DOI:
10.1039/D4MH00975D
(Review Article)
Mater. Horiz., 2024,
11, 5533-5549
Clay–polymer hybrid hydrogels in the vanguard of technological innovations for bioremediation, metal biorecovery, and diverse applications
Received
26th July 2024
, Accepted 2nd August 2024
First published on 15th August 2024
Abstract
Polymeric hydrogels are among the most studied materials due to their exceptional properties for many applications. In addition to organic and inorganic-based hydrogels, “hybrid hydrogels” have been gaining significant relevance in recent years due to their enhanced mechanical properties and a broader range of functionalities while maintaining good biocompatibility. In this sense, the addition of micro- and nanoscale clay particles seems promising for improving the physical, chemical, and biological properties of hydrogels. Nanoclays can contribute to the physical cross-linking of polymers, enhancing their mechanical strength and their swelling and biocompatibility properties. Nowadays, they are being investigated for their potential use in a wide range of applications, including medicine, industry, and environmental decontamination. The use of microorganisms for the decontamination of environments impacted by toxic compounds, known as bioremediation, represents one of the most promising approaches to address global pollution. The immobilization of microorganisms in polymeric hydrogel matrices is an attractive procedure that can offer several advantages, such as improving the preservation of cellular integrity, and facilitating cell separation, recovery, and transport. Cell immobilization also facilitates the biorecovery of critical materials from wastes within the framework of the circular economy. The present work aims to present an up-to-date overview on the different “hybrid hydrogels” used to date for bioremediation of toxic metals and recovery of critical materials, among other applications, highlighting possible drawbacks and gaps in research. This will provide the latest trends and advancements in the field and contribute to search for effective bioremediation strategies and critical materials recovery technologies.
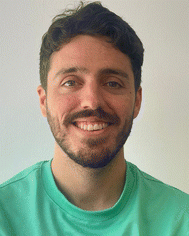
Miguel A. Ruiz-Fresneda
| Miguel A. Ruiz-Fresneda obtained his PhD in microbiology from the University of Granada (UGR), Spain in 2019. His thesis was honored with the Outstanding Doctorate Award (Escuela de Posgrado UGR). He currently works as a postdoctoral researcher in the Department of Microbiology at UGR, focusing on identifying microorganisms of significant interest for their application in the bioremediation of contaminated environments. He has contributed with 20 publications in high-impact international journals in the field of bioremediation. He is also a reviewer for several journals indexed in the JCR, such as the Journal of Hazardous Materials, Microbial Biotechnology, and Microbial Cell Factories. |
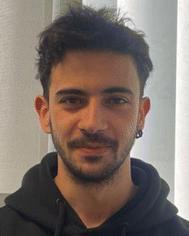
Eduardo González-Morales
| Eduardo González Morales is a PhD student in Fundamental and Systems Biology at the University of Granada (Spain). His research focuses on the development and application of natural hydrogels with immobilized microorganisms for bioremediation and the biorecovery of critical materials. |
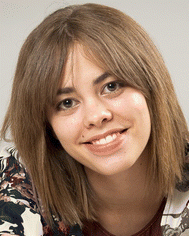
Cristina Gila-Vilchez
| Cristina Gila-Vilchez obtained her PhD in Physics from the University of Granada, Spain, in 2022, where she focused on the synthesis and characterization of magnetic particles and composite hydrogels. She currently works as a postdoctoral fellow at the Conditions Extrêmes et Matériaux: Haute Température et Irradiation (CEMHTI – CNRS) laboratory in France. Her research now is focused on the study of light propagation and light–matter interaction in random media for the development of smart optical devices. |
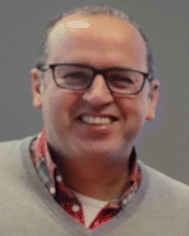
Mohamed L. Merroun
| Mohamed L. Merroun is a Professor of Microbiology at the University of Granada (Spain). He obtained his PhD degree at Department of Microbiology, University of Granada. He worked as Senior Researcher at the Helmholtz-Zentrum Dresden Rossendorf, Germany. He is the head of Geomicrobiology and Biogeochemistry Research Group. He is an active user of Radiation Synchrotron Facilities (e.g. ESRF, Soleil). He has published more than 110 peer-reviewed papers. Prof. Merroun research interests are focused on bioremediation of heavy metal/radionuclide contaminated sites, bionanotechnology, microbiology of deep geological disposal of radioactive wastes, geomicrobiology, and microbial recovery of critical materials. |
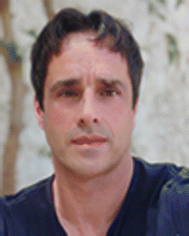
Antonio L. Medina-Castillo
| Antonio L. Medina Castillo received his PhD degree in Chemistry from the University of Granada (Spain) in 2011. In 2011, he founded the start-up company Nanomaterials and Polymer S.L. (NanoMyP®), where he continued his research career as Chief Scientific Officer (CSO) from 2011 to 2021, working on the design of smart nanostructured polymer materials and their application in biomedicine, catalysis, sensor technology, and controlled drug delivery. In 2021 he joined the Department of Analytical Chemistry at the University of Granada (Spain) where he is currently developing his research line based on the design of nanostructured smart materials for optoelectronic applications. |
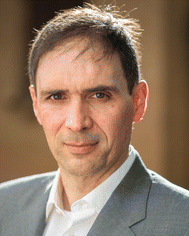
Modesto T. Lopez-Lopez
| Modesto T. Lopez-Lopez obtained his PhD in Physics from the University of Granada, Spain, in 2005. He was a postdoctoral fellow at the LPMC, a CNRS-University of Nice Sophia Antipolis joint laboratory, from 2006 to 2008. In 2008, he joined the University of Granada's Department of Applied Physics, where he became an Associate Professor in 2012 and a full Professor in 2019. Prof. Lopez-Lopez's research focuses on composite hydrogels, encompassing the synthesis and functionalization of particles, investigation of hydrogel mechanical properties and self-assembly processes, and exploration of sol–gel transitions. |
Wider impact
This review critically examines recent advancements in clay–polymer hybrid hydrogels, emphasizing their application in bioremediation and biorecovery, while identifying existing drawbacks and research gaps in the field. The incorporation of natural clay composites is being investigated for enhancing their mechanical properties, stability, and biocompatibility. Abiotic clay-based hydrogels have been studied more extensively than biotic ones for their application in remediation, medicine, and industry. However, cell immobilization offers an eco-friendly methodology not only associated with heavy metal removal, but also with the recovery of value-added products in line with the principles of circular economy. This is due to the potential of microorganisms to enzymatically transform contaminants into non-toxic nanoparticles of great interest. We suggest the use of biofilm-forming bacteria, as these structures seem to be involved in enhancing cell surviving and mechanical properties of hydrogels. Filamentous fungi must also be further investigated since their hyphal network structure could allow them to more easily reach nutrients and contaminants within the polymeric matrix. Finally, the influence of magnetite on the mechanical properties and biocompatibility of hydrogels should be studied, as it presents itself as a very useful tool in the recovery of hydrogels through the application of magnetic fields.
|
1. Introduction
Hydrogels are polymeric network matrices with high water absorption capacity. The materials composing these hydrogels could be inorganic (e.g. ceramics, clays, etc.) and organic (e.g. agarose, chitosan, alginate, etc.). Organic polymers are mostly preferred due to their inherent biocompatibility, availability, low cost, and because many of them are environmentally friendly.1 However, there are some major disadvantages associated with both natural and synthetic organic polymers, including mechanical weakness, low reproducibility, and chemical instability.1 To overcome these drawbacks, the combination of non-harmful inorganic compounds, such as clays, with natural organic polymers, known as hybrid hydrogels, seems to be an effective solution.2 Nanoclays offer new opportunities for hydrogel design as they can be used as physical cross-linkers of polymers, resulting in hydrogels with better dynamic properties (self-assembly and self-healing) and greatly enhanced mechanical stiffness and toughness.1,3–5 In addition, bentonite clay minerals can improve the biological properties of hydrogels by providing inert support surfaces for the formation of microbial biofilms. Their relative low-cost, easy fixation of bacteria, durability, swelling capacity, and high mechanical strength allow them to be used in packed or fluidized bed reactors.6 The improvement of the mechanical and biocompatibility properties of clay-based hybrid hydrogels is leading to their exhaustive investigation for numerous medical and industrial applications including drug delivery, tissue engineering, oil recovery, and agricultural applications.7–10 In recent years, their use in environmental decontamination of heavy metals and organic pollutants is also gaining special attention. Today, much research is focused on the use of hybrid hydrogels for physico-chemical remediation, mainly through the processes of adsorption, sedimentation or chemical precipitation.11–13 However, the incorporation of microorganisms into their polymeric matrices with the aim of improving the pollutant removal efficiency has not been studied as much.
Microorganisms have developed tolerance mechanisms (e.g. bioaccumulation, biosorption, precipitation, and biotransformation) through evolutionary pressure, enabling them to thrive in highly polluted environments.14,15 All these biological tolerance mechanisms can be of great interest for the bioremediation of polluted environments, as they can immobilize heavy metals reducing their solubility and toxicity. Beyond their role in bioremediation, microorganisms could also be applied for the biorecovery of critical elements such as selenium (Se), palladium (Pd), or platinum (Pt) from acidic leachates of electronic scrap and mining wastes. This is due to their ability to produce metallic nanoparticles (NPs) for use in various industries, such as electronics and catalysis, within the framework of circular economy.16–18 The use of planktonic or free-living cells has been a standard for heavy metal bioremediation due to their ease of handling and lower cost. However, in recent years, the immobilization of microbial biomass has received a great deal of attention. Immobilization is defined as the natural or artificial encapsulation that limits the mobility of the cell or any biocatalyst within an aqueous system, while maintaining their viability and metabolic activity.19,20 Cell immobilization enhances resistance to heavy metal toxicity and adverse environmental conditions, enhanced mechanical stability and metabolic activity, and easy biomass retention.21,22 One of the main methods used in cell immobilization for bioremediation purposes is cell entrapment within porous hydrogels composed of natural or synthetic cross-linked polymers. Polymers of natural origin (e.g., agarose, chitosan, alginate, etc.) are mostly preferred for the immobilization of cells due to their inherent biocompatibility, availability, and low cost.1
The present review provides a comprehensive analysis of the most recent advances and promising results of hybrid clay–polymer composite hydrogels for their application in many fields, with a special focus on bioremediation and biorecovery of heavy metals and critical elements. The enhancements provided by inert micro- or nano-clay composites in the mechanical properties of hydrogels and the immobilization of microorganisms make them potential tools for various biological applications. Both biotic and abiotic clay-based hydrogels are thoroughly compared in their use for remediation of heavy metals and other contaminants. Novel research aspects, including the use of biofilm-forming bacteria and filamentous fungi for immobilization in hydrogels, are also highlighted due to their enormous rheological and biological potential, respectively. Finally, future perspectives, trends, gaps, and possible drawbacks in the field are thoroughly discussed.
2. Hybrid hydrogels
Hydrogels are three-dimensional (3D) polymer networks that can absorb and retain large amounts of water. In the multidisciplinary field involving the synthesis of hydrogels, the concept of “hybrid hydrogel” is ambiguously used to refer to two types of materials with different physical structures and properties:23–27 (i) hydrogels whose physical structure is a homogeneous semi-solid solution (single-phase hydrogels); (ii) hydrogels whose physical structure is a heterogeneous semi-solid dispersion (composite hydrogels). In this review, we propose a simple classification of hydrogels based on their chemical composition and physical structure, establishing a well-differentiated line between hydrogels based on homogeneous semi-solid solutions and those based on heterogeneous semi-solid dispersion (Fig. 1).
