A framework to assess the terrestrial risk of antibiotic resistance from antibiotics in slurry or manure amended soils†
Received
6th December 2022
, Accepted 15th March 2023
First published on 3rd April 2023
Abstract
Antibiotic resistance (ABR) or the silent pandemic is a major global health and economic issue, threatening both modern healthcare and food production. There is increasing concern that the presence of antibiotics in the environment may select for the emergence and spread of antibiotic resistance. Currently environmental regulatory guidelines fail to address ABR risks, and while there is ongoing work to address this within aquatic environments, terrestrial systems have been somewhat overlooked – perhaps in part due to a focus on wastewater treatment plant effluent as the main source of antibiotics within the environment. Within agriculture there is an increasing push to move away from chemical-based fertilisers and towards the use of organic soil amendments such as slurry, manure or sludge, to improve soil health. However, these organic soil amendments have been shown to contain antibiotics and other pharmaceuticals alongside antibiotic resistant bacteria, posing a potential risk to the environment, livestock and humans through the proliferation and spread of ABR. It is therefore important that a risk framework is developed in relation to ABR and organic soil amendment use. Using current knowledge on the fate of antibiotics within soil and mathematical models, this manuscript presents a novel framework for assessing the terrestrial risk of antibiotic resistance through the use of farmyard manure as fertiliser.
Environmental significance
Antibiotic resistance (ABR) is a global health, economic and food security threat with the environment playing a key role in the dissemination of antibiotic-resistant bacteria, as low concentrations of antibiotics can select for ABR. Antibiotics are excreted largely unchanged by livestock and persist within manure or slurry. This manure of slurry is then used as fertiliser and the antibiotics are transferred into the soil where they have the potential to select for ABR. Here using a modelling framework that considers chemical properties of soil and antibiotics alongside reported concentrations of antibiotics in slurry this manuscript demonstrates that under certain conditions specific antibiotics may be present within the soil environment at concentrations that select for ABR.
|
1 Introduction
Antibiotics are a diverse group of pharmaceuticals used globally for the prevention and treatment of bacterial disease in humans, animals, and plants. These agents have saved millions of lives, revolutionising healthcare and agriculture in the process, and have become an integral part of modern medicine and society. However the increased use of antibiotics has led to a concomitant rise in the prevalence of antibiotic resistant bacteria and infections which are untreatable with modern antibiotics.1 The universal nature of the problem has been acknowledged by the World Health Organisation, who declared antibiotic resistance a global health and economic threat in 2015.2
Antibiotics are regularly detected in environmental matrices, including soil and water environments.3–9 Many antibiotic classes are excreted largely unchanged in animal and human waste10 reaching the environment either through manure or slurry application to land, direct excretion, or via the reuse of sludge from wastewater treatment plants (WWTPs) (Fig. 1).11 Within these same environments, antibiotic resistant (ABR) bacteria and antibiotic resistant genes (ARGs) are also present.3,12–14 ABR and ARGs are naturally present within the soil microbiome but their prevalence is increased by contamination with ABR bacteria from faecal matter.15 Studies have indicated that the presence of antibiotics in natural environments can enrich for ABR,13 which has led to the idea that there are potential hotspots for the development and spread of ABR within the environment.16 The potential of the environment to play a key role in the spread and development of ABR has been highlighted in both national and international reports2,17,18 all of which have highlighted the need for a ‘One Health’ approach in tackling this global problem. One Health involves collaborative efforts from multiple disciplines working locally, nationally, and globally to attain optimal health for people, animals, and our environment.
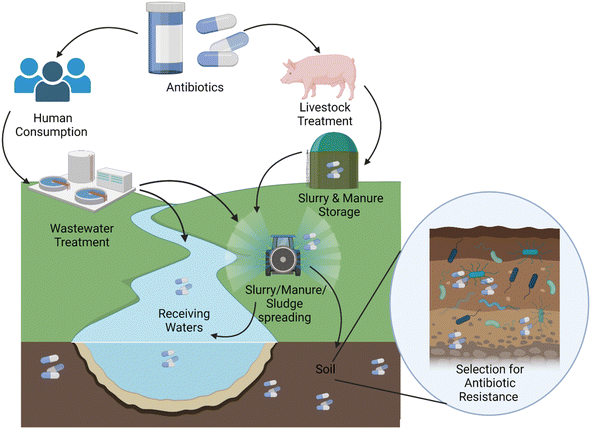 |
| Fig. 1 Pathways of antibiotic exposure into the environment. Antibiotics that are administered to humans and animals are excreted in their urine and faeces where they are often still in their active form. These antibiotics then make their way into different environments via direct excretion, wastewater treatment plants effluent and sludge or through the spreading of farmyard manure on land. Once in the environment they can select for antibiotic resistant bacteria. Biorender IM255UVJ17. | |
Research around antibiotics in the environment and their potential role in the development of ABR has largely focused on antibiotics and ARGs within the urban water cycle as wastewater treatment plants have been highlighted as a likely hotspot for ABR development and spread.19 Whilst a direct correlation between antibiotic concentrations and ARG copies within wastewater remains elusive, it is accepted that the anthropogenic presence of antibiotics in influent and effluent wastewater has the potential to select for ABR.16,20,21 However, wastewater treatment plants and the rivers they discharge into represents just one pathway by which antibiotics can enter the environment; another potentially important source of antibiotic release is agricultural use.
Agriculture is a key component of the ‘One Health’ approach to tackling ABR, but one that has been somewhat been overlooked regarding the fate of antibiotics within the environment. Antibiotics are a cornerstone of modern agriculture, not only to treat, control and prevent veterinary diseases but also in the management of crop disease. Agriculture has several different challenges to human healthcare; these include a competitive market with narrow profit margins and a need for high productivity where infectious disease can have a significant negative financial impact. The circular economy plays an important role in reducing overheads and increasing productivity within the agricultural sector. The recycling of slurry and manure, collectively referred to as farm yard manure (FYM), as a form of fertilizer has a central role within the agricultural circular economy enabling farmers to reduce overheads or providing another income source.22 In 2020, the British Survey of Fertiliser Practice reported that 65% of farms used organic fertilisers, equating to 93.9 million tonnes being applied to land per year.23 Therefore, it is vital we look to bridge the gap in knowledge on the partitioning of antibiotics during storage, treatment and spread of FYM on agricultural land, and the role it plays in the development and spread of ABR.
1.1 Current environmental risk assessments (ERA) – how environmental risk is currently assessed
The European Medicines Agency (EMA) framework for environmental risk assessments24 consists of two distinct phases, with the first phase designed to estimate the environmental exposure of the chemical under investigation and calculation of a predicted environmental concentration (PEC). The second phase then focuses on the environmental fate and ecotoxicity of the chemical, and involves calculation of a predicted no effect concentration (PNEC).24–26 This framework is not fit for purpose in the case of antibiotics in the environment, since it does not address the risk of selection of ABR. The microorganism-related toxicity tests (ASRIT or soil microorganism test) only measures an acute response to the antibiotic, with the ability of the microorganisms to continue to grow in its presence a positive result. This neglects to account for the fact this could indicate potential resistance to the antibiotic in the environment and fails to consider the wider implications in relation to ABR. It is also important to note that the idea of obtaining a low PEC to pass an environmental risk assessment is also not fit for purpose in understanding risk in the sense of antibiotic resistance, since it is well documented that very low concentrations of antibiotics can select and promote the development of ABR.27–29
Despite the current framework for ERA heavily weighted in favour of ABR selection, there have been concerted efforts to address this through the development of PNECr (Predicted No Effect Concentrations for Antibiotic Resistance), which are designed not to promote the development of ABR.30,31 Current methodology to obtain PNECr is based on EUCAST MIC (minimum inhibitory concentration) and environmental MSC (minimum selective concentration) data. This methodology has been reviewed elsewhere,32 and acknowledges that there is no standardised approach to calculate PNECr, in particular how to calculate minimum selective concentrations (MSC) within environmental compartments. How to apply these values within the aquatic environment is also under debate33 and there is the assumption that any bound antibiotic is inactive.34 To date, there has been little or no attempt to define PNECr for antibiotics within terrestrial systems, despite the fact soil micro-organisms harbour ARGs,35 and therefore selective enrichment of ARGs can occur in such environments due to the presence of antibiotics. Here we present the first framework to enable the application of current aquatic PNECr's to soil systems, thereby providing an assessment of ABR risk in the terrestrial environment.
2 Methods
Utilising previously determined PNECr for aquatic systems and knowledge of chemical fate in soils we developed a framework to assess the potential for antibiotics present in FYM to select for ABR within soil pore water. We demonstrate the use of this framework using six antibiotics commonly used in agriculture to provide the basis of a discussion on the terrestrial risk of ABR.
2.1 The framework
The presented methodology considers the application of FYM to land as the primary pathway by which antibiotics enter the terrestrial system. First environmental soil concentrations are predicted (PECsoil), then the soil sorption coefficient for the antibiotic is calculated and this applied to the PECsoil to calculate the bioavailable fraction (Fig. 2) for an antibiotic of interest. This framework therefore assumes that only bioavailable (non-adsorbed) antibiotics34 can select for ABR with the overall aim being to determine the soil pore water concentration (PECPW) of the antibiotic which is then compared to an aquatic PNECr to assess ABR risk.
