DOI:
10.1039/D1TC05966A
(Perspective)
J. Mater. Chem. C, 2022,
10, 2516-2526
Surface adhesion engineering for robust organic semiconductor devices
Received
14th December 2021
, Accepted 14th January 2022
First published on 20th January 2022
Abstract
Devices based on organic semiconductors have been widely used in various applications, for example, organic photovoltaics, organic thermoelectrics, organic solar cells, organic field-effect transistors, and organic sensors. The robust construction of organic semiconductor devices is of vital importance for stable and reliable operation work in real-world environments. The performance and lifespan of organic semiconductor devices, e.g., mechanical and electrical robustness and durability, are normally improved by enhancing the adhesion between the interfaces of organic semiconductors and supporting substrates. In this perspective, we first introduce the basics of surface adhesion from interfacial interactions and characterization methods. Then, diverse strategies for enhancing the interfacial adhesion are summarized, including (i) introducing additional adhesive layers between the interfaces of organic semiconductors and supporting substrates, (ii) functionalizing organic semiconductors with adhesive chemical groups, (iii) integrating adhesive linkers and organic semiconducting units into one chemical entity, and (iv) physically mixing organic semiconductors with various adhesive additives. Mechanisms are further discussed to provide a better understanding of the relationship between molecular interactions and macroscopic adhesion. We also provide a perspective on the up-to-date challenges and future developments in robust organic semiconductor devices.
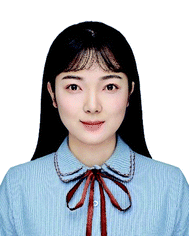
Zhao Wang
| Zhao Wang is currently a PhD candidate at the Technical Institute of Physical Chemistry, Chinese Academy of Sciences (TIPCCAS) and University of Chinese Academy of Sciences (UCAS). She received her BE degree (2016) from Northeast Normal University. Then, she joined TIPCCAS under the supervision of Prof. Shutao Wang. Her research interests include the design and synthesis of smart adhesives and their practical applications. |
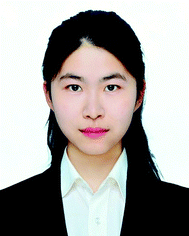
Wenbo Wang
| Wenbo Wang is currently a PhD candidate at the Technical Institute of Physical Chemistry, Chinese Academy of Sciences (TIPCCAS) and University of Chinese Academy of Sciences (UCAS). She received her BE degree (2020) from Northeast Normal University. Then, she joined TIPCCAS under the supervision of Prof. Shutao Wang. Her research interests include the design and synthesis of luminescent adhesives and their applications in electronic devices. |
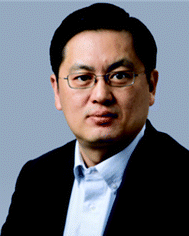
Shutao Wang
| Shutao Wang is a full professor in the Technical Institute of Physics and Chemistry, Chinese Academy of Sciences. He obtained his PhD degree in 2007 at the Institute of Chemistry, Chinese Academy of Sciences (ICCAS). He then worked as a postdoctoral researcher in the Department of Molecular and Medical Pharmacology and California NanoSystem Institute at the University of California in Los Angeles (2007–2010). Subsequently, he was appointed as a full Professor of Chemistry from 2010–2014 at ICCAS. His scientific interests focus on the design and synthesis of bio-inspired interfacial materials with special adhesion and their applications at the nano–biointerface. |
1. Introduction
Organic semiconductors, as a class of electronic materials that feature semiconducting properties and can be used to fabricate semiconductor devices and integrated circuits, have become a research hotspot in the new century.1–6 Compared with inorganic semiconductors, organic semiconductors, including small molecules,7,8 polymers,9–11 and small-molecule:polymer blends,12,13 have obvious advantages in terms of low cost, ease of dissolving, light weight, and low-temperature processing, and have been extensively used in organic solar cells,14–17 organic thermoelectric devices,18–20 organic field-effect transistors,21–24 organic light-emitting diodes,25–28 organic sensors29–32 and so on. More importantly, the solubility, polarity, and electrical properties of organic semiconductors can be feasibly adjusted by tailoring molecular interactions and structures, providing the necessary means to enrich the diversity of materials.33 In the past decades, organic semiconductors have achieved great achievements in multifunctional and advanced device construction.34–36 However, to drive the technology from the lab to actual applications, one of the key challenges that organic semiconductor devices face is the relatively weak stability that requires urgent attention.37–39
High-performance but unstable organic semiconductor devices generally fail to maintain their efficiency and break down within a few minutes to a couple of days.39 Therefore, devices need to be mechanically and electrically robust if they are to work efficiently for decades. Among many factors that affect the stability of organic semiconductor devices,40–43 an important but often overlooked problem is the weak interfacial adhesion between organic semiconductors and supporting substrates. Considering that interface friction, torsion or collision often occurs under long-term work in complex environments, the interfacial adhesion is very critical for the stability of organic semiconductor devices.44 Weak interfacial adhesion can lead to debonding of sensing materials from substrates and thus loss of electrical performance.