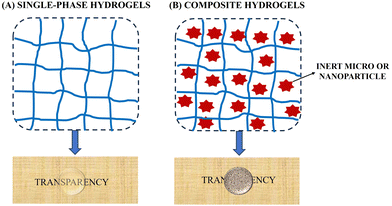 |
| Fig. 1 Classification of hydrogels based on their chemical composition and physical structure: (A) single-phase hydrogels based on homogeneous semi-solid solutions and (B) composite hydrogels based on heterogeneous semi-solid dispersion including inert micro- or nanoparticulate solid materials. | |
2.1. Single-phase hydrogels
Single-phase hydrogels consist of polymeric networks swollen by water-based fluids, which may also contain soluted polymers intertwined at the molecular scale within the polymeric network. According to their chemical composition, they may be classified into three groups: (a) inorganic hydrogels, composed of inorganic polymers and crosslinkers; (b) organic hydrogels, composed of organic polymers and crosslinkers; and (c) mixed hydrogels, composed of a mixture of at least one organic and one inorganic element (polymer or crosslinker). It is well-known that single-network hydrogels exhibit low Young's modulus, low tensile and compressive strength, and low fracture energy, also showing a slow response to swelling.24
In the last decades with the aim to enhance the mechanical properties and swelling/deswelling response of single-network hydrogels, multicomponent networks have been designed. Multicomponent polymer networks are polymeric blends of two or more polymers in a network form, with at least one such polymer polymerized and/or crosslinked in the presence of the other(s). Therefore, based on their physical structure hydrogels can be classified as follows:23 (i) hydrogels based on a single network (SNs), in which a bond or a short sequence of bonds joins one polymeric chain to another, creating a percolated mesh that on a macroscopic scale can be considered as a single molecule with a molecular weight approaching infinity.28 (ii) Hydrogels based on interpenetrating polymer networks (IPNs), that comprise two or more 3D networks that are at least partially interlaced on the molecular scale but not covalently bonded to each other. (iii) Hydrogels based on semi-interpenetrating polymer networks (SIPNs), that comprise one or more polymer networks and one or more linear or branched polymers, that are characterized by the penetration on a molecular scale of at least one of the networks by at least some of the linear or branched polymers29
Biopolymers are attracting increasing attention in recent years, with their use for the preparation of INPs and SIPNs hydrogels being an area requiring intense research in the near future. Either by leveraging their native intermolecular interactions or by chemical modifications, biopolymers can be used to form multicomponent networks (IPNs or SIPNs). An advantage of biopolymers is that many of them exhibit inherent properties such as bioactivity, degradability, and biocompatibility.30 Despite their advantages, biopolymers also have drawbacks such as weaker mechanical properties, wider distributions in molecular weights, undefined chemical compositions, etc.31 The advances in functionalization and crosslinking chemistry of biopolymers developed in the last decades have enhanced the biopolymer-based hydrogel properties.5 However, the required properties for many applications have not yet been achieved since these materials can often negatively impact encapsulated cells, undergo phase separation, and fast degradation of their mechanical properties,5 all of them being aspects that should be urgently addressed in near-future research.
2.2. Composite hydrogels
Composite hydrogels are characterized by the presence of inert micro- and/or nanoparticulates (Inert-μ-NP) dispersed within the polymer network.
In composite hydrogels, the Inert-μ-NP are distributed between the meshes of the 3D polymeric networks forming a heterogeneous semi-solid dispersion (weak interactions between the Inert-μ-NP and the 3D polymeric network). The introduction of the micro- and/or nanoparticles of inorganic materials in a polymeric matrix to fabricate composite hydrogels endows them with novel functionalities arising from the combination of the soft swollen characteristics of the matrix and the properties of the particles. For example, some hydrogels are made responsive to external stimuli, such as pH, light, heat, or magnetic field by the introduction of particles. We refer to these hydrogels as stimuli-responsive hydrogels.25–27 It should be noticed, nevertheless, that the introduction of particles is not a requirement to endow hydrogels with stimuli-responsiveness, as there are many polymeric systems that are sensitive to different stimuli such as pH and temperature e.g., agarose hydrogels, pluronic hydrogels, and polyacrylamide hydrogels. Due to their composition being like that of biological tissues, one of the applications of hydrogels is as a support medium in biological applications. However, the toughness of conventional hydrogels is about two orders of magnitude smaller than that of natural living tissues,28 and here as well the use of composite hydrogels can be an approach to improving the mechanical properties.4,29,30,32,33 However, special care should be taken to use particles with adequate functionalization so that they can serve as additional anchoring points, enhancing the crosslinking density or functionality of the crosslinking points.4 When a mismatch between the surface functionalization of the particles and the polymer materials exists, the result is a weakening or even phase-separation of the resulting hydrogels provoked by the defects created by the embedded particles.32 A particular case is that of polymer hydrogels crosslinked uniquely by the dispersed particles, known as grafting-onto method.31 For example, nanoclays can be used as physical cross-linkers of polymers, resulting in hydrogels that combine the dynamic properties (self-assembly and self-healing) associated to physical hydrogels with greatly enhanced mechanical stiffness and toughness (composite hydrogels).5,34,35 Unfortunately, regardless of the method, most existing composite hydrogels become very brittle at large particle loading, so manufacturing mechanically strong hydrogels at high particle loading is still an unsolved challenge.36
The modulation of the interactions between particles and polymer filaments by the nature of the particle coating shell may also have a direct impact on the microstructure of the hydrogels4,30,37,38 (Fig. 2). Of relevance is the preparation of anisotropic hydrogels by making use of the responsiveness of particles to external fields, with the aim of mimicking the well-defined hierarchical architecture oriented anisotropically at the macroscopic scale of biological systems. In this sense, Sharma et al. (2018)39 demonstrated that crosslinking nickel magnetic nanowires to collagen allowed a bidirectional alignment of collagen matrices when processed in a uniform magnetic field. A similar strategy was used by Shi and Coradin (2020),38 resulting in the successful preparation of anisotropic collagen hydrogels by the alignment of magnetic microfibers in the pre-gel solution by external magnetic fields.
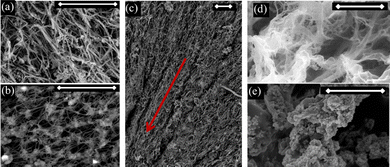 |
| Fig. 2 Impact of particles in the microstructure of hydrogels. (a)–(c) SEM images of fibrin hydrogels: without MNPs (a), with MNPs (b), with MNPs and crosslinking under a magnetic field (c) remark anisotropy in the magnetic field direction (arrow). (d) and (e) SEM images of peptide hydrogels: without MNPs (d), with MNPs (e). Bar length: (a)–(e): 10 μm. Note: MNPs (magnetic nanoparticles). | |
In the field of bioremediation, composite hydrogels offer the additional advantage of providing a solid support for bacterial cell immobilization. For example, Zvulunov et al. (2019)2 immobilized bacteria in polyethyleneimine–clay composites hydrogels and demonstrated the potential of the strategy for the absorption and biodegradation of formaldehyde from water. Clays from the group of bentonites are mainly composed of smectites, which are phyllosilicate minerals of volcanic origin. Smectite is characterized by a three-layer 2
:
1 TOT (tetrahedral–octahedral–tetrahedral) laminar structure consisting of two tetrahedral silica sheets interleaved by an octahedral aluminium sheet. Their high surface-to-volume ratio due to their small particle size, along with their high cation exchange capacity and negative layer charge, provides them with excellent physicochemical properties for industrial use.40 Specifically, clay formations have been reported for their high plasticity, thermal conductivity, low permeability, swelling capacity, and exceptional rheological and hydraulic properties, among others. All these characteristics make clays important not only for their novel use as nanocomposites, but also in numerous other applications including catalysis processes, water treatment, civil engineering, animal nutrition, drilling muds, as adsorbents, etc.41 In addition, clay minerals can enhance the biocompatibility of hydrogels as inert support surfaces for the attachment of microbial cells and biofilm formation.6 For example, bentonite clay formations have been shown to be a natural habitat for a wide diversity of microorganisms, as indicated by numerous studies.42–45 In fact, several microorganisms isolated from these geological formations have been described for their potential to be used in bioremediation and biorecovery, thanks to their ability to interact with selenium, uranium, and other toxic elements.46–48 An advantage of clay minerals such as bentonite in bioremediation strategies is that they not only offer a support for the cells, but also this is achieved at a relatively low-cost. Combining biocompatibility with durability and high mechanical strength (discussed above) we can use them in packed or fluidized bed reactors.6
In conclusion, it is evident that single-phase hydrogels, both organic and inorganic based hydrogels, as well as mixed ones, exhibit different advantages and drawbacks depending on the targeted application. However, the addition of micro- and nanoparticles to these hydrogels to form composite hydrogels seems to offer a wide range of advantages including enhanced swellability and mechanical properties as well as further possibilities of functionalization of these materials, that could be useful in numerous applications, including hydrogels with immobilized microbial cells. Therefore, the screening for new nanomaterials that allow us to develop composite hydrogels with suitable physical, chemical, and biological properties becomes imperative.
3. Bacterial immobilization methods in hydrogels
Free-living planktonic microbial cells have been traditionally used in scientific research and biotechnological applications due to their ease of handling and lower cost. Nevertheless, in recent times, the adoption of immobilized cells in supporting polymeric matrices or surfaces has gained interest as a promising approach due to its numerous advantages for their final application. The microenvironments created within these matrices can enhance the preservation of cellular integrity by providing protection against extreme physicochemical conditions.49 It would also provide a controlled system that would prevent cell release into the media, and allow substrate diffusion, facilitating the final biotechnological application. Several other practical benefits, including convenient transport and handling, reusing, as well as the ease of separating and recovering cells and accumulated products within the matrix, contribute to enhanced efficiency for economic and functional scale-up applications. Hydrogels are attracting a lot of attention as one of the most suitable polymeric networks for cell immobilization. This is mainly due to the osmotic pressure exerted by the water, which promotes the interaction among the microorganisms, the polymer forming the gel, and the target agent (contaminant, drug, etc.).1 Immobilization of living cells within solid supports is a major challenge in biotechnology and biomedical science. However, it raises important challenges in terms of chemistry as the conditions of matrix formation must be compatible with the survival of the organisms. Most of the research on microorganism immobilization using hydrogels involves the use of toxic and expensive chemical compounds (e.g., glutaraldehyde, organic acids, calcium-containing substances, etc.). In today's world, where pollution levels are increasingly higher, there is a need for more research on microorganisms immobilized on “green and sustainable” hydrogels, where most of their components are ecological and environmentally friendly. This would not only be beneficial for the environment, but also promote the development and survival of immobilized microbial cells. As we have previously mentioned, one of the composites with significant potential for use in biohydrogels are nanoclays. This natural material not only enhances the mechanical properties of the gels, but also serves as the natural habitat for a wide variety of microorganisms with bioremediation potential,46,47,50 thereby facilitating their support and growth. The use of these natural origin composites for the production of ecological hydrogels would not only be beneficial in cell immobilization, but also in preventing environmental pollution and saving costs in waste management processes.
Today, several methods of cell immobilization in hydrogels are under investigation, including entrapment, adsorption on surfaces, encapsulation, or covalent bonding, among others51,52 (Fig. 3).