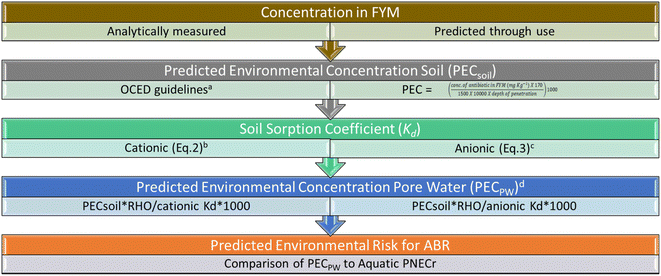 |
| Fig. 2 Framework for assessing risk for ABR following use of manure as a soil amendment. References a = OCED Guidelines;25 b = Droge and Goss 2013;36 c = Franco and Trapp;37 d = Carter et al. 2014.38 | |
2.2 Predicted environmental concentrations (PEC)
As starting point, concentrations of antibiotics in FYM can be predicted,25 measured or obtained from published literature sources. The OCED guideline25,39 (eqn (1)) is then used to calculate a PECsoil for the antibiotic, taking into consideration the method of application to the soil. This is important given the drive to use regenerative farming practices; to protect soil health, farmers are encouraged to use no-till farming over ploughing,40 which has been shown to influence the fate of antibiotics in soils (Nightingale et al. submitted Environmental Management) and will ultimately impact the bioavailable antibiotic concentration. |  | (1) |
To determine the Predicted Environmental Concentration Soil (μg kg−1) (PECsoil). Where; 170 is EU nitrogen spreading limit (N ha−1), 1500 is bulk density of dry soil (kg m−3), 10
000 is area of 1 hectare (m2 ha−1), depth of penetration either 0.25 or 0.05 m depending on whether incorporated or not, and 1000 is conversion factor (1000 μg mg−1).
2.3 Sorption behaviour of antibiotics in soil
Antibiotics, like other chemical substances in the environment, are distributed between solid, liquid, and gaseous phases depending on their chemical properties, with movement between the compartments being facilitated through water. The environmental fate of antibiotics, specifically the distribution between soil and soil pore water (also termed soil solution) is governed by sorption processes which can vary greatly depending on the properties of the antibiotics and the soil.41 The most common method to assess sorption is to measure the relationship between concentration of sorptive (e.g. antibiotic) and sorbate (e.g. soil) and calculate a soil sorption coefficient (Kd).42 The properties of soils such as pH, ionic environment, texture, and soil organic matter (SOM) alongside the amphiphilic and amphoteric properties of the antibiotic and their steric configuration have been shown to play a key role in soil sorption.42–47 The inherent variability in soil properties, coupled with the fact antibiotics are typically ionizable compounds and display pH-dependent sorption to soil, results in a range of experimentally-determined Kd's for a single antibiotic.41
Approaches to predict soil sorption therefore need to be able to account for these complexities. Simplified models built on linear relationships between Kow (n-octanol/water partition coefficient) and the soil organic carbon partitioning coefficient KOC, which assume sorption is driven by hydrophobic interactions, are not relevant for ionisable compounds, including antibiotics, where other processes (e.g. cation exchange) are known to dominate.36 For example, antibiotics such as tetracyclines and fluoroquinolones have very high sorption coefficients despite having low Kow. The use of Kow to predict KOC, as commonly done in phase I of the ERA, can lead to a significant underestimation of antibiotic sorption to soil.41 We therefore propose the use of charge-specific sorption models to predict Kd in this framework.
2.4 Soil adsorption coefficient models
Approaches to predict soil sorption coefficients have been recently reviewed elsewhere and compared to an independent experimentally-derived data set of pharmaceutical sorption coefficients.48 The model developed by Droge and Goss 2013,36 which assumes that sorption of cations is driven by cation exchange processes, was deemed the most appropriate model to estimate the sorption coefficients for pharmaceuticals in their cationic state (r2 of 0.29 for 66 compounds).48 | Kd(L kg−1) = KCEC CLAY(CECCLAY) + foc DOC ie | (2) |
Soil Sorption Coefficient for antibiotics in cationic state. Where KCEC CLAY = 1.22 Vx − 0.22 NAi +1.09 and DOC ie = 1.53 xVx + 0.32 xNAi − 0.27. (Vx = molecular volume L mol−1 and NAi = number of hydrogens bound by charged nitrogen). CECCLAY(meq. 100 g−1) = CECSOIL − 3.4 foc, where foc is percentage organic carbon.
For antibiotics in their anionic state, the model by Franco and Trapp37 predicts Koc values by combining the sorption contribution from neutral and ionised fractions. Lipophilic interactions for neutral molecules and soil are predicted using the octanol–water coefficient and the organic content of soil (SOM) which are combined with information on the pH of the soil and pKa of the pharmaceutical compound to predict the KOC for weak acids or anionic pharmaceuticals.37 This model was also assessed using an independent data set to evaluate its suitability to predict KOC for 68 compounds and it was deemed the most acceptable of models assessed for acids with an r2 of 0.17.48
| Koc(L kg−1) = Φn(100.54logPn+1.11) + Φion(100.11logPn+1.54) | (3) |
Soil organic carbon partitioning coefficient normalised to organic matter or antibiotics in anionic state. Where Φn is neutral fraction and Φion = ionic fraction37
| Kd(L kg−1) = (KOC× fOC)/100 | (4) |
Soil sorption coefficient for antibiotics in anionic state. Where KOC is the soil organic carbon partitioning coefficient normalised to organic matter (L kg−1) and fOC is the percentage of organic matter in soil.
2.5 Calculation of PECPW and comparison to PNECr
To select for ABR, antibiotics need to be bioavailable within their environment.49 On the assumption that adsorbed antibiotics are no longer bioavailable, we can utilise current research around the selection of ABR in aquatic environments to assess the potential risk of the applied organic amendment to select for ABR. To predict the bioavailable fraction of antibiotic in soil that have the potential to select for ABR, the PECPW is calculated, see eqn (5).38 | 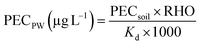 | (5) |
Predicted Environmental Concentration in Pore Water, where PEC soil is Predicted Environmental Concentration in soil (μg L−1) RHO is bulk density of soil (1500 kg m−3).
The PECPW for the antibiotic can then be compared to the published PNECr for aquatic environments (see ESI Table 1†)31 to assess the potential ABR risk associated with the application of slurry or manure on land through the calculation of an ABR risk quotient (RQ, see eqn (6)). This framework allows an interdisciplinary approach to enable the immediate assessment of the potential impact of antibiotics in FYM in relation to the selection of ABR within the terrestrial environment providing a timely step forward in this currently data-deficient area of ABR research.
| Risk Quotient (RQ) = PECPW ÷ PNECr | (6) |
ABR risk quotient (RQ). Where PECPW is the predicted concentration of antibiotic in soil pore water and PNECr is the predicted no effect concentration specific for antibiotic resistance selection.
2.6 Evaluation of framework
We have proposed a framework to assess the environmental risk of ABR following the application of FYM to agricultural land. To test this framework and provide an initial assessment of terrestrial ABR risk, we selected six antibiotics used in agriculture and followed each through our framework. These six antibiotics derive from four distinct chemical classes (tetracyclines, fluoroquinolones, sulfonamides and lincosamides), and were selected based on their common use within animal husbandry and reported presence in slurry, manure, and soil.6,9,50–52 The physicochemical properties and reported degradation rates in soil (DT50) for the selected antibiotics are summarised in Table 1.
Table 1 Physiochemical properties of the selected veterinary antibiotics and their respective chemical structuresa
Antibiotic and molecular weight (g mol−1) |
Chemical formula |
Antibacterial properties |
Excretion rate (active form) |
log Kow |
pKaa |
DT50 reported in literature (days) |
Reference; a = Drugbank.com; b = Rakonjac et al., 2012;53 c = De La Torre, 2012;54 d = Menz et al., 2019;55 e = Li et al., 2010;56 f = Slana et al., 2013;57 g = Blackwell et al., 2007;58 h = Berendsen et al., 2021;59 i = Ciprofloxacin Table Datasheet;60 j = Albero et al., 2018;52 k = Conde-Cid et al., 2020;9 l = Barra Caracciolo A. et al., 2018;61 m = Ccinelli et al., 2007;62 n = Abo El-Sooud K. et al., 2004;63 o = Cardenas-Youngs et al., 2015,64 p = Mehrtens et al., 2021.65
|
Tetracyclines
|
|
C22H24N2O8 |
Broad spectrum, bacteriostatic, and inhibits protein synthesis |
80% (cattle)a |
−1.30k |
7.8, 3.3 |
24.2c, 50d, 20.6–29.1e |
|
C22H24N2O9 |
Broad spectrum, bacteriostatic, and inhibits protein synthesis |
23% (cattle)b, 60% (pig)b |
−0.90k |
7.3, 3.3 |
16–62f, 38.8c, 21.7g, 30.2–41.3e, 8, 9h |
![[thin space (1/6-em)]](https://www.rsc.org/images/entities/char_2009.gif) |
Fluoroquinolones
|
|
C17H18FN3O3 |
Broad spectrum, bactericidal and inhibits DNA replication |
Up to 85%i |
0.28j |
5.9, 8.89 |
32–100l |
|
C19H22FN3O3 |
Broad spectrum, bactericidal and inhibits DNA replication |
90% & up to 10% CIPf |
1.1 |
6.4, 7.8 |
99–696f, 297d, 137h |
![[thin space (1/6-em)]](https://www.rsc.org/images/entities/char_2009.gif) |
Sulfonamides
|
|
C12H14N4O2S |
Broad spectrum, bacteriostatic, and inhibits tetrahydrofolate biosynthesis |
80%(cattle)m, 50% (pig)m |
0.89k |
2.07, 7.49 |
3.5c |
![[thin space (1/6-em)]](https://www.rsc.org/images/entities/char_2009.gif) |
Lincosamides
|
|
C18H34N2O6S |
Narrow spectrum, bacteriostatic and inhibits protein synthesis |
40% (pigs)n |
0.2j |
7.87p, 12.37p |
82.5c, 1.1, 11h |
FYM concentrations were obtained from the literature (ESI Table 2†) and for the purposes of this evaluation, a range of reported concentrations for each antibiotic was used to showcase the variability typically associated with this exposure. The modelled application scenarios included broadcast (non-ploughed) and incorporated (ploughed) soil following a single application of FYM. For the modelled soil, including properties used in eqn (2) and (3), average properties of soils from across the globe was used.66 These values can, however, be changed if the parameters of the soil where the FYM is to be applied are known. Two pH values were set at 5 and 8.7 to cover a range of pH reported from 100 soils by Khaledian et al.66 while the fOC or organic carbon content was set at 4.27% also reported within the same manuscript.66 The pH of the soil, either 5 or 8.7, then dictated the soil sorption coefficient modelled used depending on ionisation of the antibiotic (ESI Table 3†). For antibiotics mainly present in their cationic form, eqn (2) was used with the value for CECsoil set at 27.9885 meq. 100 g−1.66 For antibiotics present mainly in their anionic form, eqn (3) was used.37 For antibiotics with multiple pKa values, the pKa value which resulted in the strongest ionisation was used to determine the soil sorption model used.