Recently, several strategies have been proposed to enhance the interfacial adhesion of organic semiconductors to supporting substrates, including introducing additional adhesive layers between the interfaces of organic semiconductors and supporting substrates45–49 (termed as adhesive layer introduction), functionalizing organic semiconductors with adhesive chemical groups50–53 (termed as adhesive group functionalization), integrating adhesive linkers and organic semiconducting units into one chemical entity (termed as an adhesive integrated agent), and physically mixing organic semiconductors with various adhesive additives54–56 (termed as physical mixing) (Fig. 1). The engineered organic semiconductors based on the above strategies can be used to fabricate robust devices, which show great potential in organic field-effect transistors,57 organic light-emitting diodes,58 chemical sensors,59 temperature sensors,60 magnetic sensors,61 photosensors,62 solar cells,54 flexible transistors63 and so on. However, the interfacial adhesion mechanism and strength characterization of organic semiconductor devices have received very little attention and are still in their infancy.
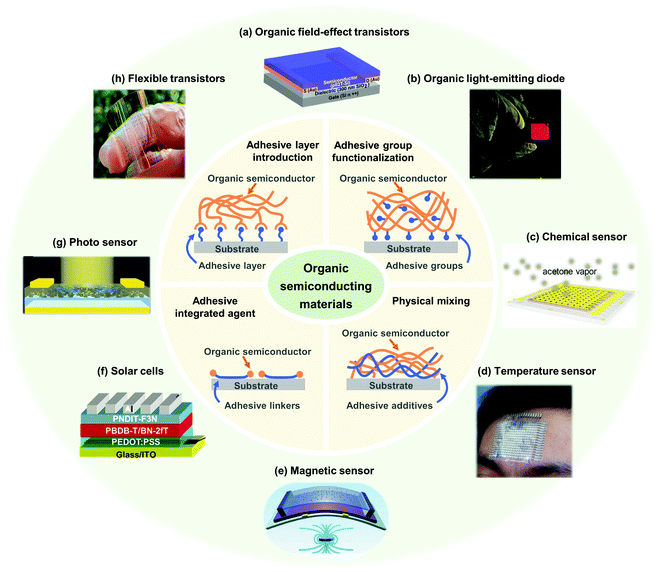 |
| Fig. 1 Schematic diagram of the adhesion enhancing strategies of organic semiconductors to the supporting substrates by introducing additional adhesive layers between interfaces of organic semiconductors and supporting substrates (termed adhesive layer introduction), functionalizing organic semiconductors with adhesive chemical groups (termed adhesive group functionalization), integrating adhesive linkers and organic semiconducting units into one chemical entity (termed adhesive integrated agent), and physically mixing organic semiconductors with various adhesive additives (termed physical mixing). The constructed devices based on the above strategies have shown promising potential in (a) organic field-effect transistors, (b) organic light-emitting diodes, (c) chemical sensors, (d) temperature sensors, (e) magnetic sensors, (f) solar cells, (g) photosensors, (h) flexible transistors and so on. (a–h) Reprinted (adapted) from ref. 57, 58, 59, 60, 61, 54, 62 and 63 with permission from Wiley. | |
In this perspective, we first provide the basics of surface adhesion from interfacial interactions and characterization methods. Then, diverse adhesion engineering strategies of organic semiconductors are summarized. Later, the applications of fabricated robust organic semiconductor devices are briefly introduced. Finally, up-to-date challenges and future developments in robust organic semiconductor devices are proposed and discussed.