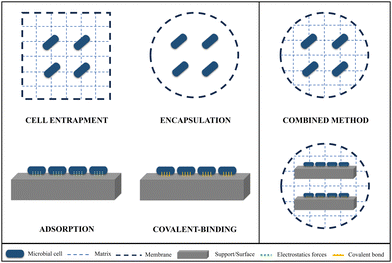 |
| Fig. 3 Illustrative scheme showing different methodologies used for microbial cell immobilization. | |
Cell entrapment is a versatile method employed to confine microbial, animal, or algal cells within a porous gel composed of natural or synthetic polymers53 (Fig. 3). Cross-linking plays a pivotal role in the entrapment method. It involves creating bonds between polymer chains, which, in turn, restricts movement and transforms a solution of polymers into a solid-like polymer gel. Therefore, cross-linking provides the necessary structural and mechanical support to maintain the integrity and functionality of the immobilization matrix. This network structure prevents the release of cells into the surrounding medium and allows for the diffusion of substrates and products while safeguarding the microorganisms. Cell entrapment also offers advantages such as a substantial cellular volume, operational ease, high cell viability, and the strength of immobilized cell spheres.54
Encapsulation approach confines the cells within a capsule space formed by semi-permeable membrane walls, which enable the transfer of nutrients and substrates from the capsule's outer region to its interior55 (Fig. 3). The diffusional properties and the restricted volume of the capsules induce the entrance of water reducing the chance for an osmotic shock. In comparison to entrapment techniques, this process involves a slight procedural variation that allows free movement of the cells within the core space of the capsule, which can improve cell metabolic activity and growth.56 Encapsulation within beads is one of the most frequently employed methods for cell immobilization. Nonetheless, encapsulation comes with its own set of limitations, including the potential fragility of capsules, instability at certain pH levels, and dissolution in buffer and other solutions.56,57
Adsorption is a method consisting of cell fixation on solid surfaces through weak electrostatic forces58 (Fig. 3). Given the low strength and reversible interactions participating in this process, it is more likely for the cells to detach from the support and potentially escape into the surrounding environment. However, some cells have the capability to develop biofilms that confer them stronger binding to the supporting material and protection against adverse environmental conditions. Furthermore, adsorption is of a simple and cost-effective nature, enabling direct interaction between immobilized cells and nutrients. This leads to elevated levels of mass transfer and substrate conversion efficiency.55 The adsorption process is influenced by several key factors including the bacterial physiological state and characteristics and the existing conditions in the surrounding medium (composition, pH, etc.). Finally, properties of the supporting matrix, such as the size, structure, or presence of pores also have a notable impact. To address some of the challenges associated with the adsorption process, covalent binding has emerged as a method which establishes strong and stable bonds between the functional groups (–COOH, –NH2, –SH) of the cells and solid surfaces (Fig. 3). Therefore, cross-linking processes are also crucial for this immobilization method. In that case, the leakage of cells is minimal, and the stability of the system is very high.1,53 However, the bacterial bioavailability and hence activity is significantly decreased due to the utilization of toxic chemical compounds as cross-linking agents (e.g., glutaraldehyde), which further diminishes cellular activity, often resulting in cell death.1,59 For this reason, covalent binding is more effective for enzyme adhesion than for whole bacterial cells.
To overcome their disadvantages and find an ideal and effective immobilization method, combined procedures employing more than one technique are also being studied (Fig. 3). One of the most popular combined immobilization technologies consists of the entrapment-encapsulation in spherical beads, through the encapsulation of microorganisms in a cross-linked polymeric matrix.57,60 The spherical shape provides a higher surface-to-volume ratio compared to other morphologies, granting them a larger contact surface area with the contaminant agent. Furthermore, they afford physical support and protection against other stressors, promoting the biological activity of the immobilized microorganisms. Other approaches involve cell entrapment or encapsulation combined with covalent-binding or adsorption to a solid surface.61,62 A wide diversity of combined innovative techniques is currently under investigation to enhance the traditional immobilization processes mentioned earlier. These investigations are mainly focused on the improvement of the mechanical and diffusional properties, porosity, and strength of the carriers employed. One of the most commonly used polymers for hydrogel formation is polyvinyl alcohol (PVA), which is capable of self-gelation in aqueous solutions. However, the solidification is slow and is formed by weak interactions (hydrogen bonds), so in most cases, the gels are not optimal for cell immobilization. To enhance and expedite the PVA or other polymers gelation process, several innovative methodologies are being employed. The freeze–thaw method, for instance, is a physical process based on temperature fluctuations that induce stronger cross-linking through the formation of electrostatic forces between polymer chains.63 Nevertheless, conducting polymerization below 0 °C could have a negative impact on cell activity. The utilization of LentiKats® technology (lens-shaped carrier made of PVA) has emerged as a room temperature alternative that does not employ hazardous compounds as cross-linker, thus not affecting cellular viability. Their lens-shaped morphology allows for effective diffusion, and their high internal porosity makes them exceptional for cell and enzyme immobilization. They also exhibit good physical and mechanical properties including low abrasion and elasticity.64 The effectiveness of this immobilization system has been demonstrated in different studies regarding bioremediation and biotechnology.65,66 In addition, Lentikats® technology has been demonstrated to be scalable for industrial use, which is the main advantage in contrast to other immobilization methods. Electrospinning is another environmentally friendly technique in which the polymer solution containing microorganisms is decomposed in nano-microfibers through the application of electrostatic forces.67 The formed polymeric fibers have a great potential for cell immobilization due to a superior surface-area ratio and mechanical strength.68 Summarizing, the results shown in the literature reveal the enormous potential of combined microbial immobilization methodologies over traditional ones, and an increased need for research in this field.
4. From physico-chemical to biological remediation using composite hydrogels
Physical and chemical techniques including sedimentation, chemical precipitation, or adsorption have been traditionally used in water and soil decontamination and critical metal recovery. One of the most widely accepted techniques is adsorption, which has been demonstrated to be an efficient, easy to implement, cost-effective, and non-contaminant strategy.69 Composite hydrogels have been widely used as adsorption matrices because of their properties and capacity of sequestering different kinds of pollutants including heavy metals, drugs, or dyes (Table 1). Different strategies combining clays (as micro- or nano- particulate composites) and organic polymers have been developed to create composite hydrogels for physico-chemical remediation and adsorption of these kinds of pollutants.
Table 1 Abiotic composite hydrogels for physico-chemical remediation purposes
Matrix composition (inorganic component) |
Matrix composition (organic component) |
Contaminant |
Removal efficiency |
Ref. |
P(AAm-co-AN)-AgNO3 |
Karaya gum |
Crystal violet |
99% |
70
|
Graphene oxide/carbon nanotubes |
Alginate |
Antibiotics |
200 mg g−1 |
71
|
Cysteine–bentonite |
Alginate/PVA |
Pb(II) |
995 μmol g−1 |
12
|
Polyacrylamide |
Carboxymethyl cellulose |
Cu(II) |
73.80% |
72
|
Pb(II) |
90.80% |
Cd(II) |
70.20% |
Magnetic bentonite |
Carboxymethyl chitosan/alginate |
Cu(II) |
92.62% |
11
|
pSiDm copolymer |
Jute cellulose nanocrystals |
Methylene blue |
88% |
73
|
Kaolin and montmorillonite clay |
Chitosan |
Cu(II) |
86% |
74
|
Clay |
Alginate–cellulose |
Pb(II) |
90% |
75
|
CeO2–MoO3–SiO2(CH2)3 |
Alginate |
Cr(VI) |
151.96 mg g−1 |
76
|
Mn(II) |
122.66 mg g−1 |
Clay |
Alginate–Saponin |
Cr(VI) |
99.70% |
77
|
Cu(II) |
99.50% |
Graphene oxide |
Alginate |
Cr(III) |
>60% |
78
|
Pb(II) |
Dellite 43B clay |
Chitosan |
Carbendazim |
>0.24 mg g−1 |
79
|
Clay |
Chitosan |
Cr(VI) |
50.90% |
80
|
Pb(II) |
39.50% |
Kaolin clay |
Polyacrylic acid |
Ni(II) |
14.40 mg g−1 |
81
|
Bentonite |
Chitosan/acrylic acid |
Cu(II) |
88.50 mg g−1 |
82
|
Zn(II) |
72.90 mg g−1 |
Cd(II) |
51.50 mg g−1 |
Graphene oxide |
Alginate |
Mn(II) |
>55% |
83
|
Montmorillonite |
Cellulose |
Methylene blue |
277 mg g−1 |
84
|
Bentonite |
PVA |
Methylene blue |
26.30 mg g−1 |
85
|
Halloysite |
Chitin |
Pb(II) |
8.20 mg g−1 |
86
|
Cu(II) |
4.20 mg g−1 |
Cd(II) |
2.10 mg g−1 |
Spirulina-modified montmorillonite |
Chitosan |
Cr(VI) |
3333 mg g−1 |
87
|
Hectorite |
Sodium alginate |
Cu(II) |
160.28 mg g−1 |
88
|
Polydopamine-coated montmorillonite |
Hexacyanoferrate |
Cs(I) |
173 mg g−1 |
89
|
Montmorillonite |
Agar/maltodextrin/PVA |
Methylene blue |
71.51 mg g−1 |
90
|
LAPONITE® |
AMPS/acrylic acid |
Methylene blue |
1385 mg g−1 |
91
|
LAPONITE® |
Poly(amidoxime)/gelatine/cellulose nanofiber |
Uranium |
8.62 mg g−1 |
92
|
Montmorillonite |
Acrylamide/acrylic acid–calcium lignosulfonate |
Methylene blue |
492.70 mg g−1 |
93
|
Cysteine-modified bentonite |
PVA/alginate |
Pb(II) |
995 μmol g−1 |
12
|
Bentonite–vermiculite |
PVA/poly acrylic acid |
Ni(II) |
238.51 mg g−1 |
94
|
Pb(II) |
357.06 mg g−1 |
Methylene blue |
343.74 mg g−1 |
Montmorillonite |
Chitosan |
Methyl green |
303.21 mg g−1 |
95
|
Montmorillonite |
PVA/chitosan |
As(V) |
69.64 mg g−1 |
13
|
Montmorillonite |
APMS/carboxymethyl cellulose |
Methylene blue |
490.50 mg g−1 |
96
|
Kaolinite |
Carboxymethyl chitosan |
Cr(VI) |
205 mg g−1 |
97
|
Pb(II) |
125 mg g−1 |
One of the most studied dyes is Methylene Blue (MB), a dye mainly coming from the textile industry, which has adverse effects on aquatic systems. For MB removal, Wang et al. (2019)84 synthesized cellulose/montmorillonite hydrogels with 277 mg g−1 capacity of adsorption. Sanchez et al. (2019)85 created PVA hydrogels with 3 wt% bentonite for MB removal and posterior reuses (5 cycles) using 0.5 M of KCl in 50
:
50 of water/ethanol mixture as the most effective desorption solution. Similarly, Nguyen et al. (2021)90 incorporated montmorillonite into agar/maltodextrin/poly(vinyl alcohol) hydrogel membranes with best results of uptake capacity (71.51 mg g−1) for 20 wt% of clay. Moreover, they obtained with these composite hydrogels good recyclability (5 cycles) and easy desorption with water and ethanol eluent at 40
:
60 volume ratio.
Another environmental problem faced in research using adsorptive composite hydrogels is the water pollution caused by heavy metals such as copper (Cu), cadmium (Cd), lead (Pb) and arsenic (As). In this regard, Nguyen et al. (2019)86 developed chitin hydrogel films containing halloysite nanoclay up to 4 wt%. These hydrogels showed better tensile strength with the addition of the clay and enhanced removal capacity of Pb (8.2 mg g−1), Cu (4.2 mg g−1), and Cd (2.1 mg g−1). Moreover, Tekay et al. (2019)87 modified montmorillonite with Spirulina biosorbent and dispersed it into chitosan hydrogels. Therefore, the pores of the hydrogels presented receptor groups for the adsorption of Cr(VI) ions. Thanks to the Spirulina modification, the adsorption capacity of Cr(VI) was enhanced compared to Spirulina, montmorillonite and chitosan hydrogels alone, obtaining a 78 mg g−1 adsorption capacity with 1% of modified clay and with an initial Cr(VI) concentration of 200 ppm. Hexavalent actinides such as uranium (U) have also been reported to be remediated with abiotic composite hydrogels. For example, Liu et al. (2021)92 reduced uranium from seawater by high adsorbent double-network hydrogels composed of poly(amidoxime), gelatin, cellulose nanofiber and LAPONITE®. The hydrogels showed high mechanical strength and the addition of the clay increased the uranium uptake amount in 73.4%, with a uranium adsorption rate of 0.345 mg g−1 day−1. Finally, composite hydrogels are also used for the adsorption and remediation of other toxic compounds like pesticides. For instance, Baigorria et al. (2022)79 removed the fungicide carbendazim from contaminated waters using chitosan/montmorillonite hydrogels which presented a maximum adsorption capacity of 0.4618 mg g−1. This effect is much higher compared to the individual chitosan hydrogel, thus the clay having a key role in the adsorption capacity of the composite hydrogel.