3 Results and discussion
A literature search for concentrations of the six test antibiotics in FYM revealed a range of concentrations for each antibiotic (See ESI Table 2†). Upper and lower concentration levels from these ranges were applied to the proposed framework to assess the risk of selection for ABR within the terrestrial environment following application of that FYM to land. Tables 2 and 3 summarise the results using the highest reported concentration of each of the six antibiotics under four different conditions – ploughed soil pH 8.7, ploughed soil pH 5, non-ploughed soil pH 8.7 and non-ploughed soil pH 5 – with additional results provided in ESI Tables 3–5.† These results demonstrate that the method of FYM application and the pH of the receiving soils play a key role in the associated ABR risk from applying FYM to agricultural land.
Table 2 Ploughed or incorporated FYM framework results for the top concentration for each antibiotic reported in literature
Antibiotic |
FYM conc. (mg kg−1) |
PECsoil (μg kg−1) ploughed |
Soil pH |
%Ionised |
Soil sorption model |
K
d (L kg−1) |
PECPW (μg L−1) |
PNECr (μg L−1) |
RQ |
Eqn (3) – Franco and Trapp(2008).37
Eqn (2) – Droge and Goss (2013).36
If PNECr of 16μgL−1 for sulfamethoxazole is used.
|
TET |
300 |
13.6 |
8.7, 5.0 |
66.9, 32.8 |
Eqn (3),aeqn (2)b |
0.0007, 5958.2 |
2871.48, 3.42 × 10−3 |
1.0 |
2871, 3,4 × 10−3 |
OXY |
700 |
34.9 |
8.7, 5.0 |
74.7, 32.9 |
Eqn (3),aeqn (2)b |
0.0002, 6172 |
25567.09, 8.48 × 10−3 |
0.5 |
51134, 0.017 |
CIPRO |
43 |
0.4 |
8.7, 5.0 |
70.8, 2.2 |
Eqn (3),aeqn (2)b |
0.26, 5038.8 |
23.73, 1.21 × 10−3 |
0.06 |
396, 0.002 |
ENRO |
60.2 |
2.73 |
8.7, 5.0 |
92.9, 3.8 |
Eqn (3),aeqn (2)b |
5.73, 6434.8 |
0.71, 6.36 × 10−4 |
0.06 |
12, 0.001 |
SMZ |
8.0 |
0.36 |
8.7, 5.0 |
97.1, 50.1 |
Eqn (3),a NA |
8.57, NA |
0.063, 1.2 × 10−4 |
No PNEC |
0.004c |
LINCO |
227 |
10.29 |
8.7, 5.0 |
22.7, 0.01 |
Eqn (2),beqn (2)b |
7818.3 |
1.97 × 10−3 |
2 |
9.8 × 10−4 |
3.1 Tetracyclines
Tetracyclines are a class of broad-spectrum antibiotics characterised by a four-hydrocarbon ring backbone. In view of their regular use in agriculture, they are commonly found within manure,6,50 slurry50 and agricultural soil.9 Tetracyclines, like many antibiotics, are ionisable and have multiple acid dissociation coefficients, which means that they can exist in either cationic, anionic or zwitterionic form depending on the pH of the environment. This we know plays a key role in their sorption to soil and fate within the terrestrial environment. As such, reported soil sorption coefficients for tetracyclines within literature vary greatly.11 It is therefore essential that the mathematical model best represents the fate of these antibiotics in a particular physiochemical form.
The soil sorption coefficient Kd of TET varies greatly depending on whether modelled in their anionic or cationic state, which in turn is dictated by the pH of the environment they are in. When within a soil environment of pH 8.7, there is 66.9% acid ionisation giving tetracycline an overall negative charge (anionic), and thus a low modelled soil sorption coefficient of 0.0007 L kg−1 when modelled using eqn (3). However, when tetracycline (TET) is within a more acidic environment (soil at pH 5), the total acid ionisation drops to 32.8%, giving tetracycline an overall positive charge (cationic); under such conditions, eqn (2) would be more suitable for predicting soil sorption within this environment, and the predicted Kd increases very substantially to 5958.2 L kg−1.
The FYM concentrations for TET reported within the literature range from 0.16–300 mg kg−1 (ESI Table 2†), which translate into PECsoil concentrations of 0.036–68 μg kg−1 for non-ploughed soils or 0.007–13.6 μg kg−1 for ploughed soil. The lower concentration for ploughed soils is a result of the increased penetration depth, allowing for increased migration of the antibiotic from the site of application. When a soil pH of 8.7 was modelled for non-ploughed soil, FYM concentrations of TET greater than 0.02 mg kg−1 resulted in a PECPW greater than the PNECr for tetracycline of 1 μg L−1. Based on the reported FYM concentrations in ESI Table 2,† 100% of manures would exceed this threshold, with risk quotients (RQ) ranging from 7.7–14357 (an RQ greater than 1 indicates there is risk for the selection of ABR). For FYM incorporated or ploughed into soil a TET concentration of greater than 0.1 mg kg−1 resulted in a PECPW higher than the PNECr. Comparatively, when the pH 5 scenario was used in this framework, and tetracycline was strongly sorbed to the soil, none of the reported concentrations of tetracycline in FYM resulted in a PECPW greater than the PNECr of TET. The framework can also be used to calculate the concentration of TET in FYM that would give rise to a PECPW greater than the PNECr, and thus a risk quotient of greater than 1. For a ploughed soil with a pH of 5, this concentration would have to be greater than 90
000 mg kg−1 and for non-ploughed soil 19
000 mg kg−1 which is 300 and 63 times greater respectively than the highest TET concentration reported in FYM in published literature.
Oxytetracycline (OXY) presents a similar scenario, with a low predicted Kd of 0.0002 L kg−1, when within a soil environment of pH 8.7 due to being predominantly negatively charged or in an anionic state. When present in acidic soil (pH 5), OXY has a Kd of 6172 L kg−1 due to the fact it is predominately positively charged. The concentrations for OXY reported within FYM were significantly higher than for those of TET, with concentrations ranging from 4.8 mg kg−1 to 700 mg kg−1 (ESI Table 2†), despite livestock dosing rates for both drugs being similar,67–69 with dosing rates of between 3–8 mg kg−1. This variability could be due to the differences in numbers of animals treated, treatment method and time, or the storage conditions of the FYM. Translated through the proposed framework these concentrations give PECsoil for OXY of 1.09–174.53 μg kg−1 for non-ploughed application and 0.22–34.9 μg kg−1 for ploughed (ESI Table 3†). These PECsoil values are comparable to reported concentrations within agricultural soil.70–72
The PNECr for oxytetracycline of 0.5 μg L−1 was exceeded in this framework for all scenarios when FYM was applied to soils with a pH of 8.7, whether ploughed or not (ESI Table 3†), with risk quotients ranging from 1600 to 255
671 for non-ploughed soil, and 160–25567 for ploughed soil. At pH 5, even the highest reported OXY concentrations in FYM would not lead to a PECPW greater than PNECr; indeed, the concentration of OXY would have to exceed 90 mg kg−1 and 4600 mg kg−1 in FYM when applied to non-ploughed and ploughed soil, respectively if PECPW were to exceed the PNECr, to give rise to a risk quotient greater than 1, in soil with a pH of 5. Comparatively, for ploughed soils with a pH of 8.7 any FYM with a concentration of greater than 0.015 mg kg−1 there is potential for the PECPW to exceed the PNECr for OXY, while for non-ploughed soils (pH 8.7) a FYM concentration of 0.003 mg kg−1 or greater would lead to a PECPW greater than the PNECr.
The worldwide average agricultural soil has a pH range from 5–8.7 (ref. 66) meaning potentially both TET and OXY could exist predominately within soil in their anionic form, which is when these antibiotics are most bioavailable and there is the potential to exceed the PNECr. The half-lives of both TET and OXY are between 8–62 days,54,55,57–59 suggesting that environmental persistence of these compounds is likely within soil11 and that concentrations of these antibiotics are unlikely to return to negligible levels between slurry applications. There is therefore potential for concentrations to build over time, highlighting two further research questions that need answering in relation to antibiotics in the terrestrial environment. Firstly, is there a maximum sorption capacity of soil, and secondly is the risk linear as antibiotic concentrations increase?