2. The basics of surface adhesion
The basic adhesion is presented in this part to facilitate the understanding of the adhesion between the interfaces of organic semiconductors and supporting substrates. Adhesion is a typical physicochemical phenomenon stemming from the same attractive forces between molecules.64 To understand adhesion, we should first figure out the interactions existing between two adhered surfaces and then apply that knowledge to what occurs at surfaces and within interphases.
2.1 Different types of adhesion forces
Adhesion is usually described in terms of forces to separate two adhered materials.65 There are some interaction forces involved in the observed adhesion phenomena such as chemical bonds, Coulomb forces, van der Waals forces, mechanical interlocking, diffusion, suction forces, etc.,66–70 which are schematically shown in Fig. 2a. All of these interactions play significant roles in interfacial bonding.
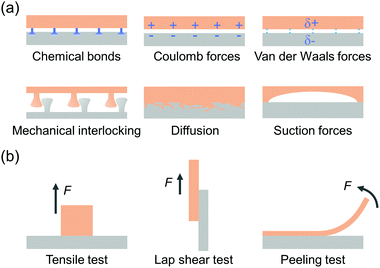 |
| Fig. 2 Adhesion forces and characterization methods. (a) Schematic diagram of diverse adhesion forces existing between the interfaces of two adhered surfaces, including chemical bonds, Coulomb forces, van der Waals forces, mechanical interlocking, diffusion, and suction forces. (b) Characterization methods of adhesion strength between two adhered surfaces through the tensile test, lap shear test, and peeling test. | |
Chemical bonding can take place between two surfaces through the formation of chemical bonds by intermolecular sharing of electron pairs.71,72 Chemical bonds are short-range (0.1–0.2 nm) and very strong (about 100–1000 kJ mol−1) interactions.73,74 Much research has been devoted to the generation of interfacial chemical bonds.75 The formation of chemical bonds between two surfaces requires specific chemical and physical properties of the surfaces. Based on this, the industry has been developed to sell agents that “couple” an organic phase and an inorganic phase, namely, coupling agents.76,77 Coupling agents feature two chemical functional groups, one being reactive with the inorganic phase and the other being reactive with the organic phase, for connecting the two surfaces.
Coulomb forces occur between molecules that have electronegative or electropositive characters.78–80 Surfaces bearing like electrical charges repel each other while surfaces bearing opposite electrical charges attract each other. The Coulomb force is the strongest force of interactions (other than chemical bonds) between molecules. This force has been studied as a potential contributor to adhesion phenomena and can control the adhesive strength between two surfaces but only when substantial differences in electronegativity exist between the materials brought into contact.
Most molecules do not carry electrical charges. In this case, van der Waals force plays a key role in adhesion phenomena because it is always present among all molecules.81,82 The van der Waals interactions generally include dipole–dipole interactions, dipole–induced dipole interactions, and dispersion forces.83 The dispersion force, as a long-range force, exists universally in molecules that have an important and leading role in the study of adhesion.84,85
Mechanical interlocking is another kind of structure-based force in which materials are partially blocked by each other to resist separation.86–88 The interlocking interphase between the two materials will exhibit the so-called “lock and key” effect.89 The key cannot be easily removed from the lock due to the physical impediment provided by the tumblers. Upon separating the two interlocking surfaces, plastic deformation would occur and absorb energy to improve the adhesion bonding.90,91 The effect of surface roughness can increase the plastic deformation of adhesion at the interphase, resulting in an increased adhesion strength.92,93
The diffusion phenomenon of adhesion occurs between two mutually soluble materials when they are brought into close contact.94–96 When the adhesive and adherend are not soluble in one another, a substantial mismatch between the properties of the adhesive and the adherend exists, leading to a stress concentration. The interphase formed in diffusive bonding avoids the stress concentration plane, achieving strong interfacial adhesion.