Despite the good results obtained by abiotic hydrogels, the use of biological procedures for pollutant decontamination, known as bioremediation, is gaining attention due to their improved properties. While the optimization of cell immobilization procedures for manufacturing hybrid composite hydrogels have been studied for years, their environmental application in the fields of bioremediation and critical material biorecovery have not been investigated so far (Table 2) (Fig. 4). Indeed, literature surveys show that the number of studies on biotic clay–polymer composite hydrogels for bioremediation (Table 2) is much lower compared to abiotic ones (Table 1). Biotic hydrogels offer an eco-friendly approach that promotes a circular economy, as microorganisms not only remove contaminants but can also transform them into valuable products of industrial interest (Fig. 4).
Table 2 Microorganisms immobilized within composite hydrogels for bioremediation purposes
Matrix composition (organic/inorganic) |
Immobilization method |
Immobilized microorganism |
Contaminant |
Removal efficiency |
Ref. |
PVA/alginate/bentonite clay |
Entrapment/encapsulation |
Bacillus fusiformis
|
Naphthalene |
99.70% |
98
|
PVA/Na-alginate/kaolin |
Entrapment/encapsulation |
Sphingomonas sp. GY2B |
Phenol |
99.50% |
99
|
PVA/alginate/clay mix |
Entrapment/encapsulation |
Pseudomonas stutzeri
|
Crude oil hydrocarbons |
74% |
100
|
Rhodococcus qingshengii
|
67% |
Polyethyleneimine/montmorillonite clay |
Adsorption |
Pseudomonas putida
|
Formaldehyde |
— |
2
|
Iron oxide/alginate |
Encapsulation |
Agrobacterium fabrum
|
Methylene blue |
45% |
101
|
PVA/poly(p-xylylene) (PPX) |
Wet spinning |
Nitrobacter winogradskyi
|
Nitrite |
>99% |
102
|
Magnetic silica/alginate |
Entrapment/encapsulation |
Pseudomonas fluorescens
|
Rhodamine B dye 99 |
86–99% |
103
|
PVA/Na-alginate/bentonite |
Encapsulation |
Pseudomonas aeruginosa
|
Hydrocarbons |
99% |
104
|
Bacillus subtilis
|
Ralstonia pickettii
|
Silica/alginate |
Entrapment/encapsulation |
Stereum hirsutum
|
Malachite green |
80% |
105
|
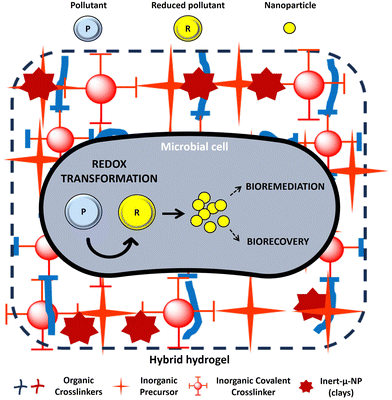 |
| Fig. 4 Schematic illustration of hybrid clay/polymer hydrogels with immobilized bacteria, which offer a green and effective methodology for bioremediation of toxic compounds and their biorecovery as valuable products. | |
It is important to emphasize that most studies employing composite hydrogels with immobilized microbial cells are primarily focused on the removal of synthetic organic chemicals (e.g. phenol, formaldehyde, methylene blue, rhodamine B dye, etc.) and hydrocarbons (Table 2). However, despite its intrinsic interest, there is very little research on their use on the removal of heavy metals (Table 2), which are among the most hazardous pollutants released by anthropogenic activities today. Today, the use of microorganisms for heavy metal decontamination is one of the most promising green alternatives under investigation. In fact, many microorganisms including Bacillus megaterium Y-4106 and Weissella oryzae107 have been reported to efficiently remove heavy metals transforming them into palladium and silver NPs of interest, respectively. Therefore, this area is still an “in development” scientific discipline and it is expected that more investigations focused on heavy metal bioremediation using hydrogels will be conducted in the near future. Review of the literature also reveals that bacteria (Bacillus, Pseudomonas, or Rhodococcus, etc.) are the most commonly immobilized microorganisms in hydrogels. This is due to their high diversity, metabolic versatility, adaptability, and rapid growth in optimal conditions in comparison to other microbial cells.108 For example, Zvulunov et al., (2019)2 and Zvulunov and Radian (2021)109 used the synthetic polymer polyethylenimine (PEI) mixed with montmorillonite clay to produce hydrogels in which Pseudomonas putida cells were adsorbed. They tested its potential to remove formaldehyde from solution and compared the results obtained between using free cells and immobilized cells. They observed that the hydrogel/clay system acted as a pH buffer and only when immobilized bacteria were used, more than 99% of formaldehyde degradation was attained. Biofilm-forming bacteria should also be further researched for their inclusion in biohydrogels, as they could improve their mechanical properties. Extracellular polymeric substances (EPSs) released by some microorganisms establish the functional and structural integrity of biofilms, determining their physicochemical properties. These substances confer viscoelasticity, tightness, and self-healing abilities to biofilms, which could be highly useful for improving their rheological properties.110–112 Therefore, biofilm-forming bacteria capable of eliminating heavy metals and converting them into valuable products are excellent candidates for developing bioremediation applications with hydrogels.
We have also identified a need to investigate other types of microorganisms as the majority of studies are focused on bacteria. The variety of microorganisms with bioremediation and metal recovery potential, including yeasts, filamentous fungi, algae, or archaea, offers a wide range of possibilities for exploration. For instance, filamentous fungi also possess bioremediation potential, and their immobilization within composite hydrogels has proven to be effective. A notable example is the work by Perullini et al., (2010),105 wherein they immobilized the mycelium of the fungus species Stereum hirsutum within alginate beads inside a sodium silicate monolith for the removal of malachite green. The obtained results highlight that the composite hydrogels in which the beads were encapsulated in silica, showed higher removal rates than others, achieving 80% of malachite green elimination.
Despite the enormous potential presented by both bacteria and filamentous fungi, it is also important to consider their possible drawbacks or areas for improvement. One of these drawbacks is the potential degradation of the polymer matrix due to the release of secondary metabolites by microbial cells or physical stresses occurring during their application, both in the environment (in situ experimentation) or in bioreactors (ex situ experimentation). This fact could also make the scaling-up process of these hydrogels more difficult. Therefore, we consider fundamental the study of the rheological properties of the hydrogels investigated as potential candidates for bioremediation. Unfortunately, very few studies contribute to this today.
Finally, the use of cell-free biohydrogels systems is also being studied. Most of them consist basically in both bacterial or fungal bioproducts such as enzymes, that are isolated and immobilized within the polymeric matrices. An important practical benefit that enzymes offer against whole cells is that it is not necessary to remove cell biomass debris due to the biodegradable nature of enzymes.113 Moreover, enzymatic reactions reduce the required activation energy, leading to a faster reaction compared to non-enzymatic reactions.114 All this results in a more cost-effective process with reduced waste production.
5. Other applications of composite hydrogels
5.1. Biomedical industry
Due to their biocompatibility, biodegradability, soft consistency and microstructure, polymeric hydrogels have been widely used for biomedical applications such as drug delivery or tissue engineering. The combination of these matrices with other compounds such as magnetic particles or clays makes them more versatile materials with enhanced mechanical, structural and swelling properties. For example, composite hydrogels have been widely developed as 3D inks for the creation of scaffolds useful in regenerative medicine and tissue engineering. In this scenario, Alexa et al. (2021a)115 studied the addition of organomodified montmorillonite into alginate hydrogels as 3D-printed scaffolds for bone tissue regeneration. The introduction of the clay leads to an improvement of the porosity, mechanical properties and biocompatibility of the hydrogel. In another work, Alexa et al. (2021b)9 developed 3D printed scaffolds for tissue engineering by adding the montmorillonite into methacrylated gelatin hydrogels, increasing the viscosity of the inks, the porosity and mechanical properties of the hydrogels. Similarly, gelatin methacryloyl hydrogels with synthetic nanoclay LAPONITE® were used by Man et al. (2022)116 to create 3D printed structures able to retain and release epigenetically activated osteoblast-derived extracellular vesicles for bone repair. Here, the clay binds, stabilizes, and improves the retention and release of the extracellular vesicles in the hydrogels, thus enhancing the proliferation, migration, histone acetylation and mineralisation of human bone marrow stromal cells. Composite hydrogels are also used in cosmetics formulations. For example, Cavallaro et al. (2022)117 introduced halloysite nanotubes into keratin/alginate hydrogels for protective treatment of human hair. They demonstrate that the addition of keratin and halloysite enhances the tensile strength of the human hair after the exposure to UV irradiation.
Another interesting and widely studied application for the composite hydrogels is wound dressing. In this regard, Elbassyoni et al. (2020)118 studied the effect of attapulgite clay in the properties of PVA and hydroxyethyl starch hybrid hydrogels. They observed that the clay increased the swelling ability, the mechanical characteristics and the antimicrobial activity for their use in dermal wound dressings. In a later work of the same group,119 the attapulgite was introduced into PVA–carboxymethyl cellulose hydrogels to improve their mechanical, thermal properties and antimicrobial activity. Similarly, Feiz et al. (2019)120 introduced chitosan-modified montmorillonite (CsMMT) and nitrofurazone into PVA hydrogel films for wound dressing with drug release. Thus, the drug release was controlled with the quantity of CsMMT, which also modified the gel fraction, water vapor permeability, water uptake and tensile/rheological properties of films.
As clay composites considerably change the drug release behaviour due to their effect on the swelling, diffusion and network parameters, composite hydrogels also find several advantages as drug delivery materials. For example, Nath et al. (2019)121 reported that when montmorillonite was added to gelatin (Ge)-g-poly(acrylic acid-co-acrylamide) hydrogels, the controlled release of vitamin B12 in artificial gastric fluid and artificial intestinal fluid, was enhanced due to the better swellability of the hydrogels with the addition of clay. Samanta et al. (2022)122 introduced the montmorillonite into acrylamide-polyethylene glycol hydrogels to increase the efficient cure of different diseases and reduce the side effects. Here, the swelling, drug loading, drug entrapment efficiency (86%), and drug release properties (96.6%) increased for 3.2 wt% clay and 24 wt% PEG. Moreover, montmorillonite was modified with curdlan-grafted poly-N-isopropylacrylamide by Bera et al. (2023)123 for the delivery of erlotinib HCl (ERL) to triple-negative breast cancer cells. Again, the clay reinforced the drug entrapment efficiency drug release profile, temperature/pH-responsive swelling, biodegradability and mucoadhesion properties. Not only montmorillonite but also other types of clays are widely used for drug delivery, due to their capacity of adsorption of small molecules in contrast to the high burst release and rapid diffusion from the pristine hydrogels. Thus, Khachani et al. (2022)124 studied the drug release from PEG hydrogels with LAPONITE®, montmorillonite, and halloysite. They were able not only to control the release profile using the different clay combined with the PEG, but also controlling the buffer salt concentration and pH. Finally, Luo et al. (2019)125 used the adsorption capacity of halloysite nanotubes to create chitosan hybrid hydrogels for sustained drug release.
5.2. Oil recovery
Composite hydrogels have been also used in oil reservoir applications to palliate some of the deficiencies such as the large energy waste, large inert gas consumption and low efficiency of traditional methods. These problems are mainly due to the hot work on oil pipelines and the high permeability zones of the channels where part of the oil is left behind. Different strategies using composite hydrogels had been developed to face these problems and enhance the oil recovery process. For this aim, Tessarolli et al. (2019)8 developed hybrid composite hydrogels based on acrylamide, bentonite and polyethylenimine with good stability, strength and blocking ability characteristics under harsh conditions of salinity and hardness. Polyacrylamide hydrogels with bentonite and montmorillonite were used in oil reservoirs by Amiri (2019)126 for water shut-off operations. The strong and reversible interactions between the polyacrylamide and the clays improved the thermal stability, strength, and gelation time of these hydrogels. Similarly, Roslan et al. (2023)127 developed water shut-off techniques in oil reservoirs by using composite hydrogels based on acrylamide, PEG, attapulgite and bentonite. While the addition of clays improves the swelling capacity and thermal stability of the hydrogels, an excessive concentration leads to a decrease in the swelling ratio due to the additional crosslinking points which strengthen the polymeric chain and lower the porosity. Another problem in the water shut-off operations is the thixotropy of the hydrogels when filling in the annulus between the slotted liners and the wellbore in horizontal wells. In this regard, Chen et al. (2022)128 introduced LAPONITE® into acrylamide matrices to enhance the hydrogels thixotropy performance and thus their filling shape. Finally, Fan et al. (2022)129 also demonstrated that the inclusion of montmorillonite in acrylamide-based hydrogels significantly improves their compressive strength, flame resistance and oil and gas isolation.