3.2 Fluoroquinolones
Fluoroquinolones are a class of broad-spectrum synthetic antibiotics whose presence in the environment has become of increasing concern due to their ecotoxicity, their role in the spread of ABR and other potential adverse effects on human health they may have, with ciprofloxacin being placed on the Water Watch List.73 Like many other antibiotics, fluoroquinolones are also ionisable compounds, which depending on pH can either be anionic, cationic or zwitterionic, in turn influencing their environmental fate.
Two fluoroquinolones, ciprofloxacin (CIP) and enrofloxacin (ENRO), were evaluated using the proposed modelling framework in soils with a pH of 5 and 8.7. CIP is the most widely prescribed fluoroquinolone worldwide74 and is also a metabolite of ENRO,75 which is one of the most used and important FQ within agriculture.74 In a soil with pH 5, both antibiotics were present mainly within their cationic form and thus eqn (2) was used36 to predict Kd values of 5038.8 L kg−1 and 6434.8 L kg−1 for CIP and ENRO respectively (Table 3). Figuerou-Diva et al., 2010 (ref. 46) calculated the Kd of CIP and ENRO in a range of soils with different pH values, the Kd calculated at pH5 ± 0.1 ranged from 140–54600 L kg−1 for CIP and 120–33
600 L kg−1 for ENRO. However, the soils used within these experiments varied greatly in respect of organic carbon content (0.3–8.9%) and cation exchange capacity (2.4–62 cmol kg−1), both of which are known to influence sorption of antibiotics to soil.44 This proposed framework however enables one to take these variables into account and our predicted Kd's sit within the range of Kd's measured by Figuerou-Diva et al., 2010.46 To predict the Kd for both antibiotics in a pH 8.7 soil, eqn (3) (ref. 76) was used as the antibiotics were predominantly anionic, which resulted in Kd values of 0.26 and 5.73 L kg−1 for CIP and ENRO, respectively.
Table 3 Non-ploughed or not incorporated FYM framework results for the top concentration for each antibiotic reported in literature. Eqn (3) – Franco and Trapp 2008.37Eqn (2) – Droge and Goss 2013 (ref. 36)
Antibiotic |
FYM conc. (mg kg−1) |
PECsoil. (μg kg−1), non ploughed |
Soil pH |
%Ionised |
Soil sorption model |
K
d (L kg−1) |
PECPW (μg L−1) |
PNECr (μg L−1) |
RQ |
If PNECr of 16μgL−1 for sulfamethoxazole is used.
|
TET |
300 |
68 |
8.7, 5.0 |
66.9, 32.8 |
Eqn (3), eqn (2) |
0.0007, 5958.2 |
14357.41, 1.7 × 10−2 |
1.0 |
14357, 1.7 × 10−2 |
OXY |
700 |
174.5 |
8.7, 5.0 |
74.7, 32.9 |
Eqn (3), eqn (2) |
0.0002, 6172 |
127835.45, 0.04 |
0.5 |
255671, 0.085 |
CIPRO |
43 |
9.75 |
8.7, 5.0 |
70.8, 2.2 |
Eqn (3), eqn (2) |
0.26, 5038.8 |
118.67, 6.07 × 10−3 |
0.06 |
1978, 0.01 |
ENRO |
60.2 |
13.6 |
8.7, 5.0 |
92.9, 3.8 |
Eqn (3), eqn (2) |
5.73, 6434.8 |
3.57, 3.18 × 10−3 |
0.06 |
60, 3.7 × 10−5 |
SMZ |
4.9 |
1.1 |
8.7, 5.0 |
97.1, 50.1 |
Eqn (3), NA |
8.57, NA |
0.32, NA |
No PNEC |
0.02a |
LINCO |
227 |
51.5 |
8.7, 5.0 |
22.7, 0.01 |
eqn (2), eqn (2) |
7818.3 |
9.87 × 10−3 |
2 |
4.9 × 10−3 |
In comparison to the tetracyclines, reported concentrations for the two fluoroquinolones CIP and ENRO in FYM were lower and ranged from 0.06–43 mg kg−1 for CIP and 0.14–60.2 mg kg−1 for ENRO (ESI Table 2†). However, their PNECr values were also considerably lower at 0.06 μg L−1. When the ABR risk in the terrestrial environment for these two antibiotics was assessed using the proposed framework, PECPW concentrations were highest in non-ploughed soils with a pH of 8.7 (ESI Table 4†), calculated at 0.08–118.67 μg L−1 for CIP, and 0.01–3.57 μg L−1 for ENRO. For ploughed soils with the same FYM concentrations, PECPW ranged from 0.02–23.73 μg L−1 (CIP) and 0.002–0.71 μg L−1 (ENRO). All five of the reported FYM concentrations for CIP6,50,77 resulted in exposures which exceeded the PNECr, with risk quotients ranging from 1.4–1978, in unploughed soil at pH 8.7 (ESI Table 4†), whereas in ploughed soils all reported concentrations bar the lowest (0.06 mg kg−1) exceed the PNECr, or had a risk quotient of greater than 1. Therefore, the application of FYM to soil presents a clear risk for the selection of FQ resistant micro-organisms. Three of the five concentrations of ENRO6,78 when applied either unploughed or ploughed soils with a pH of 8.7 gave a PECPW concentration of greater than the PNECr. In comparison the PECPW for both antibiotics within pH 5 soil, ploughed or unploughed, were considerably lower due to the higher Kd values for both antibiotics as the soil pH decreased, representing minimal risk for the selection of ABR, with risk quotients of 0.00005–0.01 for CIP and 0.0004–0.05 for ENRO, see ESI Table 4.†
As a class of antibiotics, fluoroquinolones have some of the longest half-lives in soil with reported DT50's ranging between 32–100 days for CIP(61) and 99–696 days for ENRO55,57,59 meaning that with additional applications of slurry or manure to the land (2 or 3 applications) between 1st February and 15th October (255/6 days per modelled year) there is significant potential for the accumulation of these antibiotics in the soil environment. As with tetracyclines, there is a clear knowledge gap regarding the potential loading of soils and whether there is a maximum capacity for sorption of antibiotics. Nevertheless, these results show that there is the potential for pore water concentrations to rise above the PNECr. This again highlights the importance of considering the application scenario when evaluating risk alongside the potential for a maximum antibiotic load that solid matter in soil can adsorb.
3.3 Sulfonamides
Sulfonamides are one of the most heavily used ABs worldwide.79 Sulfamethazine (SMZ) is commonly used in agriculture80 and was modelled here as an example. SMZ has two pKa values of 2.07 and 7.49, meaning it exists in its cationic form in environments with a pH of less than 2. As worldwide soil environments are unlikely to reach a pH of 2, the cationic form of SMZ is unlikely to be found within the average worldwide terrestrial environment (pH range 5–8.5).66 Instead, SMZ will most likely be in either its zwitterionic or anionic form (Table 3). Hence, the anionic form was modelled at a pH of 8.7 utilising the Franco model,76 yielding a Kd value of 8.57 L kg−1. In the literature, reported concentrations of SMZ in FYM range from 0.03 mg kg−1 to 8 mg kg−1(ESI Table 2†). Taking the highest concentration of 8 mg kg−1,81 which was reported in chicken manure, and applying this proposed framework, a PECPW of 0.063 μg L−1 was calculated for ploughed soils and 1.81 μg L−1 in non-ploughed soils (ESI Table 4†). Currently, there are no published PNECr values for SMZ.82 However, a PNECr published for sulfamethoxazole30 (SMX) – a member of the same drug class with similar chemical properties,83 same mode of action84 and resistance breakpoint85,86 allows for an initial evaluation of risk. The PNECr of SMX is 16 μg L−1, which is over 8 times higher than the highest PECPW for SMZ (1.81 μg L−1), giving a risk quotient for PECPW ranging from 0.000015 to 0.02 for SMZ depending on application method. Whilst not definitive, this result would suggest that the risk of applying FYM with residues of SMZ in relation to ABR is low. There is however a clear research gap in the lack of a PNECr for SMZ. At pH 5, SMZ would be in its zwitterionic form; given the lack of models developed to predict sorption of compounds with multiple charges, the present framework is unable to accurately model PECPW at this pH.
3.4 Lincosamides
Lincomycin (LINCO) is commonly used within the swine industry for the treatment of dysentery in weaned animals.87 With a pKa of 7.97 and 12.37, it will exist predominantly within soils of pH 5 in its cationic form where it is sorbed to solid matter within the soil through electrochemical interactions. Consequently, Kd values were modelled using eqn (2),36 resulting in a predicted Kd of 7818.34 L kg−1. It has been reported that it is not possible to derive experimental Kd's for LINCO when in its cationic form due to its strong affinity to bind to soil.88 In soils with a pH of 8.7, LINCOwill exist in its zwitterionic form, meaning this framework is unable to accurately model the PECPW at this pH. However, experimental Kds have been reported for soils with a pH of between 7.3 and 8.3, and these ranged from 2–210 L kg−1.6,50 These values are considerably lower than the Kd modelled within this framework for soils with a pH of 5 but higher than if eqn (3) (ref. 76) was used to model the LINCO Kd (0.0158 L kg−1).