Suction forces are produced by the creation of reduced pressure in the space between the internal and surrounding pressures.97–99 The plates or hemispheres with elastic edges are usually designed for suction attachment that can conform closely to an adherend surface. The use of suction forces is remarkably common in the most diverse classes of animals. Suction cups serve most commonly for static attachment of an animal to its substrate.
2.2 Adhesion properties characterization methods
It is important to measure the adhesion properties between the interfaces of two adhered surfaces by choosing suitable adhesion characterization methods. Because of the complexity of adhesion phenomena, three typical test methods are usually applied to measure the adhesion performance, which are schematically shown in Fig. 2b. The main difference between these testing methods is how the load is applied to the materials.
The first fundamental measurement is the tensile test in which the applied force is perpendicular to the loading axis until adhesive failure occurs.100 The maximum separation force provides the ultimate interfacial adhesion force. Further tensile strength is obtained by calculating the ratio of the tensile force to the contact area between adhesives and separated substrates. Lap shear testing is another type of adhesion test that applies a lateral shear force to the specimen until failure results.101 Lap shear testing is often carried out to determine the highest shear strength of an adhesive under shear loading. The final measurement used to characterize adhesion property is the peeling test by peeling one end of the sample from the substrate at a certain angle.70,102 This method is intended for flexible adhesives that are not deformed significantly during measurement. These physical measurements provide a lot of adhesive properties and manufacturing processes such as bonding strength, adherence energy, cohesive properties of the interface, bond durability, and other parameters.
3. Chemical strategies to enhance interfacial adhesion of organic semiconductor devices
Based on the basic knowledge of surface adhesion, adhesion strategies for organic semiconductors can be divided into two main categories: chemical strategies and physical mixing. Chemical strategies require the introduction of an additional adhesive layer between the interface of the organic semiconductors and the supporting substrates, the functionalization of organic semiconductors with adhesive chemical groups, or the integration of adhesive linkers and organic semiconductor units into one chemical entity. In this section, recent studies on chemical strategies to enhance interfacial adhesion of organic semiconductor devices are first introduced.
3.1 Adhesive layer introduction
The strong interfacial adhesion of organic semiconductors plays an important role in the development of various electronic devices.103 An effective route to achieve strong interfacial adhesion is the introduction of an adhesive layer (marked in blue) between the interfaces of targeted organic semiconductors and supporting substrates (Fig. 3a). Some specific examples are displayed to explain this approach in Fig. 3b–e. Poly(3,4-ethylenedioxythiophene) (PEDOT), as one of the most promising electrically conducting conjugated polymers, features unique flexibility, high intrinsic electrical conductivity, and ease of processing.104 However, the weak interfacial adhesion of PEDOT to targeted substrates has seriously hampered its practical applications.105 To address this problem, an adhesive layer was introduced into the interfaces between PEDOT and targeted substrates. As shown in Fig. 3b, the plastic/glass substrates were firstly modified with di-Si(OEt)3 functionalized free radical initiators (AIBN).46 And then, 3,4-(vinylenedioxy)thiophene (VDOT) with isolated double bonds was attached to the targeted substrate by the radical addition reaction between vinylenedioxy groups and AIBN on the substrate to act as an adhesive layer (marked in blue). Subsequently, poly(3,4-ethylenedioxythiophene) (PEDOT) was connected with VDOT by chemical polymerization, eventually achieving strong adhesion of PEDOT to substrates owing to the formation of covalent bonds. The adhesion strength was measured using the tape test by drawing 100 squares on the film with sharp objects and counting the number of squares that survived the test. The results showed that all 100 squares remained intact on the AIBN-modified substrate, while no squares were present on the surface of the control film made using the initiator-free substrate. The robust adhesion of the PEDOT membrane is valuable for prolonging the service life of antistatic coatings. Besides, 2,3-dihydrothieno(3,4b)(1,4)dioxine-2-carboxylic acid (EDOT-acid) was also introduced as an interfacial adhesive monolayer to improve the weak adhesion of PEDOT to substrates (Fig. 3c).47 As indicated in the figure, EDOT-acid was first attached to indium tin oxide by the chemisorption interactions between the carboxyl groups of EDOT-acid and M+ of the ITO electrode to act as an adhesive layer. Subsequently, PEDOT was polymerized onto the EDOT acid-modified substrate to form a strong attachment to the ITO substrates. Ultrasonic experiments demonstrated that the PEDOT films coated onto EDOT acid-modified ITO remained intact even after 2 min of sonication; in contrast, the PEDOT films on unmodified ITO electrodes could only sustain up to 5 s of sonication. This indirectly indicated that the surface treated with EDOT-acid exhibited stronger adhesion than untreated substrates.
