Heterocyclic Carbenes and Simple Lewis Acids, Macromolecules, 2019, 52(2), 487–494 CrossRef CAS
.
- A. Gomez-Lopez, F. Elizalde, I. Calvo and H. Sardon, Trends in non-isocyanate polyurethane (NIPU) development, Chem. Commun., 2021, 57(92), 12254–12265 RSC
.
- L. Ehrenberg and S. Hussain, Genetic toxicity of some important epoxides, Mutat. Res., Rev. Genet. Toxicol., 1981, 86(1), 1–113 CrossRef CAS PubMed
.
- Y. Iwakura, S. I. Izawa and F. Hayano, Polyoxazolidones prepared from bisurethans and bisepoxides, J. Polym. Sci., Part A-1: Polym. Chem., 1966, 4(4), 751–760 CrossRef CAS
.
- Q. Wu, J. Chen, X. Guo and Y. Xu, Copper(I)–Catalyzed Four–Component Coupling Using Renewable Building Blocks of CO2 and Biomass–Based Aldehydes, Eur. J. Org. Chem., 2018, 2018(24), 3105–3113 CrossRef CAS
.
- D. Teffahi, S. Hocine and C.-J. Li, Synthesis of oxazolidinones, dioxazolidinone and polyoxazolidinone (a new polyurethane) via a multi component-coupling of aldehyde, diamine dihydrochloride, terminal alkyne and CO2, Lett. Org. Chem., 2012, 9(8), 585–593 CrossRef CAS
.
- S. Gennen, B. Grignard, T. Tassaing, C. Jérôme and C. Detrembleur, CO2−Sourced α–Alkylidene Cyclic Carbonates: A Step Forward in the Quest for Functional Regioregular Poly (urethane) s and Poly (carbonate) s, Angew. Chem., 2017, 129(35), 10530–10534 CrossRef
.
- C. Ngassam Tounzoua, B. Grignard and C. Detrembleur, Exovinylene Cyclic Carbonates: Multifaceted CO2−Based Building Blocks for Modern Chemistry and Polymer Science, Angew. Chem., Int. Ed., 2022, 61(22), e202116066 CrossRef CAS PubMed
.
- T. Habets, F. Siragusa, B. Grignard and C. Detrembleur, Advancing the synthesis of isocyanate-free poly (oxazolidones) s: Scope and limitations, Macromolecules, 2020, 53(15), 6396–6408 CrossRef CAS
.
- S. Dabral, U. Licht, P. Rudolf, G. Bollmann, A. S. K. Hashmi and T. Schaub, Synthesis and polymerisation of α-alkylidene cyclic carbonates from carbon dioxide, epoxides and the primary propargylic alcohol 1, 4-butynediol, Green Chem., 2020, 22(5), 1553–1558 RSC
.
- A. R. Wong, M. Barrera, A. Pal and J. R. Lamb, Improved Characterization of Polyoxazolidinones by Incorporating Solubilizing Side Chains, Macromolecules, 2022, 55(24), 11006–11012 CrossRef CAS
.
- T. Habets, F. Siragusa, A. J. Müller, Q. Grossman, D. Ruffoni, B. Grignard and C. Detrembleur, Facile construction of functional poly (monothiocarbonate) copolymers under mild operating conditions, Polym. Chem., 2022, 13(21), 3076–3090 RSC
.
- T. Habets, G. Seychal, M. Caliari, J.-M. Raquez, H. Sardon, B. Grignard and C. Detrembleur, Covalent adaptable networks through dynamic N, S-acetal chemistry: toward recyclable CO2-based thermosets, J. Am. Chem. Soc., 2023, 145, 25450–25462 CrossRef CAS PubMed
.
- F. Siragusa, E. Van Den Broeck, C. Ocando, A. J. Müller, G. De Smet, B. U. Maes, J. De Winter, V. Van Speybroeck, B. Grignard and C. Detrembleur, Access to biorenewable and CO2-based polycarbonates from exovinylene cyclic carbonates, ACS Sustainable Chem. Eng., 2021, 9(4), 1714–1728 CrossRef CAS
.
- F. Siragusa, J. Demarteau, T. Habets, I. Olazabal, K. Robeyns, G. Evano, R. Mereau, T. Tassaing, B. Grignard, H. Sardon and C. Detrembleur, Unifying Step-Growth Polymerization and On-Demand Cascade Ring-Closure Depolymerization via Polymer Skeletal Editing, Macromolecules, 2022, 55(11), 4637–4646 CrossRef CAS
.
- C. Ngassam Tounzoua, B. Grignard, A. Brege, C. Jerome, T. Tassaing, R. Mereau and C. Detrembleur, A catalytic domino approach toward oxo-alkyl carbonates and polycarbonates from CO2, propargylic alcohols, and (mono-and di-) alcohols, ACS Sustainable Chem. Eng., 2020, 8(26), 9698–9710 CrossRef CAS
.
- F. Siragusa, C. Detrembleur and B. Grignard, The advent of recyclable CO 2-based polycarbonates, Polym. Chem., 2023, 14(11), 1164–1183 RSC
.
- M. Lanzi and A. W. Kleij, Recent advances in the synthesis and polymerization of new CO2-based cyclic carbonates, Chin. J. Chem., 2024, 42, 430–443 CrossRef CAS
.