Reported concentrations of LINCO within slurry or manure ranged from 0.36 to 227 mg kg−1 (ESI Table 2†), with the highest concentration being reported in swine slurry in Spain.6 Such values correspond to PECsoil concentrations between 0.02 and 10.29 μg kg−1 for ploughed soil, and 0.018–51.45 μg kg−1 for non-ploughed soil (ESI Table 5†). As it was not possible to model the soil sorption coefficient at a pH of 8.7, only the results for pH 5 soils are reported. This resulted in PECPW of 3.13E-06-1.97 × 10−3 μg L−1 (non-ploughed) and 1.57 × 10−5 − 9.87 × 10−3 μg L−1 (ploughed). In comparison to PNECr for LINCO of 2 μg L−1, the modelled PECPW for LINCO in soil with a pH of 5 generated a risk quotient for ABR of between 1.57 × 10−6 and 4.96 × 10−3 indicating that within this setting LINCO is unlikely to select for ABR. This conclusion does not, however, consider any preloading of the soils.
4 Summary
Application of this framework to measured concentrations of the four chemical different classes of antibiotics reveals a clear environmental risk for the selection of ABR through the application of farmyard manure containing tetracyclines and fluoroquinolones to agricultural soil. The level of risk depends upon the pH of the soil and the method of application. Both classes of antibiotics also have relatively long half-lives within the terrestrial environment, with values of 8–50 days54–59 being reported for tetracyclines and between 32–100 days for fluoroquinolones,55,57,59,61 meaning there is also the potential that this risk will increase further upon multiple applications of farmyard manure. By contrast, our analysis revealed a minimal risk with respect to the development of environmental antibiotic resistance for lincomycin, where PECPW values were up to 200-times lower than the PNECr for lincomycin. In our evaluation of the environmental ABR risk for sulfamethazine, there were two key knowledge gaps that need to be addressed to truly predict risk; first, the calculation of a PNECr for sulfamethazine and second, the development of sorption models for zwitterionic compounds. In this framework we predicted the risk based on current scientific knowledge using PNECr for sulfamethoxazole, an antibiotic from the same class as sulfamethazine and only within soils with a pH of 8.7 where sulfamethazine is in its anionic form with PECPW for sulfamethazine being at least 8 times lower than the PNECr for sulfamethoxazole. Sulfamethazine and lincomycin have relatively short half-lives, 3.5 days and 1.1–82.5 days respectively,54,59 and therefore are not expected to exceed the concentration required to select for resistance within soil (16 μg L−1 and 4 μg L−1 for sulfamethazine and lincomycin, respectively). The soil properties used within this analysis were based on properties of average worldwide agricultural soils, and these risks will alter as soil properties such as pH, cation exchange capacity and organic matter of the soil changes. It is therefore possible that evaluating the sorption and partitioning of antibiotics on a field-by-field basis may alter the ABR risk profile.
Previous research has shown that the addition of slurry or manure to land drives an increase in soil pH,89 with the increase in organic matter suppressing the acidification of soils that is commonly seen when nitrogen based chemical fertilisers are used.90 This increase in pH has the potential to increase the bioavailability, and therefore the selective pressure of tetracycline and oxytetracycline in the pore water, in turn increasing the risk of selection of ABR within the soil environment. This highlights the importance of considering the application method and pathways by which the antibiotics enter the environment, as this can alter the soil properties and ultimately the fate of the antibiotic.
4.1 Framework limitations
Currently the majority of regulation around antibiotics and their role in the spread of ABR within the environment has focused on the aquatic environment91 with a drive to set guidelines for ABs within effluent wastewater (industrial and municipal). This focus has meant that there is a significant data gap in relation to the assessment of AB and ABR in the soil environment.
Using our current understanding of the fate of ABs within soil, alongside our current knowledge on selection of ABR within the environment, this manuscript proposes a standardised framework to assess ABR risk of applying slurry and manure to agricultural land. This has been achieved by applying a standardised approach to calculate a soil sorption coefficient for the antibiotic in question within the soil to which is it being applied. From this, the assumed bioavailable fraction of the antibiotic in soil pore water can be calculated and compared to current PNECr's. Our framework therefore enables current PNECr's, which have been developed for aquatic systems, to be utilised within a terrestrial setting and fill the data gap around the assessment of ABR risk in soil from the application of slurry and manure.
However, it is important to highlight where knowledge gaps exist which limit the application of this framework and suggest areas to target future research efforts to refine risk assessment approaches to consider terrestrial ABR risk. Current limitations include an understanding of the:
(1) maximum capacity of soils to adsorb antibiotics and other emerging contaminants;
(2) potential mixture effects on sorption and subsequent role in the selection of ABR;
(3) methods to model the soil sorption coefficient of zwitterions;
(4) extent that sorbed antibiotics exert a selection pressure for ABR;
(5) relevance of aquatic PNECr to assess resistance risk in terrestrial systems;
(6) impact of other environmental factors on the selection of ABR including temperature, available nutrients, carbon sources, the presence of heavy metals and of other emerging contaminants including ARGs.
To fully assess the ABR risk of applying antibiotic containing slurry, manure, or other organic amendment such as biosolids to agricultural land, we need to fully understand their fate within the terrestrial environment. Key to this understanding is whether there is a maximum capacity for antibiotic sorption in soils. This is important as current models for predicting the sorption of ABs to soils assumes all sorption sites are available. However, sorption relies on the ability of the AB to access sorption sites within the solid matter; if these are already occupied, sorption will be decreased, and soil pore water concentrations will be higher. Our framework does not consider the potential preloading of soils with antibiotics (and other chemicals) and the impact this may have on the bioavailable fraction of the antibiotic within pore water. Competitive and synergistic sorption of ionisable pharmaceuticals including antibiotics has been reported within soils, including the competitive sorption of fluoroquinolones within wetland soils92 but also the decreased sorption of other pharmaceuticals in the presence of certain antibiotics.93 To date, there have been no comprehensive studies on the maximum sorption nor the competitive sorption of a full range of antibiotics within different soils. An understanding of how preloading and competitive sorption of antibiotics alters antibiotic fate, and thus bioavailability together with the factors which influence this, is needed to refine models used within this framework.
The fate of the antibiotics is strongly linked to their charged state in the soil, with some antibiotics possessing both cationic and anionic charged functional groups. The presented framework accounts for the ionisation of antibiotics using charge-specific sorption models, but there is currently a knowledge gap on how to successfully model the sorption of zwitterions.48 The framework therefore does not take this into account and crudely estimates the Kd values when antibiotics are either in their cationic or anionic state which may not fully represent their charged states within the soil, this is particularly true for tetracyclines. Zwitterionic models have been developed their SD is still relatively high compared to experiment data.94 To be able to fully predict the fate of antibiotics within terrestrial systems – and therefore their potential risk – this knowledge gap needs to be filled.
Using predicted pore water concentrations, the framework assumes that sorbed antibiotics do not exert any biological impact in relation to ABR or toxicity and only the bioavailable fraction (i.e., concentration in porewater) is relevant. This is based on the idea that antibiotics, once sorbed, have a higher affinity for the soil than the organism.34 However, data regarding the bioavailability of sorbed antibiotics to soil bacteria are sparse, and the studies that have been conducted report contrasting results.95–97 In comparison to antibiotics, there have been extensive studies into the role that soil bacteria play in the fate and degradation of other organic contaminants such as polyaromatic hydrocarbons (PAHs) and polychlorinated biphenyls (PCBs), where it has been shown that certain soil bacteria are able to access soil sorbed PAHs and PCBs.98,99 This is probably due to extracellular substances produced by the soil bacteria that enable de-sorption of the PAHs and PCBs,97 enabling the soil bacteria to utilise the PAHs and PCBs as a carbon source. While we typically consider antibiotics as harmful to bacteria – as something they want to avoid – it is important to recognise that we do not truly understand the role of these antibiotics within the wider soil microbiome. This is especially the case since it has been shown that bacteria can utilise antibiotics as a carbon source,100,101 indicating the potential for soil bacteria to influence the sorption of antibiotics within soil. Further research is needed to establish if this is the case or if an additional step is needed in the model to account for the risk derived from sorbed antibiotics.
Finally, current PNECr values have mainly been developed to mitigate against the risk of ABR development in aquatic systems, with little thought to antibiotics selecting for ABR in terrestrial environments. PNECr targets do not include every antibiotic nor even every antibiotic class and the MIC/MSC data91 used to generate them does not cover every bacterial genus. Many bacteria present within terrestrial systems are not culturable within the laboratory102 meaning use of the current MIC/MSC modelling approach for PNECr's may not necessarily protect against ABR development in the actual soil environment. It is also important to highlight that methods to determine MSCs for different microbes or microbial communities are not currently standardised, meaning data published is not always comparable and may only include the impact of the antibiotic on a small subset of the soil microbiome. This is a very large knowledge gap within the setting of safe and protective targets for antibiotics within all environmental compartments, and there needs to be universal agreement over the scientific methods used to establish PNECr values32 and the bacterial communities tested.
5 Conclusion
This manuscript proposes a framework to allow PNECr values developed for aquatic systems to be applied to soil through the modelling of predicted pore water concentrations of ABs using pH-dependent sorption models. Slurry and manure application scenarios are based on realistic exposures following current EMA25 guidance. By considering the impact of soil pH on antibiotic fate and method of manure and slurry application, we can evaluate a range of realistic exposure scenarios with the potential to extrapolate this approach to long-term repeated applications. As highlighted, there are several limitations to this framework that span the chemical–biological interface. Specifically, sorption models need to be developed for antibiotics which have multiple pKa values and we need to improve our understanding around whether there is a maximum load of antibiotic that can be adsorbed to solid matter within the soil and how this relates the development of resistance to consider repeat application of organic fertilisers. The biological knowledge gaps are centred on the use of aquatic based PNECr's and specifically whether soil micro-organisms were represented within this assessment, as well as the need to confirm the assumption that adsorbed antibiotics do not exert a biological impact.