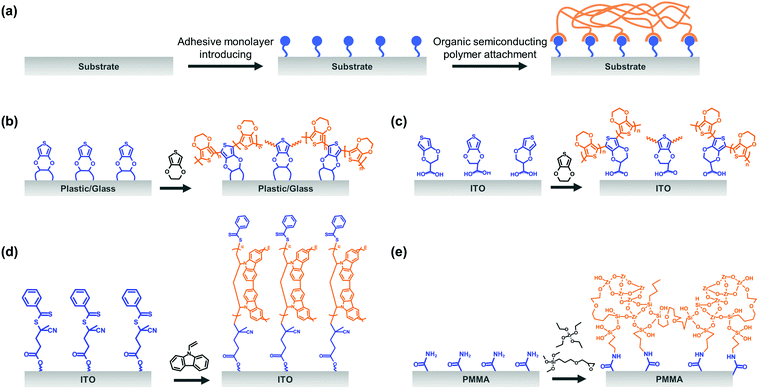 |
| Fig. 3 Introducing adhesive layers to achieve the strong interfacial adhesion of organic semiconductors to the supporting substrates. (a) A flow chart showing the process of introducing an adhesive layer and covalently bonding of organic semiconductors to targeted substrates. (b) Covalently bonding PEDOT on a VDOT modified substrate to achieve strong interfacial adhesion. (c) Introducing EDOT-acid as an adhesion-enhanced layer for the polymerization of PEDOT onto the substrate to form a strongly bonded coating. (d) Covalently connected poly(N-vinylcarbazole) (PVK) on an ITO surface modified with a CTA adhesive layer. (e) A tough adhesion of the deposited coating by the covalent cross-linking reaction between amino groups on substrates and epoxy or alcohol groups on semiconductors. | |
To achieve strong adhesion of N-vinylcarbazole (PVK) to an ITO substrate, a reversible addition–fragmentation chain transfer (RAFT) agent (CBz-CTA) was firstly electro-deposited onto the ITO surface to serve as an adhesive layer (Fig. 3d).45 And then, PVK was polymerized on the CTA-modified surface using the surface-initiated RAFT method. This method provides an effective way to create a strong attachment between PVK and the ITO substrate by chemical bonding. The strong adhesion has been testified by no damage of the PVK layer after over one hour of ultrasonic treatment. The covalent-bonded PVK was used as the hole transport layer in organic photovoltaic devices, thereby guaranteeing stable electrical conductivity of the devices. To realize strong adhesion of tetraethyl orthosilicate coatings to the PMMA substrate, the PMMA surface was pre-treated with (3-aminopropyl)triethoxysilane (APTES) to obtain an amino-modified surface (Fig. 3e).48 These amino groups reacted with epoxy or alcohol groups of (3-glycidyloxypropyl)trimethoxysilane (GPTMS) to form a tough covalent cross-linking. APTES molecules act as a molecular bridge to promote the strong adhesion of the deposited coatings to the PMMA substrate. After surface treatment using the APTES coupling agent, the adhesion energy increased from ca. 26 to ca. 54 J m−2.