5.3. Agricultural applications
The great ability of composite hydrogels to absorb water by swelling processes makes them of special interest as growing scaffolds. For example, Santoso et al. (2019)7 demonstrated that the addition of bentonite (30 wt%) in cellulose hydrogels resulted in a remarkable improvement of the Congo red adsorption (45.77 mg g−1). These hydrogels were also used as substrates for soilless culture, promoting germination and growth of mung bean (Vigna radiata L.) and mouse-ear cress (Arabidopsis thaliana), thanks to the hydrated microenvironment created by the bentonite into the hydrogel matrix. Similarly, pectin–montmorillonite hydrogels were used by Fernandes Filho et al. (2023)10 (122) as dye and herbicide adsorbents, as the inclusion of montmorillonite at low concentrations (1%) increased the methylene blue and 2,4-dichlorophenoxyacetic acid removal efficiency of the hydrogels. Moreover, Aydınoğlu et al. (2021)130 fabricated carrageenan/psyllium/montmorillonite composite hydrogels as water absorbents for soil irrigation in agriculture. Another strategy for the use of hybrid composite hydrogels in agriculture, is the reduction of pesticides by controlled release formulations. In this concern, Zhang et al. (2020)131 propose the use of alginate–bentonite hydrogels for the controlled release of imidacloprid, a widely used agricultural insecticide. More recently, Dou et al. (2023)132 fabricated chitosan–montmorillonite hydrogels as controlled-release fertilizers. In this case, the addition of montmorillonite, leads to an enhancement of the phosphorus and potassium release, in the water retention of the soil with a daily release of 2.8% of the loaded fertilizer and a high degradation (57%) after 20 days.
6. Conclusions and future perspectives
The current literature reflects that the most promising research for improving the physical, chemical, and biological properties of hybrid hydrogels involves the addition of inert and natural particles of micro- or nanoscopic size (composites), such as nanoclays. The incorporation of natural nanoclays would not only improve mechanical, physical, and chemical properties, but also provide support for the fixation of microorganisms and the formation of biofilms. Indeed, clays are the natural habitat of a wide variety of organisms with proven capabilities as bioremediation agents.47,48 This represents a significant advancement in improving both the biocompatibility of hydrogels and the processes of microorganism immobilization within them. These recent developments in the field open the door to the search for new natural micro- or nano-composite materials that can stimulate the metabolic activity of indigenous microorganisms with bioremediation and biotechnological capabilities. In the present manuscript, we have emphasized the use of organic and eco-friendly polymers for the fabrication of hydrogels. This would not only be beneficial in preventing environmental pollution and ensuring biocompatibility, but also in saving costs in manufacturing and waste management processes. However, the inclusion of inorganic clay composites can also provide hydrogels with greater long-term mechanical stability since they are non-biodegradable natural minerals. Furthermore, clays are natural compounds, thus they have no impact on the environment. This increased temporal stability grants clay-based hybrid hydrogels superior properties for industrial applications.
The utilization of clay-based hydrogels for the physico-chemical remediation of environments contaminated with toxic compounds have been extensively studied in recent years (see Table 1). However, the use of these gels with immobilized microbial cells has not been explored as much in this field as summarized in Table 2. The increased use of abiotic clay-based hydrogels may be due to the fact that clays are capable of directly absorbing metals, eliminating the need for the addition of microorganisms. This can be the case for heavy metals and other contaminants that can be absorbed through electrostatic interactions, such as cadmium or zinc. However, for other elements that can be removed through processes like biomineralization (e.g. Pd) or redox biotransformation (e.g. Se), the addition of microorganisms would greatly enhance the bioremediation efficiency of the hydrogels. Therefore, the effectiveness of biotic or abiotic clay-based hydrogels for bioremediation can vary depending on the specific metal to be removed.
Compared to conventional abiotic physico-chemical remediation strategies, bioremediation is often cheaper, eco-friendly, and more effective towards several contaminants.133 In many cases, bioremediation of contaminants by hydrogels is not only associated with pollutant removal, but also with the recovery of critical materials and value-added products, which makes them a promising and efficient strategy in line with the principles of the circular economy. This is due to the potential of microorganisms not only for the adsorption and immobilization of the pollutants, but also for its ability to enzymatically transform them into less toxic nanoparticles of great interest in both medicine and industry. For all these reasons, major efforts must be made in designing efficient biohydrogels over physico-chemical ones for environmental remediation purposes. Interestingly, in the present review, we have identified bacteria as the most studied microorganisms for their immobilization in clay-based hydrogels. This is mainly due to their high diversity, metabolic versatility, adaptability, and rapid growth in comparison to other microbial cells. In addition, recent studies conducted in our research group revealed that biofilm-forming bacteria are capable of enhancing the rheological properties of hybrid hydrogels composed of alginate, cellulose, and bentonite clays (data not shown). This is most probably due to the wide variety of unique properties of extracellular polymeric substances (EPSs) forming the biofilms. Bacterial biofilms have a slimy consistency and can be described as viscoelastic, which are materials exhibiting both fluid-like and solid-like characteristics.112 Depending on the bacterial species, biofilms have also shown very high stiffness ability, resisting high pressure values ranging from hundreds to several thousand kPa.111 Their self-healing abilities have been proved as well. When exposed to shear forces, biofilms can quickly and fully recover their initial viscoelastic properties.110 Considering all the points discussed above, the combined use of clays and biofilm-forming microorganisms in the preparation of hydrogels emerges as an ideal system for three main reasons: (a) both can enhance the mechanical properties of the gels, (b) clays can facilitate biofilm formation by acting as a support, and (c) microorganisms can improve the efficiency of bioremediation and biorecovery processes.
Other less explored microorganisms with bioremediation potential, such as fungi, have been reported to be effective in pollutant removal within silica composite hydrogels.105 The filamentous structure of some fungal species, forming extensive hyphal networks, could allow them to explore larger areas and reach nutrients and contaminants within the polymeric matrix that might be less accessible to bacteria. This could greatly enhance the efficiency of redox biotransformation, adsorption, or biomineralization microbial processes. Therefore, we consider that further research is necessary on hydrogels with immobilized filamentous fungi for the bioremediation of contaminated environments.
As discussed before, biohydrogels could also be used for the biorecovery of precious metals and valuable products derived from the interaction between the contaminant and the microorganism. Inclusion of magnetic materials such as magnetite (Fe3O4) NPs within hydrogels is one of the latest innovations being researched in the field of biorecovery. Magnetite NPs produced by magnetic bacteria are being investigated as suitable for bioscience and biomedicine due to their water solubility and biocompatibility.134 It is well known that magnetic NP surfaces can be functionalized for different purposes. The nature of the coating can modulate the interactions between the NPs and the polymeric filaments of hydrogels, having a direct impact on their properties, as we have demonstrated in various studies.4 Additionally, magnetic NPs with the appropriate surface chemistry can conjugate drugs, proteins, enzymes, antibodies, or nucleotides for use in numerous applications. The most significant advantage of magnetic hydrogels is their easy recovery and separation simply by applying a magnetic field.135 However, magnetic hydrogels with encapsulated microorganisms have not been explored for bioremediation and biorecovery yet. In fact, most works found in the literature are focused on their application in biomedicine for targeted drug delivery and for physico-chemical remediation. Therefore, the study of magnetic hydrogels for the bioremediation and biorecovery of contaminated environments emerges as an interesting field of study to explore.
Author contributions
Miguel A. Ruiz-Fresneda: conceptualization, writing – original draft, writing – review & editing, visualization. Eduardo González-Morales: conceptualization, writing – original draft, writing – review & editing, visualization. Cristina Gila-Vilchez: conceptualization, writing – original draft, writing – review & editing, visualization. Alberto Leon-Cecilla: writing – review & editing, visualization. Mohamed L. Merroun: conceptualization, writing – original draft, writing – review & editing, resources, supervision, project administration, funding acquisition, visualization. Antonio L. Medina-Castillo: conceptualization, writing – original draft, writing – review & editing, resources, supervision, visualization. Modesto T. Lopez-Lopez: conceptualization, writing – original draft, writing – review & editing, resources, supervision, project administration, funding acquisition, visualization.
Data availability
No primary research results, software or code have been included and no new data were generated or analysed as part of this review.
Conflicts of interest
There are no conflicts to declare.
Acknowledgements
This study was supported by grant TED2021-131099B-I00 funded by MCIN/AEI/10.13039/501100011033, Spain, and by the European Union NextGenerationEU/PRTR, awarded to M. L. M. and M. T. L.-L., A. L.-C. acknowledges grant FPU19/01801 funded by MCIN/AEI/10.13039/501100011033 and “ESF Investing in your future”, Spain.
References
- T. Mehrotra, S. Dev, A. Banerjee, A. Chatterjee, R. Singh and S. Aggarwal, Use of immobilized bacteria for environmental bioremediation: A review, J. Environ. Chem. Eng., 2021, 9(5), 105920 CrossRef CAS.
- Y. Zvulunov, Z. Ben-Barak-Zelas, A. Fishman and A. Radian, A self-regenerating clay-polymer-bacteria composite for formaldehyde removal from water, Chem. Eng. J., 2019, 374, 1275–1285 CrossRef CAS.
- J. I. Dawson and R. O. C. Oreffo, Clay: new opportunities for tissue regeneration and biomaterial design, Adv. Mater., 2013, 25(30), 4069–4086 CrossRef CAS PubMed.
- A. B. Bonhome-Espinosa, F. Campos, I. A. Rodriguez, V. Carriel, J. A. Marins and A. Zubarev,
et al., Effect of particle concentration on the microstructural and macromechanical properties of biocompatible magnetic hydrogels, Soft Matter, 2017, 13(16), 2928–2941 RSC.
- Y. H. Kim, X. Yang, L. Shi, S. A. Lanham, J. Hilborn and R. O. C. Oreffo,
et al., Bisphosphonate nanoclay edge-site interactions facilitate hydrogel self-assembly and sustained growth factor localization, Nat. Commun., 2020, 11(1), 1365 CrossRef CAS PubMed.
- J. P. Chen and Y. S. Lin, Decolorization of azo dye by immobilized Pseudomonas luteola entrapped in alginate–silicate sol–gel beads, Process Biochem., 2007, 42(6), 934–942 CrossRef CAS.
- S. P. Santoso, A. Kurniawan, F. E. Soetaredjo, K. C. Cheng, J. N. Putro and S. Ismadji,
et al., Eco-friendly cellulose–bentonite porous composite hydrogels for adsorptive removal of azo dye and soilless culture, Cellulose, 2019, 26(5), 3339–3358 CrossRef CAS.
- F. G. C. Tessarolli, S. T. S. Souza, A. S. Gomes and C. R. E. Mansur, Influence of polymer structure on the gelation kinetics and gel strength of acrylamide-based copolymers, bentonite and polyethylenimine systems for conformance control of oil reservoirs, J. Appl. Polym. Sci., 2019, 136(22), 47556 CrossRef.
- R. L. Alexa, H. Iovu, B. Trica, C. Zaharia, A. Serafim and E. Alexandrescu,
et al., Assessment of Naturally Sourced Mineral Clays for the 3D Printing of Biopolymer-Based Nanocomposite Inks, Nanomaterials, 2021, 11(3), 703 CrossRef CAS PubMed.
- W. B. F. Filho, S. T. R. Agassin, K. P. Naidek and A. T. Paulino, Pectin hydrogels modified with montmorillonite: Case studies for the removal of dyes and herbicides from water, J. Environ. Chem. Eng., 2023, 11(5), 110846 CrossRef CAS.