Nevertheless, this framework provides an important step forward in evaluating the terrestrial component of a ‘one health, one environment’ approach to consider ABR, presenting a feasible solution for quickly and easily assessing the risk of applying soil amendments such as slurry or manure containing antibiotics to agricultural fields based on the current state of both the chemical and microbiological knowledge. As the questions raised are answered and knowledge gaps filled, this framework can be easily adapted to include them. It is vital to develop this framework now, even before all the knowledge gaps have been answered, given the drive to reduce our ‘waste’ and adopt circular economy principles, this in turn encourages the re-use of resources within the agricultural setting. Beyond the determination of ABR risk in soils, this framework paves the way for the development of a potential set of guidelines on permissible AB concentrations in slurry or manure to enable their safe use as agricultural fertilisers.
Abbreviations
ABR | Antibiotic resistance |
ARG | Antibiotic resistant gene |
CEC | Cation exchange capacity |
f
oc
| Fraction of organic carbon |
FYM | farmyard manure |
K
d
| Soil sorption coefficient |
K
OC
|
K
d corrected for organic carbon content |
MIC | Minimum inhibitory concentration |
MSC | Minimum selective concentration |
PEC | Predicted environmental concentration |
PNEC | Predicted no effect concentration |
PNECr | Predicted no effect concentration for antibiotic resistance |
Author contributions
Felicity Elder: conceptualisation, writing, data collection and interpretation; Alex O'Neill: conceptualisation, supervision, contribution to drafting the manuscript; Lisa Collins: conceptualisation, supervision; Laura Carter: conceptualisation, writing, supervision.
Conflicts of interest
The authors can confirm that the research was conducted in the absence of any commercial or financial interests that would be construed as potentially a conflict of interest.
Acknowledgements
Funding for Felicity C T Elders time was provided through a University of Leeds Pump Priming Grant for interdisciplinary projects. Laura J Carter's time was funded from a UK Research and Innovation (UKRI) Future Leaders Fellowship (Grant Number MR/S032126/1).
References
- J. Bengtsson-Palme and D. G. J. Larsson, Protection goals must guide risk assessment for antibiotics, Environ Int., 2018, 111, 352–353, DOI:10.1016/j.envint.2017.10.019.
-
World Health Organization, Global Action Plan on Antimicrobial Resistance, 2015 Search PubMed.
- A. C. Singer, H. Shaw, V. Rhodes and A. Hart, Review of antimicrobial resistance in the environment and its relevance to environmental regulators, Front Microbiol., 2016, 7, 1–22 Search PubMed.
- M. Qiao, G. G. Ying, A. C. Singer and Y. G. Zhu, Review of antibiotic resistance in China and its environment, Environ Int., 2018, 110, 160–172, DOI:10.1016/j.envint.2017.10.016.
- D. G. J. Larsson, Pollution from drug manufacturing: Review and perspectives, Philos. Trans. R. Soc. B, 2014, 369(1656) DOI:10.1098/rstb.2013.0571.
- M. Gros, J. Mas-Pla, M. Boy-Roura, I. Geli, F. Domingo and M. Petrović, Veterinary pharmaceuticals and antibiotics in manure and slurry and their fate in amended agricultural soils: Findings from an experimental field site (Baix Empordà, NE Catalonia), Sci. Total Environ., 2019, 654, 1337–1349 CrossRef CAS PubMed.
- P. Li, Y. Wu, Y. He, B. Zhang, Y. Huang and Q. Yuan,
et al., Occurrence and fate of antibiotic residues and antibiotic resistance genes in a reservoir with ecological purification facilities for drinking water sources, Sci. Total Environ., 2020, 707, 135276, DOI:10.1016/j.scitotenv.2019.135276.
- I. T. Carvalho and L. Santos, Antibiotics in the aquatic environments : A review of the European scenario, Environ Int., 2016, 94, 736–757, DOI:10.1016/j.envint.2016.06.025.
- M. Conde-Cid, A. Núñez-Delgado, M. J. Fernández-Sanjurjo, E. Álvarez-Rodríguez, D. Fernández-Calviño and M. Arias-Estévez, Tetracycline and sulfonamide antibiotics in soils: Presence, fate and environmental risks, Processes, 2020, 8(11), 1–40 CrossRef.
- I. C. Stanton, A. Bethel, A. F. C. Leonard, W. H. Gaze and R. Garside, Existing evidence on antibiotic resistance exposure and transmission to humans from the environment: a systematic map, Environ Evid., 2022, 11(1), 1–24, DOI:10.1186/s13750-022-00262-2.
- M. Cycoń, A. Mrozik and Z. Piotrowska-Seget, Antibiotics in the soil environment—degradation and their impact on microbial activity and diversity, Front Microbiol., 2019, 10, 338 CrossRef PubMed.
- M. Zhuang, Y. Achmon, Y. Cao, X. Liang, L. Chen and H. Wang,
et al., Distribution of antibiotic resistance genes in the environment, Environ Pollut., 2021, 285, 117402, DOI:10.1016/j.envpol.2021.117402.
- D. G. J. Larsson and C. F. Flach, Antibiotic resistance in the environment, Nat. Rev. Microbiol., 2022, 20(5), 257–269 CrossRef CAS PubMed.
-
World Health Organization, Call to Action on Antimicrobial Resistance (AMR) 2021, 2021 Search PubMed.
- N. Udikovic-Kolic, F. Wichmann, N. A. Broderick and J. Handelsman, Bloom of resident antibiotic-resistant bacteria in soil following manure fertilization, Proc. Natl. Acad. Sci. U. S. A., 2014, 111(42), 15202–15207 CrossRef CAS PubMed.
- I. Michael, L. Rizzo, C. S. McArdell, C. M. Manaia, C. Merlin and T. Schwartz,
et al., Urban wastewater treatment plants as hotspots for the release of antibiotics in the environment: A review, Water Res., 2013, 47(3), 957–995, DOI:10.1016/j.watres.2012.11.027.
-
L. J. O'Neill, Antimicrobial Resistance : Tackling a Crisis for the Health and Wealth of Nations, 2014 Search PubMed.
-
UN Environment, Frontiers 2017 - Emerging Issues of Environmental Concern, United Nations Environment Programme, 2017, pp. 1–22 Search PubMed.
- C. M. Manaia, G. Macedo, D. Fatta-Kassinos and O. C. Nunes, Antibiotic resistance in urban aquatic environments: can it be controlled?, Appl. Microbiol. Biotechnol., 2016, 100(4), 1543–1557 CrossRef CAS PubMed.
- L. Proia, A. Adriana, S. Jessica, B. Carles, F. Marinella and L. Marta,
et al., Antibiotic resistance in urban and hospital wastewaters and their impact on a receiving freshwater ecosystem, Chemosphere, 2018, 206, 70–82 CrossRef PubMed.
- H. Bürgmann, D. Frigon, W. H. Gaze, C. M. Manaia, A. Pruden and A. C. Singer,
et al., Water and sanitation: An essential battlefront in the war on antimicrobial resistance, FEMS Microbiol. Ecol., 2018, 94(9), 1–14 CrossRef PubMed.
-
P. Note, S. Banwart, L. Carter, T. Daniell, Y. Zhu and H. Guo, et al.Expanding the Agricultural – Sanitation Circular Economy : Opportunities and Benefits, 2021, vol. 1–2 Search PubMed.
-
Department for Environment F & RA, British Survey of Fertiliser Practice 2020, 2020 Search PubMed.
-
EMA, Guideline on the Environmental Risk Assessment of Medicinal, 2006, 1–12 Search PubMed.
-
CVMP, Guideline on Environmental Impact Assessment for Veterinary Medicinal Products in Support of VICH Guidelines GL6 and GL38, EMA/CVMP/ERA/418282/2005-Rev.1-Corr. Eur Med Agency, 2016, 44, pp. 1–13, https://www.ema.europa.eu/en/documents/scientific-guideline/guideline-environmental-impact-assessment-veterinary-medicinal-products-support-vich-guidelines-gl6_en.pdf Search PubMed.
- J. P. Sumpter, A. C. Johnson and T. J. Runnalls, Renewing and improving the environmental risk assessment of chemicals, Sci. Total Environ., 2022, 845, 157256, DOI:10.1016/j.scitotenv.2022.157256.
- E. Gullberg, S. Cao, O. G. Berg, C. Ilbäck, L. Sandegren and D. Hughes,
et al., Selection of resistant bacteria at very low antibiotic concentrations, PLoS Pathog., 2011, 7(7), 1–9 Search PubMed.
- S. Chetri, K. Singha, D. Bhowmik, D. Chanda, A. Chakravarty and A. Bhattacharjee, Sub-inhibitory concentration of ertapenem induces overexpression of regulator of antibiotic resistance A in Escherichia coli, Indian J. Med. Microbiol., 2018, 36(4), 569–571, DOI:10.4103/ijmm.IJMM_18_436.
- D. Hughes and D. I. Andersson, Selection of resistance at lethal and non-lethal antibiotic concentrations, Curr. Opin. Microbiol., 2012, 15(5), 555–560, DOI:10.1016/j.mib.2012.07.005.
- J. Bengtsson-Palme and D. G. J. Larsson, Concentrations of antibiotics predicted to select for resistant bacteria: Proposed limits for environmental regulation, Environ. Int., 2016, 86, 140–149 CrossRef CAS PubMed.
- J. Tell, D. J. Caldwell, A. Häner, J. Hellstern, B. Hoeger and R. Journel,
et al., Science-based Targets for Antibiotics in Receiving Waters from Pharmaceutical Manufacturing Operations, Integr. Environ. Assess. Manag., 2019, 15(3), 312–319 CrossRef CAS PubMed.