- Y.-F. Zhang, W.-M. Lai, S. Xie, H. Zhou and X.-B. Lu, Facile synthesis, structure and properties of CO 2-sourced poly (thioether-co-carbonate) s containing acetyl pendants via thio-ene click polymerization, Polym. Chem., 2022, 13(2), 201–208 RSC
.
- F. Ouhib, L. Meabe, A. Mahmoud, N. Eshraghi, B. Grignard, J.-M. Thomassin, A. Aqil, F. Boschini, C. Jérôme, D. Mecerreyes and C. Detrembleur, CO 2-sourced polycarbonates as solid electrolytes for room temperature operating lithium batteries, J. Mater. Chem. A, 2019, 7(16), 9844–9853 RSC
.
- F. Siragusa, T. Habets, R. Méreau, G. Evano, B. Grignard and C. Detrembleur, Catalyst-Free Approach for the Degradation of Bio-and CO2-Sourced Polycarbonates: A Step toward a Circular Plastic Economy, ACS Sustainable Chem. Eng., 2022, 10(27), 8863–8875 CrossRef CAS
.
- J. M. Sarapas and G. N. Tew, Poly (ether–thioethers) by thiol–ene click and their oxidized analogues as lithium polymer electrolytes, Macromolecules, 2016, 49(4), 1154–1162 CrossRef CAS
.
- F. El Mohtadi, R. d'Arcy, X. Yang, Z. Y. Turhan, A. Alshamsan and N. Tirelli, Main chain polysulfoxides as active ‘stealth'polymers with additional antioxidant and anti-inflammatory behaviour, Int. J. Mol. Sci., 2019, 20(18), 4583 CrossRef CAS PubMed
.
- P. C. B. Page, B. R. Buckley, C. Elliott, Y. Chan, N. Dreyfus and F. Marken, Chemoselective oxidation of sulfides to sulfoxides with urea–hydrogen peroxide complex catalysed by diselenide, Synlett, 2015, 80–82 CrossRef
.
- G. R. Younes, M. Kamel, H. M. Titi, M. Farkhondehnia and M. Marić, Sugar-based thermoplastic polyhydroxyurethanes: effects of sorbitol and mannitol diastereomers on polymer properties and applications in melt blending, ACS Appl. Polym. Mater., 2022, 4(7), 5161–5172 CrossRef CAS
.
- R. Jaratrotkamjorn, A. Nourry, P. Pasetto, E. Choppé, W. Panwiriyarat, V. Tanrattanakul and J. F. Pilard, Synthesis and characterization of elastomeric, biobased, nonisocyanate polyurethane from natural rubber, J. Appl. Polym. Sci., 2017, 134(42), 45427 CrossRef
.
- M. Bourguignon, B. Grignard and C. Detrembleur, Water–Induced Self–Blown Non–Isocyanate Polyurethane Foams, Angew. Chem., Int. Ed., 2022, 61(51), e202213422 CrossRef CAS PubMed
.
- D. J. Fortman, J. P. Brutman, C. J. Cramer, M. A. Hillmyer and W. R. Dichtel, Mechanically activated, catalyst-free polyhydroxyurethane vitrimers, J. Am. Chem. Soc., 2015, 137(44), 14019–14022 CrossRef CAS PubMed
.
- G. R. Younes and M. Marić, Bio-based thermoplastic polyhydroxyurethanes synthesized from the terpolymerization of a dicarbonate and two diamines: design, rheology, and application in melt blending, Macromolecules, 2021, 54(21), 10189–10202 CrossRef CAS
.
- J. Yook, D. Jeong and J.-C. Lee, Synthesis of Citronellol-Derived Antibacterial Polymers and Effect of Thioether, Sulfoxide, Sulfone, and Ether Functional Groups on Their Bactericidal Activity, Macromolecules, 2023, 56, 3406–3420 CrossRef CAS
.
- B. Yan, Y. Zhang, C. Wei and Y. Xu, Facile synthesis of ROS-responsive biodegradable main chain poly (carbonate-thioether) copolymers, Polym. Chem., 2018, 9(7), 904–911 RSC
.
- P. K. Dannecker, U. Biermann, A. Sink, F. R. Bloesser, J. O. Metzger and M. A. Meier, Fatty Acid–Derived Aliphatic Long Chain Polyethers by a Combination of Catalytic Ester Reduction and ADMET or Thiol–Ene Polymerization, Macromol. Chem. Phys., 2019, 220(4), 1800440 CrossRef
.
- L. Infante Teixeira, K. Landfester and H. Thérien-Aubin, Selective Oxidation of Polysulfide Latexes to Produce Polysulfoxide and Polysulfone in a Waterborne Environment, Macromolecules, 2021, 54(8), 3659–3667 CrossRef CAS PubMed
.
Footnote |
† Electronic supplementary information (ESI) available: Materials and instrumentation, additional information about optimization reactions, solubility tables, NMR spectra, SEC chromatograms, TGA & DSC analyses, FT-IR spectra, and a setup for the photopolymerization reaction. See DOI: https://doi.org/10.1039/d4py00101j |
|
This journal is © The Royal Society of Chemistry 2024 |