- H. Zhang, A. M. Omer, Z. Hu, L. Y. Yang, C. Ji and X. K. Ouyang., Fabrication of magnetic bentonite/carboxymethyl chitosan/sodium alginate hydrogel beads for Cu(II) adsorption, Int. J. Biol. Macromol., 2019, 135, 490–500 CrossRef CAS PubMed.
- T. Salehi, M. Shirvani, M. Dinari and E. Gavili, Adsorptive Removal of Lead from Water Using a Novel Cysteine-Bentonite/Poly(vinyl alcohol)/Alginate Nanocomposite, J. Polym. Environ., 2022, 30(10), 4463–4478 CrossRef CAS.
- C. Wu, Q. Ma, W. Ge, S. Yan, L. Xia and S. Song, Preparation of Fe-La montmorillonite nanosheets based composite hydrogel beads for As(V) removal from water, Chem. Phys. Lett., 2022, 806, 139994 CrossRef CAS.
-
P. K. Gautam, R. Gautam, S. Banerjee, M. Chattopadhyaya and J. Pandey, Heavy metals in the environment: Fate, transport, toxicity and remediation technologies, in Heavy Metals: Sources, Toxicity and Remediation Techniques, 2016, pp. 101–130 Search PubMed.
- D. M. Voica, L. Bartha, H. L. Banciu and A. Oren, Heavy metal resistance in halophilic Bacteria and Archaea, FEMS Microbiol. Lett., 2016, 363(14), fnw146 CrossRef PubMed.
- A. V. Tugarova, P. V. Mamchenkova, Y. A. Dyatlova and A. A. Kamnev, FTIR and Raman spectroscopic studies of selenium nanoparticles synthesised by the bacterium Azospirillum thiophilum, Spectrochim. Acta, Part A, 2018, 192, 458–463 CrossRef CAS PubMed.
- Y. Zhu, X. Kong, E. Wu, N. Zhu, D. Liang and M. Lou,
et al., Biosynthesis of spherical selenium nanoparticles with halophilic Bacillus subtilis subspecies stercoris strain XP for inhibition of strawberry pathogens, Shengwu Gongcheng Xuebao, 2021, 37(8), 2825–2835 CAS.
- M. A. Ruiz-Fresneda, S. Schaefer, R. Hübner, K. Fahmy and M. L. Merroun, Exploring Antibacterial Activity and Bacterial-Mediated Allotropic Transition of Differentially Coated Selenium Nanoparticles, ACS Appl. Mater. Interfaces, 2023, 15(25), 29958–29970 CrossRef CAS PubMed.
- S. C. Martins, C. M. Martins, L. Maria and S. T. Santaella, Immobilization of microbial cells: A promising tool for treatment of toxic pollutants in industrial wastewater, Afr. J. Biotechnol., 2013, 12(28), 4412–4418 CrossRef CAS.
- N. Mallick, Biotechnological potential of immobilized algae for wastewater N, P and metal removal: A review, Biometals, 2022, 15, 377–390 CrossRef PubMed.
- S. A. Covarrubias, L. E. de-Bashan, M. Moreno and Y. Bashan, Alginate beads provide a beneficial physical barrier against native microorganisms in wastewater treated with immobilized bacteria and microalgae, Appl. Microbiol. Biotechnol., 2012, 93(6), 2669–2680 CrossRef CAS PubMed.
- S. Girijan and M. Kumar, Immobilized biomass systems: an approach for trace organics removal from wastewater and environmental remediation, Curr. Opin. Environ. Sci. Health, 2019, 12, 18–29 CrossRef.
-
Interpenetrating Polymer Networks, ed. D. Klempner, L. H. Sperling and L. A. Utracki, Advances in Chemistry, American Chemical Society, Washington, DC, 1994, vol. 239, https://pubs.acs.org/doi/book/10.1021/ba-1994-0239 Search PubMed.
- L. H. Sperling and V. Mishra, The Current Status of Interpenetrating Polymer Networks, Polym. Adv. Technol., 1996, 7(4), 197–208 CrossRef CAS.
- Z. Li, Y. Zhou, T. Li, J. Zhang and H. Tian, Stimuli-responsive hydrogels: Fabrication and biomedical applications, View, 2022, 3(2), 20200112 CrossRef CAS.
- Z. Yuan, J. Ding, Y. Zhang, B. Huang, Z. Song and X. Meng,
et al., Components, mechanisms and applications of stimuli-responsive polymer gels, Eur. Polym. J., 2022, 177, 111473 CrossRef CAS.
- Z. U. Arif, M. Y. Khalid, A. Tariq, M. Hossain and R. Umer, 3D printing of stimuli-responsive hydrogel materials: Literature review and emerging applications, Giant, 2024, 17, 100209 CrossRef CAS.
- S. Fuchs, K. Shariati and M. Ma, Specialty Tough Hydrogels and Their Biomedical Applications, Adv. Healthcare Mater., 2020, 9(2), 1901396 CrossRef CAS PubMed.
- P. Ilg, Stimuli-responsive hydrogels cross-linked by magnetic nanoparticles, Soft Matter, 2013, 9(13), 3465–3468 RSC.
- L. Mei, J. Cong, S. Li, T. Ren, Y. Bai and Q. Liu,
et al., Polyacrylamide-Chitosan based magnetic hydrogels with high stiffness and ultra-toughness, Composites, Part A, 2023, 168, 107478 CrossRef CAS.
- Z. Liu, J. Liu, X. Cui, X. Wang, L. Zhang and P. Tang, Recent Advances on Magnetic Sensitive Hydrogels in Tissue Engineering, Front. Chem., 2020, 8, 124 CrossRef CAS PubMed.
- M. Barczak, P. Borowski, C. Gila-Vilchez, M. Alaminos, F. González-Caballero and M. T. López-López, Revealing importance of particles’ surface functionalization on the properties of magnetic alginate hydrogels, Carbohydr. Polym., 2020, 247, 116747 CrossRef CAS PubMed.
- T. Yan, D. Rao, Y. Chen, Y. Wang, Q. Zhang and S. Wu, Magnetic nanocomposite hydrogel with tunable stiffness for probing cellular responses to matrix stiffening, Acta Biomater., 2022, 138, 112–123 CrossRef CAS PubMed.
- M. Zhang, J. Yu, K. Shen, R. Wang, J. Du and X. Zhao,
et al., Highly Stretchable Nanocomposite Hydrogels with Outstanding Antifatigue Fracture Based on Robust Noncovalent Interactions for Wound Healing, Chem. Mater., 2021, 33(16), 6453–6463 CrossRef CAS.
- C. Chen, Z. Li, C. Xu, M. Kang, C. Lee and T. Aghaloo,
et al., Self-Assembled Nanocomposite Hydrogels as Carriers for Demineralized Bone Matrix Particles and Enhanced Bone Repair. Adv Healthcare, Materials, 2024, 2303592 CAS.
- X. Hu, G. Nian, X. Liang, L. Wu, T. Yin and H. Lu,
et al., Adhesive Tough Magnetic Hydrogels with High Fe3O4 Content, ACS Appl. Mater. Interfaces, 2019, 11(10), 10292–10300 CrossRef CAS PubMed.
- C. Gila-Vilchez, M. C. Mañas-Torres, R. Contreras-Montoya, M. Alaminos, J. D. G. Duran and L. A. De Cienfuegos,
et al., Anisotropic magnetic hydrogels: design, structure and mechanical properties, Philos. Trans. R. Soc., A, 2019, 377(2143), 20180217 CrossRef CAS PubMed.
- Y. Shi, Y. Li and T. Coradin, Magnetically-oriented type I collagen-SiO2@Fe3O4 rods composite hydrogels tuning skin cell growth, Colloids Surf., B, 2020, 185, 110597 CrossRef CAS PubMed.
- A. Sharma, M. D. DiVito, D. E. Shore, A. D. Block, K. Pollock and P. Solheid,
et al., Alignment of collagen matrices using magnetic nanowires and magnetic barcode readout using first order reversal curves (FORC) (invited), J. Magn. Magn. Mater., 2018, 459, 176–181 CrossRef CAS.
- E. García-Romero, E. María Manchado, M. Suárez and J. García-Rivas, Spanish Bentonites: A Review and New Data on Their Geology, Mineralogy, and Crystal Chemistry, Minerals, 2019, 9(11), 696 CrossRef.
-
C. C. Harvey and G. Lagaly, Industrial Applications, In Handbook of Clay Science, ed. F. Bergaya and G. Lagaly, Developments in Clay Science, Elsevier, 2013, ch. 4.2, vol. 5,pp. 451–490. Available from: https://www.sciencedirect.com/science/article/pii/B9780080982595000184 Search PubMed.
- M. Lopez-Fernandez, R. Vilchez-Vargas, F. Jroundi, N. Boon, D. Pieper and M. L. Merroun, Microbial community changes induced by uranyl nitrate in bentonite clay microcosms, Appl. Clay Sci., 2018, 160, 206–216 CrossRef CAS.
- K. A. Gilmour, C. T. Davie and N. Gray, An indigenous iron-reducing microbial community from MX80 bentonite - A study in the framework of nuclear waste disposal, Appl. Clay Sci., 2021, 205, 106039 CrossRef CAS.
- K. Mijnendonckx, N. Bleyen, A. Van Gompel, I. Coninx and N. Leys, pH and microbial community determine the denitrifying activity in the presence of nitrate-containing radioactive waste, Front. Microbiol., 2022, 13 Search PubMed.
- R. Shrestha, K. Cerna, R. Spanek, D. Bartak, T. Cernousek and A. Sevcu, The effect of low-pH concrete on microbial community development in bentonite suspensions as a model for microbial activity prediction in future nuclear waste repository, Sci. Total Environ., 2022, 808, 151861 CrossRef CAS PubMed.
- M. A. Ruiz-Fresneda, M. Lopez-Fernandez, M. F. Martinez-Moreno, A. Cherkouk, Y. Ju-Nam and J. J. Ojeda,
et al., Molecular Binding of Eu III/CmIII by Stenotrophomonas bentonitica and Its Impact on the Safety of Future Geodisposal of Radioactive Waste, Environ. Sci. Technol., 2020, 54(23), 15180–15190 CrossRef CAS PubMed.
- M. A. Ruiz-Fresneda, M. Morales-Hidalgo, C. Povedano-Priego, F. Jroundi, J. Hidalgo-Iruela and M. Cano-Cano,
et al., Unlocking the key role of bentonite fungal isolates in tellurium and selenium bioremediation and biorecovery: Implications in the safety of radioactive waste disposal, Sci. Total Environ., 2024, 912, 169242 CrossRef CAS PubMed.
- M. A. Ruiz-Fresneda, M. V. Fernández-Cantos, J. Gómez-Bolívar, A. S. Eswayah, P. H. E. Gardiner and M. Pinel-Cabello,
et al., Combined bioreduction and volatilization of SeVI by Stenotrophomonas bentonitica: Formation of trigonal selenium nanorods and methylated species, Sci. Total Environ., 2023, 858, 160030 CrossRef CAS PubMed.
- K. K. Kadimpati, K. P. Mondithoka, S. Bheemaraju and V. R. M. Challa, Entrapment of marine microalga, Isochrysis galbana, for biosorption of Cr(III) from aqueous solution: isotherms and spectroscopic characterization, Appl. Water Sci., 2013, 3(1), 85–92 CrossRef CAS.
- M. López-Fernández, O. Fernández-Sanfrancisco, A. Moreno-García, I. Martín-Sánchez, I. Sánchez-Castro and M. L. Merroun, Microbial communities in bentonite formations and their interactions with uranium, Appl. Geochem., 2014, 49, 77–86 CrossRef.
-
J. A. Trelles and C. W. Rivero, Whole Cell Entrapment Techniques, in Immobilization of Enzymes and Cells, Methods in Molecular Biology, Humana Press, Totowa, NJ, 3rd edn, 2013, vol. 1051 Search PubMed.
- J. Żur, D. Wojcieszyńska and U. Guzik, Metabolic Responses of Bacterial Cells to Immobilization, Molecules, 2016, 21(7), 958 CrossRef PubMed.
- M. J. Lapponi, M. B. Méndez, J. A. Trelles and C. W. Rivero, Cell immobilization strategies for biotransformations. Current Opinion in Green and Sustainable, Chemistry, 2022, 33, 100565 CAS.