- A. K. Murray, I. Stanton, W. H. Gaze and J. Snape, Dawning of a new ERA: Environmental Risk Assessment of antibiotics and their potential to select for antimicrobial resistance, Water Res., 2021, 200, 117233, DOI:10.1016/j.watres.2021.117233.
- F. C. T. Elder, K. Proctor, R. Barden, W. H. Gaze, J. Snape and E. J. Feil,
et al., Spatiotemporal profiling of antibiotics and resistance genes in a river catchment: human population as the main driver of antibiotic and antibiotic resistance gene presence in the environment, Water Res., 2021, 117533 CrossRef CAS PubMed.
- K. Kümmerer, Chemosphere Antibiotics in the aquatic environment – A review – Part I, Chemosphere, 2009, 75(4), 417–434, DOI:10.1016/j.chemosphere.2008.11.086.
- E. Peterson and P. Kaur, Antibiotic resistance mechanisms in bacteria: Relationships between resistance determinants of antibiotic producers, environmental bacteria, and clinical pathogens, Front Microbiol., 2018, 9, 1–21 CrossRef PubMed.
- S. T. J. Droge and K. U. Goss, Development and evaluation of a new sorption model for organic cations in soil: Contributions from organic matter and clay minerals, Environ. Sci. Technol., 2013, 47(24), 14233–14241 CrossRef CAS PubMed.
- A. Franco and S. Trapp, Estimation of the soil-water partition coefficient normalized organic carbon for ionizable organic chemicals, Environ. Toxicol. Chem., 2008, 27(10), 1995–2004 CrossRef CAS PubMed.
- L. J. Carter, C. D. Garman, J. Ryan, A. Dowle, E. Bergström and J. Thomas-Oates,
et al., Fate and uptake of pharmaceuticals in soil-earthworm systems, Environ. Sci. Technol., 2014, 48(10), 5955–5963 CrossRef CAS PubMed.
- L. A. Kelly, M. A. Taylor and M. J. A. Wooldridge, Estimating the predicted environmental concentration of the residues of veterinary medicines: Should uncertainty and variability be ignored?, Risk Anal., 2003, 23(3), 489–496 CrossRef PubMed.
-
To Plough or Not to Plough. Tillage Soil Carbon Sequestration, Soil Association, 2018, pp. 5–20 Search PubMed.
- J. Harrower, M. McNaughtan, C. Hunter, R. Hough, Z. Zhang and K. Helwig, Chemical Fate and Partitioning Behavior of Antibiotics in the Aquatic Environment—A Review, Environ. Toxicol. Chem., 2021, 40(12), 3275–3298 CrossRef CAS PubMed.
- D. Zhi, D. Yang, Y. Zheng, Y. Yang, Y. He and L. Luo,
et al., Current progress in the adsorption, transport and biodegradation of antibiotics in soil, J. Environ. Manage., 2019, 251, 109598, DOI:10.1016/j.jenvman.2019.109598.
- S. Wang and H. Wang, Adsorption behavior of antibiotic in soil environment: a critical review, Front. Environ. Sci. Eng., 2015, 9(4), 565–574 CrossRef CAS.
- J. Tolls, Sorption of veterinary pharmaceuticals in soils: A review, Environ. Sci. Technol., 2001, 35(17), 3397–3406 CrossRef CAS PubMed.
- J. Y. Park and B. Huwe, Effect of pH and soil structure on transport of sulfonamide antibiotics in agricultural soils, Environ Pollut., 2016, 213, 561–570, DOI:10.1016/j.envpol.2016.01.089.
- R. A. Figueroa-Diva, D. Vasudevan and A. A. MacKay, Trends in soil sorption coefficients within common antimicrobial families, Chemosphere, 2010, 79(8), 786–793, DOI:10.1016/j.chemosphere.2010.03.017.
- A. J. Carrasquillo, G. L. Bruland, A. A. Mackay and D. Vasudevan, Sorption of ciprofloxacin and oxytetracycline zwitterions to soils and soil minerals: Influence of compound structure, Environ. Sci. Technol., 2008, 42(20), 7634–7642 CrossRef CAS PubMed.
- L. J. Carter, J. L. Wilkinson and A. B. A. Boxall, Evaluation of existing models to estimate sorption coefficients for ionisable pharmaceuticals in soils and sludge, Toxics, 2020, 8(1), 5–7 CrossRef PubMed.
- J. Bengtsson-Palme and D. G. J. Larsson, Concentrations of antibiotics predicted to select for resistant bacteria: Proposed limits for environmental regulation, Environ. Int., 2016, 86, 140–149 CrossRef CAS PubMed.
- J. Huygens, E. Daeseleire, J. Mahillon, D. Van Elst, J. Decrop and J. Meirlaen,
et al., Presence of antibiotic residues and antibiotic resistant bacteria in cattle manure intended for fertilization of agricultural fields: A one health perspective, Antibiotics, 2021, 10(4) DOI:10.3390/antibiotics10040410.
- G. Rasschaert, D. Van Elst, L. Colson, L. Herman, H. C. de C. Ferreira and J. Dewulf,
et al., Antibiotic residues and antibiotic-resistant bacteria in pig slurry used to fertilize agricultural fields, Antibiotics, 2020, 9(1), 34 CrossRef CAS PubMed.
- B. Albero, J. L. Tadeo, M. Escario, E. Miguel and R. A. Pérez, Persistence and availability of veterinary antibiotics in soil and soil-manure systems, Sci. Total Environ., 2018, 643, 1562–1570, DOI:10.1016/j.scitotenv.2018.06.314.
- N. Rakonjac, S. E. A. T. M. van der Zee, L. Wipfler, E. Roex and H. Kros, Emission estimation and prioritization of veterinary pharmaceuticals in manure slurries applied to soil, Sci. Total Environ., 2022, 815 Search PubMed.
- A. De La Torre, I. Iglesias, M. Carballo, P. Ramírez and M. J. Muñoz, An approach for mapping the vulnerability of European Union soils to antibiotic contamination, Sci. Total Environ., 2012, 414, 672–679, DOI:10.1016/j.scitotenv.2011.10.032.
- J. Menz, O. Olsson and K. Kümmerer, Antibiotic residues in livestock manure: Does the EU risk assessment sufficiently protect against microbial toxicity and selection of resistant bacteria in the environment?, J. Hazard Mater., 2019, 379, 120807, DOI:10.1016/j.jhazmat.2019.120807.
- L. L. Li, L. D. Huang, R. S. Chung, K. H. Fok and Y. S. Zhang, Sorption and Dissipation of Tetracyclines in Soils and Compost, Pedosphere, 2010, 20(6), 807–816, DOI:10.1016/S1002-0160(10)60071-9.
- M. Slana and M. S. Dolenc, Environmental Risk Assessment of antimicrobials applied in veterinary medicine-A field study and laboratory approach, Environ. Toxicol. Pharmacol., 2013, 35(1), 131–141, DOI:10.1016/j.etap.2012.11.017.
- P. A. Blackwell, P. Kay and A. B. A. Boxall, The dissipation and transport of veterinary antibiotics in a sandy loam soil, Chemosphere, 2007, 67(2), 292–299 CrossRef CAS PubMed.
- B. J. A. Berendsen, G. Roelofs, B. van Zanten, W. D. M. Driessen-van Lankveld, M. G. Pikkemaat and I. E. A. Bongers,
et al., A strategy to determine the fate of active chemical compounds in soil; applied to antimicrobially active substances, Chemosphere, 2021, 279 Search PubMed.
-
A. Petersons, Ciprofloxacin Tablet Info Sheet. Ciprofloxacin Tablet in for Sheet, 2009, pp. 711–719 Search PubMed.
- A. Barra Caracciolo, P. Grenni, J. Rauseo, N. Ademollo, M. Cardoni and L. Rolando,
et al., Degradation of a fluoroquinolone antibiotic in an urbanized stretch of the River Tiber, Microchem J., 2018, 136, 43–48, DOI:10.1016/j.microc.2016.12.008.
-
C. E. A. Ccinelli, W. I. Oskinen, J. M. B. Ecker and M. J. S. Adowsky, Environmental Fate of Two Sulfonamide Antimicrobial Agents in Soil, 2007, 5 Search PubMed.
- K. Abo El-Sooud, A. Goudah and A. M. Abd El-Aty, Lack of pharmacokinetic interaction between lincomycin and aspirin in healthy goats, J. Vet. Pharmacol. Ther., 2004, 27(5), 389–392 CrossRef CAS PubMed.
- G. M. Cárdenas-Youngs and J. L. Beltrán, Dissociation Constants and Octanol-Water Partition Equilibria for Several Fluoroquinolones, J. Chem. Eng. Data, 2015, 60(11), 3327–3332 CrossRef.
- A. Mehrtens, T. Licha and V. Burke, Occurrence, effects and behaviour of the antibiotic lincomycin in the agricultural and aquatic environment – A review, Sci. Total Environ., 2021, 778, 146306, DOI:10.1016/j.scitotenv.2021.146306.
- Y. Khaledian, E. C. Brevik, P. Pereira, A. Cerdà, M. A. Fattah and H. Tazikeh, Modeling soil cation exchange capacity in multiple countries, Catena, 2017, 158, 194–200, DOI:10.1016/j.catena.2017.07.002.
-
VMD DEFRA, Summary of Product Characteristics Tetracycline, 2018, pp. 4–8 Search PubMed.
-
VMD DEFRA, Summary of Product Characteristic Oxytetracycline, 2020 Search PubMed.