- J. Lu, W. Peng, Y. Lv, Y. Jiang, B. Xu and W. Zhang,
et al., Application of Cell Immobilization Technology in Microbial Cocultivation Systems for Biochemicals Production, Ind. Eng. Chem. Res., 2020, 59(39), 17026–17034 CrossRef CAS.
- E. C. Giese, D. D. V. Silva, A. F. M. Costa, S. G. C. Almeida and K. J. Dussán, Immobilized microbial nanoparticles for biosorption, Crit. Rev. Biotechnol., 2020, 40(5), 653–666 CrossRef CAS PubMed.
- M. E. Mahmoud, A. E. H. Abdou, S. M. S. Mohamed and M. M. Osman, Engineered staphylococcus aureus via immobilization on magnetic Fe3O4-phthalate nanoparticles for biosorption of divalent ions from aqueous solutions, J. Environ. Chem. Eng., 2016, 4(4), 3810–3824 CrossRef CAS.
- S. H. Song, S. S. Choi, K. Park and Y. J. Yoo, Novel hybrid immobilization of microorganisms and its applications to biological denitrification, Enzyme Microb. Technol., 2005, 37(6), 567–573 CrossRef CAS.
- P. Kilonzo and M. Bergougnou, Surface Modifications for Controlled and Optimized Cell Immobilization by Adsorption: Applications in Fibrous Bed Bioreactors Containing Recombinant Cells, J. Microb. Biochem. Technol., 2012, S8, 001 Search PubMed.
- Y. H. Hsueh, W. C. Liaw, J. M. Kuo, C. S. Deng and C. H. Wu, Hydrogel Film-Immobilized Lactobacillus brevis RK03 for γ-Aminobutyric Acid Production, Int. J. Mater. Sci., 2017, 18(11), 2324 Search PubMed.
- G. Kumar, P. Sivagurunathan, J. H. Park and S. H. Kim, Improved Hydrogen Production from Galactose Via Immobilized Mixed Consortia, Arabian J. Sci. Eng., 2015, 40(8), 2117–2122 CrossRef CAS.
- M. Christwardana, Combination of physico-chemical entrapment and crosslinking of low activity laccase-based biocathode on carboxylated carbon nanotube for increasing biofuel cell performance, Enzyme Microb. Technol., 2017, 106, 1–10 CrossRef CAS PubMed.
- M. C. P. Gonçalves, S. A. V. Morales, E. S. Silva, A. E. Maiorano, R. F. Perna and T. G. Kieckbusch, Entrapment of glutaraldehyde-crosslinked cells from Aspergillus oryzae IPT-301 in calcium alginate for high transfructosylation activity, J. Chem. Technol. Biotechnol., 2020, 95(9), 2473–2482 CrossRef.
- W. X. Waresindo, H. R. Luthfianti, A. Priyanto, D. A. Hapidin, D. Edikresnha and A. H. Aimon,
et al., Freeze–thaw hydrogel fabrication method: basic principles, synthesis parameters, properties, and biomedical applications, Mater. Res. Express, 2023, 10(2), 024003 CrossRef.
- V. Krasňan, R. Stloukal, M. Rosenberg and M. Rebroš, Immobilization of cells and enzymes to LentiKats®, Appl. Microbiol. Biotechnol., 2016, 100(6), 2535–2553 CrossRef PubMed.
- A. Durieux, X. Nicolay and J. P. Simon, Continuous malolactic fermentation by Oenococcus oeni entrapped in LentiKats, Biotechnol. Lett., 2000, 22(21), 1679–1684 CrossRef CAS.
- A. Boušková, J. Mrákota, R. Stloukal, J. Trögl, V. Pilařová and L. Křiklavová,
et al., Three examples of nitrogen removal from industrial wastewater using Lentikats
Biotechnology, Desalination, 2011, 280(1), 191–196 CrossRef.
- Y. Zhao, A. Hussain, Y. Liu, Z. Yang, T. Zhao and B. Bamanu,
et al., Electrospinning micro-nanofibers immobilized aerobic denitrifying bacteria for efficient nitrogen removal in wastewater, J. Environ. Manage., 2023, 343, 118230 CrossRef CAS PubMed.
- P. Wang, H. Lv, X. Cao, Y. Liu and D. G. Yu, Recent Progress of the Preparation and Application of Electrospun Porous Nanofibers, Polymers, 2023, 15(4), 921 CrossRef CAS PubMed.
- S. A. Khan and T. A. Khan, Clay-hydrogel nanocomposites for adsorptive amputation of environmental contaminants from aqueous phase: A review, J. Environ. Chem. Eng., 2021, 9(4), 105575 CrossRef CAS.
- S. Pandey, N. Son and M. Kang, Synergistic sorption performance of karaya gum crosslink poly(acrylamide-co-acrylonitrile) @ metal nanoparticle for organic pollutants, Int. J. Biol. Macromol., 2022, 210, 300–314 CrossRef CAS PubMed.
- J. Ma, Z. Jiang, J. Cao and F. Yu, Enhanced adsorption for the removal of antibiotics by carbon nanotubes/graphene oxide/sodium alginate triple-network nanocomposite hydrogels in aqueous solutions, Chemosphere, 2020, 242, 125188 CrossRef CAS PubMed.
- C. B. Godiya, X. Cheng, D. Li, Z. Chen and X. Lu, Carboxymethyl cellulose/polyacrylamide composite hydrogel for cascaded treatment/reuse of heavy metal ions in wastewater, J. Hazard. Mater., 2019, 364, 28–38 CrossRef CAS PubMed.
- S. Hossain, M. Shahruzzaman, S. F. Kabir, M. S. Rahman, S. Sultana and A. K. Mallik,
et al., Jute cellulose nanocrystal/poly(N,N-dimethylacrylamide-co-3-methacryloxypropyltrimethoxysilane) hybrid hydrogels for removing methylene blue dye from aqueous solution, J. Sci.: Adv. Mater. Devices, 2021, 6(2), 254–263 CAS.
- S. S. Vedula and G. D. Yadav, Superior efficacy of biocomposite membranes of chitosan with montmorillonite and kaolin vs pure chitosan for removal of Cu(II) from wastewater, J. Chem. Sci., 2022, 134(2), 55 CrossRef CAS.
- E. Matei, M. Rapa, C. I. Covaliu, A. M. Predescu, A. Turcanu and C. Predescu,
et al., Sodium Alginate-Cellulose-Nano-Clay Composite Adsorbent Applied for Lead Removal from Wastewater, Rev. Chim., 2020, 71(3), 416–424 CrossRef CAS.
- E. A. Allam, R. M. El-Sharkawy, M. A. Gizawy and M. E. Mahmoud, Assembly of CeO2–MoO3–SiO2(CH2)3-(Alginate)2 As A novel nanocomposite for removal of MnII/CrVI and 56Mn/51Cr radionuclides from water, Mater. Chem. Phys., 2021, 262, 124278 CrossRef CAS.
- S. P. Santoso, A. Kurniawan, A. E. Angkawijaya, H. Shuwanto, I. D. A. A. Warmadewanthi and C. W. Hsieh,
et al., Removal of heavy metals from water by macro-mesoporous calcium alginate–exfoliated clay composite sponges, Chem. Eng. J., 2023, 452, 139261 CrossRef CAS.
- C. Bai, L. Wang and Z. Zhu, Adsorption of Cr(III) and Pb(II) by graphene oxide/alginate hydrogel membrane: Characterization, adsorption kinetics, isotherm and thermodynamics studies, Int. J. Biol. Macromol., 2020, 147, 898–910 CrossRef CAS PubMed.
- E. Baigorria and L. F. Fraceto, Novel nanostructured materials based on polymer/organic-clay composite networks for the removal of carbendazim from waters, J. Cleaner Prod., 2022, 331, 129867 CrossRef CAS.
- S. Biswas, T. U. Rashid, T. Debnath, P. Haque and M. M. Rahman, Application of Chitosan-Clay Biocomposite Beads for Removal of Heavy Metal and Dye from Industrial Effluent, J. Compos. Sci., 2020, 4(1), 16 CrossRef CAS.
- M. M. Barbooti, B. D. Al-Dabbagh and R. H. Hilal, Preparation, characterization and utilization of polyacrylic acid–kaolin composite in the removal of heavy metals from water, Int. J. Environ. Sci. Technol., 2019, 16(8), 4571–4582 CrossRef CAS.
- P. Kumararaja, K. M. Manjaiah, S. C. Datta, T. P. Ahammed Shabeer and B. Sarkar, Chitosan-g-poly(acrylic acid)-bentonite composite: a potential immobilizing agent of heavy metals in soil, Cellulose, 2018, 25(7), 3985–3999 CrossRef CAS.
- X. Yang, T. Zhou, B. Ren, A. Hursthouse and Y. Zhang, Removal of Mn (II) by Sodium Alginate/Graphene Oxide Composite Double-Network Hydrogel Beads from Aqueous Solutions, Sci. Rep., 2018, 8(1), 10717 CrossRef PubMed.
- Q. Wang, Y. Wang and L. Chen, A green composite hydrogel based on cellulose and clay as efficient absorbent of colored organic effluent, Carbohydr. Polym., 2019, 210, 314–321 CrossRef CAS PubMed.
- L. M. Sanchez, R. P. Ollier and V. A. Alvarez, Sorption behavior of polyvinyl alcohol/bentonite hydrogels for dyes removal, J. Polym. Res., 2019, 26(6), 142 CrossRef.
- K. D. Nguyen, T. T. C. Trang and T. Kobayashi, Chitin-halloysite nanoclay hydrogel composite adsorbent to aqueous heavy metal ions, J. Appl. Polym. Sci., 2019, 136(11), 47207 CrossRef.
- E. Tekay, D. Aydınoğlu and S. Şen, Effective Adsorption of Cr(VI) by High Strength Chitosan/Montmorillonite Composite Hydrogels Involving Spirulina Biomass/Microalgae, J. Polym. Environ., 2019, 27(8), 1828–1842 CrossRef CAS.
- D. Tong, K. Fang, H. Yang, J. Wang, C. Zhou and W. Yu, Efficient removal of copper ions using a hydrogel bead triggered by the cationic hectorite clay and anionic sodium alginate, Environ. Sci. Pollut. Res., 2019, 26(16), 16482–16492 CrossRef CAS PubMed.
- H. Zhang, C. S. Hodges, P. K. Mishra, J. Y. Yoon, T. N. Hunter and J. W. Lee,
et al., Bio-Inspired Preparation of Clay–Hexacyanoferrate Composite Hydrogels as Super Adsorbents for Cs+, ACS Appl. Mater. Interfaces, 2020, 12(29), 33173–33185 CrossRef CAS PubMed.
- T. T. Nguyen, B. N. Hoang, T. V. Tran, D. V. Nguyen, T. D. Nguyen and D. V. N. Vo, Agar/maltodextrin/poly(vinyl alcohol) walled montmorillonite composites for removal of methylene blue from aqueous solutions, Surf. Interfaces, 2021, 26, 101410 CrossRef CAS.
- A. Büyükbektaş, A. Delibaş, A. Benk and R. Coşkun, LAPONITE®-AMPS/AA composite hydrogels for efficient removal of methylene blue (MB), J. Polym. Res., 2021, 28(8), 307 CrossRef.
- R. Liu, S. Wen, Y. Sun, B. Yan, J. Wang and L. Chen,
et al., A nanoclay enhanced Amidoxime-Functionalized Double-Network hydrogel for fast and massive uranium recovery from seawater, Chem. Eng. J., 2021, 422, 130060 CrossRef CAS.
- L. Shi, W. Liu, X. Zhang and J. Hu, Preparation of Lignin Sulfonate Based/Organo-Montmorillonite Composite Hydrogel for Adsorbing Methylene Blue from Aqueous Solution, J. Polym. Environ., 2022, 30(10), 4287–4303 CrossRef CAS.
- Z. Wang, T. Li, H. Peng, H. Ren, J. Lin and C. Lou, Natural-clay-reinforced hydrogel adsorbent: Rapid adsorption of heavy-metal ions and dyes from textile wastewater, Water Environ. Res., 2022, 94(4), e10698 CrossRef CAS PubMed.
- J. Kurczewska, Chitosan-montmorillonite hydrogel beads for effective dye adsorption, J. Water Process Eng., 2022, 48, 102928 CrossRef.
- L. Shi, W. Liu, X. Zhang and J. Hu, Adsorption of methylene blue from aqueous solution by crosslinked carboxymethyl cellulose/organo-montmorillonite composite hydrogels, J. Polym. Res., 2023, 30(8), 305 CrossRef CAS.
- M. El-Sayed Abdel-Raouf, R. S. Kamal, D. E. Hegazy and A. Sayed, “Gamma Irradiation Synthesis of Carboxymethyl Chitosan-Nanoclay Hydrogel for the Removal of Cr(VI) and Pb(II) from Aqueous Media”, J. Inorg. Organomet. Polym., 2023, 33(4), 895–913 CrossRef CAS.
- C. Lin, L. Gan, Z. Chen, M. Megharaj and R. Naidu, Biodegradation of naphthalene using a functional biomaterial based on immobilized Bacillus fusiformis (BFN), Biochem. Eng. J., 2014, 90, 1–7 CrossRef CAS.
- B. Ruan, P. Wu, M. Chen, X. Lai, L. Chen and L. Yu,
et al., Immobilization of Sphingomonas sp. GY2B in polyvinyl alcohol–alginate–kaolin beads for efficient degradation of phenol against unfavorable environmental factors, Ecotoxicol. Environ. Saf., 2018, 162, 103–111 CrossRef CAS PubMed.
- E. Farid, E. A. Kamoun, T. H. Taha, A. El-Dissouky and T. E. Khalil, Eco-friendly Biodegradation of Hydrocarbons Compounds from Crude Oily Wastewater Using PVA/Alginate/Clay Composite Hydrogels, J. Polym. Environ., 2024, 32(1), 225–245 CrossRef CAS.
- S. Sharma, A. Hasan, N. Kumar and L. M. Pandey, Removal of methylene blue dye from aqueous solution using immobilized Agrobacterium fabrum biomass along with iron oxide nanoparticles as biosorbent, Environ. Sci. Pollut. Res., 2018, 25(22), 21605–21615 CrossRef CAS PubMed.
- C. Knierim, M. Enzeroth, P. Kaiser, C. Dams, D. Nette and A. Seubert,
et al., Living Composites of Bacteria and Polymers as Biomimetic Films for Metal Sequestration and Bioremediation: Living Composites of Bacteria and Polymers as Biomimetic Films, Macromol. Biosci., 2015, 15(8), 1052–1059 CrossRef CAS PubMed.
- G. J. Joshiba, P. S. Kumar, M. Govarthanan, P. T. Ngueagni, A. Abilarasu and C. F. Carolin, Investigation of magnetic silica nanocomposite immobilized Pseudomonas fluorescens as a biosorbent for the effective sequestration of Rhodamine B from aqueous systems, Environ. Pollut., 2021, 269, 116173 CrossRef CAS PubMed.
- A. S. Purnomo, S. R. Putra, H. S. Putro, A. Hamzah, N. A. Rohma and A. A. Rohmah,
et al., The application of biosurfactant-producing bacteria immobilized in PVA/SA/bentonite bio-composite for hydrocarbon-contaminated soil bioremediation, RSC Adv., 2023, 13(31), 21163–21170 RSC.
- M. Perullini, M. Jobbágy, N. Mouso, F. Forchiassin and S. A. Bilmes, Silica-alginate-fungi biocomposites for remediation of polluted water, J. Mater. Chem., 2010, 20(31), 6479 RSC.
- Y. Chen, K. Hu and Y. Chen, The effect of biotic and abiotic environmental factors on Pd(II) adsorption and reduction by Bacillus megaterium Y-4, Chemosphere, 2019, 220, 1058–1066 CrossRef CAS PubMed.
- P. Singh, Y. J. Kim, C. Wang, R. Mathiyalagan and D. C. Yang,
Weissella oryzae DC6-facilitated green synthesis of silver nanoparticles and their antimicrobial potential, Artif. Cells, Nanomed., Biotechnol., 2016, 44(6), 1569–1575 CrossRef CAS PubMed.
- H. Harms, D. Schlosser and L. Y. Wick, Untapped potential: exploiting fungi in bioremediation of hazardous chemicals, Nat. Rev. Microbiol., 2011, 9(3), 177–192 CrossRef CAS PubMed.
- Y. Zvulunov and A. Radian, Alginate Composites Reinforced with Polyelectrolytes and Clay for Improved Adsorption and Bioremediation of Formaldehyde from Water. ACS EST, Water, 2021, 1(8), 1837–1848 CAS.
- E. N. Hayta, M. J. Ertelt, M. Kretschmer and O. Lieleg, Bacterial Materials: Applications of Natural and Modified Biofilms, Adv. Mater. Interfaces, 2021, 8(21), 2101024 CrossRef CAS.
- M. Kretschmer, C. A. Schüßler and O. Lieleg, Biofilm Adhesion to Surfaces is Modulated by Biofilm Wettability and Stiffness, Adv. Mater. Interfaces, 2021, 8(5), 2001658 CrossRef CAS.
- M. Wells, R. Schneider, B. Bhattarai, H. Currie, B. Chavez and G. Christopher,
et al., Perspective: The viscoelastic properties of biofilm infections and mechanical interactions with phagocytic immune cells, Front. Cell. Infect. Microbiol., 2023, 13 Search PubMed.
- P. Somu, S. Narayanasamy, L. A. Gomez, S. Rajendran, Y. R. Lee and D. Balakrishnan, Immobilization of enzymes for bioremediation: A future remedial and mitigating strategy, Environ. Res., 2022, 212, 113411 CrossRef CAS PubMed.
- R. A. Sheldon and S. Van Pelt, Enzyme immobilisation in biocatalysis: why, what and how, Chem. Soc. Rev., 2013, 42(15), 6223–6235 RSC.
- R. Leu Alexa, R. Ianchis, D. Savu, M. Temelie, B. Trica and A. Serafim,
et al., 3D Printing of Alginate-Natural Clay Hydrogel-Based Nanocomposites, Gels, 2021, 7(4), 211 CrossRef CAS PubMed.
- K. Man, I. A. Barroso, M. Y. Brunet, B. Peacock, A. S. Federici and D. A. Hoey,
et al., Controlled Release of Epigenetically-Enhanced Extracellular Vesicles from a GelMA/Nanoclay Composite Hydrogel to Promote Bone Repair, Int. J. Mater. Sci., 2022, 23(2), 832 CAS.
- G. Cavallaro, M. R. Caruso, S. Milioto, R. Fakhrullin and G. Lazzara, Keratin/alginate hybrid hydrogels filled with halloysite clay nanotubes for protective treatment of human hair, Int. J. Biol. Macromol., 2022, 222, 228–238 CrossRef CAS PubMed.
- S. Elbassyoni, E. A. Kamoun, T. H. Taha, M. A. Rashed and F. A. ElNozahi, Effect of Egyptian Attapulgite Clay on the Properties of PVA-HES–Clay Nanocomposite Hydrogel Membranes for Wound Dressing Applications, Arabian J. Sci. Eng., 2020, 45(6), 4737–4749 CrossRef CAS.
- E. Farid, E. A. Kamoun, T. H. Taha, A. El-Dissouky and T. E. Khalil, PVA/CMC/Attapulgite Clay Composite Hydrogel Membranes for Biomedical Applications: Factors Affecting Hydrogel Membranes Crosslinking and Bio-evaluation Tests, J. Polym. Environ., 2022, 30(11), 4675–4689 CrossRef CAS.
- S. Feiz and A. H. Navarchian, Poly(vinyl alcohol) Hydrogel/Chitosan-Modified Clay Nanocomposites for Wound Dressing Application and Controlled Drug Release, Macromol. Res., 2019, 27(3), 290–300 CrossRef CAS.
- J. Nath, A. Chowdhury, I. Ali and S. K. Dolui, Development of a gelatin- g -poly(acrylic acid- co -acrylamide)–montmorillonite superabsorbent hydrogels for in vitro controlled release of vitamin B 12, J. Appl. Polym. Sci., 2019, 136(22), 47596 CrossRef.
- H. S. Samanta and S. K. Ray, Effect of polyethylene glycol and nano clay on swelling, diffusion, network parameters and drug release behavior of interpenetrating network copolymer, J. Appl. Polym. Sci., 2022, 139(8), 51678 CrossRef CAS.
- H. Bera and Y. Abbasi, Thakur. Curdlan/Clay Nanocomposite-Reinforced Alginate Beads as Drug Carriers, J. Polym. Environ., 2023, 32, 1–16 Search PubMed.
- M. Khachani, S. Stealey, E. Dharmesh, M. S. Kader, S. W. Buckner and P. A. Jelliss,
et al., Silicate Clay-Hydrogel Nanoscale Composites for Sustained Delivery of Small Molecules, ACS Appl. Nano Mater., 2022, 5(12), 18940–18954 CrossRef CAS.
- Y. Luo and D. K. Mills, The Effect of Halloysite Addition on the Material Properties of Chitosan–Halloysite Hydrogel Composites, Gels, 2019, 5(3), 40 CrossRef CAS PubMed.
- S. Amiri, Preparation and Characterization of Nanoclay-Based (Na-MMT and Bentonite) Polyacrylamide Hydrogels as Water Shut-Off Agent for Enhanced Oil Recovery, Silicon, 2019, 11(3), 1193–1203 CrossRef CAS.
- A. A. Roslan, N. A. A. Aziz, N. F. A. Deraman and I. Dzulkarnain, Effects of synthesis parameters on the performance of crosslinked Co-polymers with clays for conformance control, Pet. Res., 2023, S2096249523000534 Search PubMed.
- L. Chen, G. Li, Y. Chen, H. Zeng, Z. Mao and L. Liu,
et al., Thixotropy research of LAPONITE®-hydrogel composites for water shutoff in horizontal wells, J. Pet. Sci. Eng., 2022, 208, 109600 CrossRef CAS.
- T. Fan, Z. Liu, Y. Zhao, M. Li and X. Li, Synthesis of a tough montmorillonite/hydrogel composites for hot work of long-distance oil pipelines, Compos. Sci. Technol., 2022, 220, 109268 CrossRef CAS.
- D. Aydınoğlu, N. Karaca and Ö. Ceylan, Natural Carrageenan/Psyllium Composite Hydrogels Embedded Montmorillonite and Investigation of Their Use in Agricultural Water Management, J. Polym. Environ., 2021, 29(3), 785–798 CrossRef.
- H. Zhang, Y. Shi, X. Xu, M. Zhang and L. Ma, Structure Regulation of Bentonite-Alginate Nanocomposites for Controlled Release of Imidacloprid, ACS Omega, 2020, 5(17), 10068–10076 CrossRef CAS PubMed.
- Z. Dou, M. V. Bini Farias, W. Chen, D. He, Y. Hu and X. Xie, Highly degradable chitosan-montmorillonite (MMT) nano-composite hydrogel for controlled fertilizer release, Front. Environ. Sci. Eng., 2023, 17(5), 53 CrossRef CAS.
- A. Sinha and S. K. Khare, Mercury bioremediation by mercury accumulating Enterobacter sp. cells and its alginate immobilized application, Biodegradation, 2012, 23(1), 25–34 CrossRef CAS PubMed.
- R. I. Baron, G. Biliuta, V. Socoliuc and S. Coseri, Affordable Magnetic Hydrogels Prepared from Biocompatible and Biodegradable Sources, Polymers, 2021, 13(11), 1693 CrossRef CAS PubMed.
- R. Singh and B. Datta, Advances in Biomedical and Environmental Applications of Magnetic Hydrogels, ACS Appl. Polym. Mater., 2023, 5(7), 5474–5494 CrossRef CAS.
Footnote |
† These authors contributed equally. |
|
This journal is © The Royal Society of Chemistry 2024 |
Click here to see how this site uses Cookies. View our privacy policy here.