- K. Græsbøll, P. Damborg, A. Mellerup, A. Herrero-Fresno, I. Larsen and A. Holm,
et al., Effect of tetracycline dose and treatment mode on selection of resistant coliform bacteria in nursery pigs, Appl. Environ. Microbiol., 2017, 83(12), 1–12 CrossRef PubMed.
- H. Schmitt, K. Stoob, G. Hamscher, E. Smit and W. Seinen, Tetracyclines and tetracycline resistance in agricultural soils: Microcosm and field studies, Microb. Ecol., 2006, 51(3), 267–276 CrossRef PubMed.
- L. Wu, X. Pan, L. Chen, Y. Huang, Y. Teng and Y. Luo,
et al., Occurrence and distribution of heavy metals and tetracyclines in agricultural soils after typical land use change in east China, Environ. Sci. Pollut. Res., 2013, 20(12), 8342–8354 CrossRef CAS PubMed.
- L. Pan, X. Feng, M. Cao, S. Zhang, Y. Huang and T. Xu,
et al., Determination and distribution of pesticides and antibiotics in agricultural soils from northern China, RSC Adv., 2019, 9(28), 15686–15693 RSC.
-
R. N. Carvalho, L. Ceriani and A. Ippolito, Development of the First Watch List under the Environmental Quality Standards Directive Water Policy, 2008, http://publications.jrc.ec.europa.eu/repository/bitstream/JRC95018/lbna27142enn.pdf Search PubMed.
- Y. Picó and V. Andreu, Fluoroquinolones in soil-risks and challenges, Anal. Bioanal. Chem., 2007, 387(4), 1287–1299 CrossRef PubMed.
- T. Trouchon, S. Lefebvre, T. Trouchon and S. Lefebvre, Review of Enrofloxacin for Veterinary Use, Open J. Vet. Med., 2016, 6(2), 40–58 CrossRef CAS.
- A. Franco, W. Fu and S. Trapp, Influence of soil pH on the sorption of ionizable chemicals: Modeling advances, Environ. Toxicol. Chem., 2009, 28(9), 2018 CrossRef CAS.
- X. Hu, Q. Zhou and Y. Luo, Occurrence and source analysis of typical veterinary antibiotics in manure, soil, vegetables and groundwater from organic vegetable bases, northern China, Environ Pollut., 2010, 158(9), 2992–2998, DOI:10.1016/j.envpol.2010.05.023.
- A. Widyasari-Mehta, S. Hartung and R. Kreuzig, From the application of antibiotics to antibiotic residues in liquid manures and digestates: A screening study in one European center of conventional pig husbandry, J. Environ. Manage., 2016, 177, 129–137, DOI:10.1016/j.jenvman.2016.04.012.
- Y. Deng, B. Li and T. Zhang, Bacteria That Make a Meal of Sulfonamide Antibiotics: Blind Spots and Emerging Opportunities, Environ. Sci. Technol., 2018, 52(7), 3854–3868 CrossRef CAS PubMed.
- S. S. Awaisheh, M. S. Khalifeh, R. J. Rahahleh, J. M. Al-Khaza’Leh and R. M. Algroom, Sulfamethazine contamination level and exposure assessment in domestic and imported poultry meats in Jordan, Vet. World, 2019, 12(12), 1992–1997 CAS.
- Y. B. Ho, M. P. Zakaria, P. A. Latif and N. Saari, Occurrence of veterinary antibiotics and progesterone in broiler manure and agricultural soil in Malaysia, Sci. Total Environ., 2014, 488–489(1), 261–267, DOI:10.1016/j.scitotenv.2014.04.109.
- K. Koutsoumanis, A. Allende, A. Alvarez-Ordóñez, D. Bolton, S. Bover-Cid and M. Chemaly,
et al., Maximum levels of cross-contamination for 24 antimicrobial active substances in non-target feed. Part 11: Sulfonamides, EFSA J., 2021, 19(10) DOI:10.2903/j.efsa.2021.6863.
- J. Li, L. Zhao, M. Feng, C. H. Huang and P. Sun, Abiotic transformation and ecotoxicity change of sulfonamide antibiotics in environmental and water treatment processes: A critical review, Water Res., 2021, 202, 117463, DOI:10.1016/j.watres.2021.117463.
- S. B. Christensen, Drugs that changed society: History and current status of the early antibiotics: Salvarsan, sulfonamides, and β-lactams, Molecules, 2021, 26, 6057 CrossRef CAS PubMed.
- A. G. Mathew, F. Jackson and A. M. Saxton, Effects of antibiotic regimens on resistance of Escherichia coli and Salmonella serovar Typhimurium in swine, J. Swine Health Prod., 2002, 10(1), 7–13 Search PubMed.
- C. M. Schroeder, J. Meng, S. Zhao, C. Debroy, J. Torcolini and C. Zhao,
et al., Antimicrobial Resistance of Escherichia coli O26, O103, O111, O128, 0145 from Animals and Humans, Emerging Infect. Dis., 2002, 8(12), 1409–1414 CrossRef PubMed.
- C. E. Dewey, B. D. Cox, B. E. Straw, E. J. Bush and S. Hurd, Use of antimicrobials in swine feeds in the United States, J. Swine Health Prod., 1999, 7(1), 19–25 Search PubMed.
- S. Kutzner, M. Schaffer, T. Licha, E. Worch and H. Börnick, Sorption of cationic organic substances onto synthetic oxides: Evaluation of sorbent parameters as possible predictors, Sci. Total Environ., 2018, 643, 632–639, DOI:10.1016/j.scitotenv.2018.05.393.
- M. Xu, Y. Xian, J. Wu, Y. Gu, G. Yang and X. Zhang,
et al., Effect of biogas slurry addition on soil properties, yields, and bacterial composition in the rice-rape rotation ecosystem over 3 years, J. Soils Sediments, 2019, 19(5), 2534–2542 CrossRef CAS.
- D. Tian and S. Niu, A global analysis of soil acidification caused by nitrogen addition, Environ. Res. Lett., 2015, 10(2), 024019 CrossRef.
- J. Vestel, D. J. Caldwell, J. Tell, L. Constantine, A. Häner and J. Hellstern,
et al., Default predicted no-effect target concentrations for antibiotics in the absence of data for the protection against antibiotic resistance and environmental toxicity, Integr. Environ. Assess. Manag., 2022, 18(4), 863–867 CrossRef CAS PubMed.
- J. L. Conkle, C. Lattao, J. R. White and R. L. Cook, Competitive sorption and desorption behavior for three fluoroquinolone antibiotics in a wastewater treatment wetland soil, Chemosphere, 2010, 80(11), 1353–1359, DOI:10.1016/j.chemosphere.2010.06.012.
- Z. Schmidtová, R. Kodešová, K. Grabicová, M. Kočárek, M. Fér and H. Švecová,
et al., Competitive and synergic sorption of carbamazepine, citalopram, clindamycin, fexofenadine, irbesartan and sulfamethoxazole in seven soils, J. Contam. Hydrol., 2020, 234, 103680, DOI:10.1016/j.jconhyd.2020.103680.
- J. Li, L. J. Carter and A. B. A. Boxall, Evaluation and development of models for estimating the sorption behaviour of pharmaceuticals in soils, J. Hazard Mater., 2020, 392, 122469, DOI:10.1016/j.jhazmat.2020.122469.
- Y. Chander, K. Kumar, S. M. Goyal and S. C. Gupta, Antibacterial Activity of Soil-Bound Antibiotics, J. Environ. Qual., 2005, 34(6), 1952–1957 CrossRef CAS PubMed.
- M. Subbiah, S. M. Mitchell, J. L. Ullman and D. R. Call, β-Lactams and Florfenicol Antibiotics Remain Bioactive in Soils While Ciprofloxacin, Neomycin, and Tetracycline Are Neutralized, Appl. Environ. Microbiol., 2011, 77(20), 7255–7260 CrossRef CAS PubMed.
- Z. Chen, W. Zhang, G. Wang, Y. Zhang, Y. Gao and S. A. Boyd,
et al., Bioavailability of Soil-Sorbed Tetracycline to Escherichia coli under Unsaturated Conditions, Environ. Sci. Technol., 2017, 51(11), 6165–6173 CrossRef CAS PubMed.
- W. F. Guerin and S. A. Boyd, Differential bioavailability of soil-sorbed naphthalene to two bacterial species, Appl. Environ. Microbiol., 1992, 58(4), 1142–1152 CrossRef CAS PubMed.
- Y. Feng, J. H. Park, T. C. Voice and S. A. Boyd, Bioavailability of soil-sorbed biphenyl to bacteria, Environ. Sci. Technol., 2000, 34(10), 1977–1984 CrossRef CAS.
- Y. Woappi, P. Gabani, A. Singh and O. V. Singh, Antibiotrophs: The complexity of antibiotic-subsisting and antibiotic-resistant microorganisms, Crit. Rev. Microbiol., 2016, 42(1), 17–30 CrossRef CAS PubMed.
- L. Billet, S. Pesce, N. Rouard, A. Spor, L. Paris and M. Leremboure,
et al., Antibiotrophy: Key Function for Antibiotic-Resistant Bacteria to Colonize Soils—Case of Sulfamethazine-Degrading Microbacterium sp. C448, Front Microbiol., 2021, 1–13 Search PubMed.
- W. H. Lewis, G. Tahon, P. Geesink, D. Z. Sousa and T. J. G. Ettema, Innovations to culturing the uncultured microbial majority, Nat. Rev. Microbiol., 2021, 19(4), 225–240, DOI:10.1038/s41579-020-00458-8.
|
This journal is © The Royal Society of Chemistry 2023 |
Click here to see how this site uses Cookies. View our privacy policy here.