In addition to the introduction of the adhesive monolayers, a nano-layer of adhesive polymers could also be introduced as the interfacial connection between organic semiconductors and supporting substrates.106 As shown in Fig. 4, the adhesive nano-layer of polyurethane/polyacrylate can be applied to different amino-functionalized substrates by various coating methods such as spin coating, spray coating, and dip coating. Subsequently, the conducting polymers are applied to the adhesive nano-layer coated substrates by solvent-casting or electrodeposition. An interpenetrating polymer network is formed between the conductive polymers and the adhesive layer, eventually resulting in robust interfacial adhesion. Through lap shear testing, an amine-functionalized glass substrate with a PU adhesive layer (160 kPa) provides a higher shear strength, nearly three times that of the pristine glass substrate with a PU adhesive layer. This work provides a general strategy to enhance the interfacial adhesion of organic semiconductors.
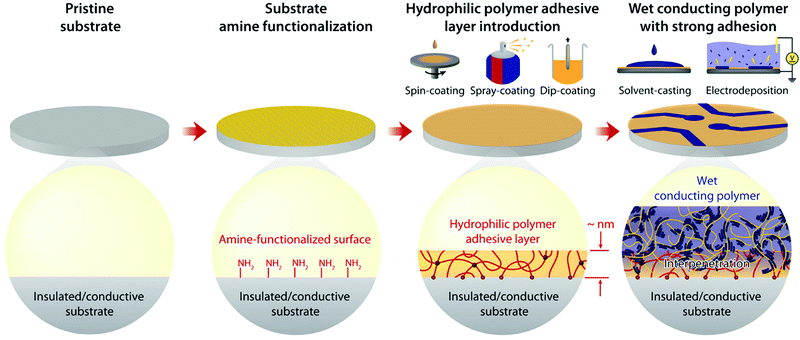 |
| Fig. 4 Schematic diagram of constructing robust conducting polymer films on insulated/conductive substrates via the introduction of hydrophilic adhesive nano-layers. The substrate was firstly functionalized with amino groups, and then a hydrophilic polymer adhesive nano-layer was coated on the amino-functionalized substrate. The formation of an interpenetrating network between the conducting polymer and the adhesive nano-layer results in the strong attachment of organic semiconductors to targeted substrates. Reprinted from ref. 106 with permission from 2020 Science. | |
The introduction of adhesive layers between the interfaces of organic semiconductors and targeted substrates can achieve strong interfacial adhesion through covalent bonds or physical interactions. However, this method inevitably requires pre-treatment of the substrate surface with active groups or polymers, which is relatively complex and limited to specific substrates.
3.2 Adhesive group functionalization
Another chemical strategy to enhance the interfacial adhesion of organic semiconductors to targeted substrates is achieved by functionalizing them with adhesive chemical groups, which are marked in blue color (Fig. 5a). The designed organic semiconductors can form strong adhesion with the supporting substrates. Compared to the strategy of introducing adhesive layers, this method is more convenient and does not need pre-treatment of the substrate. The most important step of this approach is the selection of adhesive groups. In recent years, catechol and its derivatives have gained a lot of attention due to their dramatic interfacial adhesion strength,107 which are often used to modify molecules or polymers.108–110 As indicated in Fig. 5b, poly(N-methacryloyl-3,4-dihydroxyl-L-phenylalanineco-3-sulfopropyl methacrylate) (PSS) containing adhesive catechol groups was oxidatively polymerized with conductive poly(3,4-ethylenedioxythiophene) (PEDOT) to obtain a fully organic polymer adhesive with a high electrical conductivity, namely PMS:PEDOT.52 PMS:PEDOT demonstrated an adhesion strength of 2.53 × 10−1 MPa, which is 6.4 times higher than that of PMTS:PEDOT. It is supposed that the dihydroxy groups of catechol (hydrogen bond donor) can be bonded to the surface of polyester (ester group-hydrogen bond acceptor) through hydrogen bonds. Besides, benzene rings of catechol bind to the aryl groups on the PET main chains via π–π interactions. In Fig. 5c, catechol can also be grafted onto macromolecule polypyrrole (PPy) to serve as side chain groups, which could endow PPy with enhanced adhesive properties.103 Only a small amount of catechol derivatives can dramatically improve the adhesion of PPy, which is about three orders of magnitude higher than that of pure PPy. The synthesized conductive materials can firmly adhere to the surface, which could be widely used in the manufacture of biosensors and electronic devices.
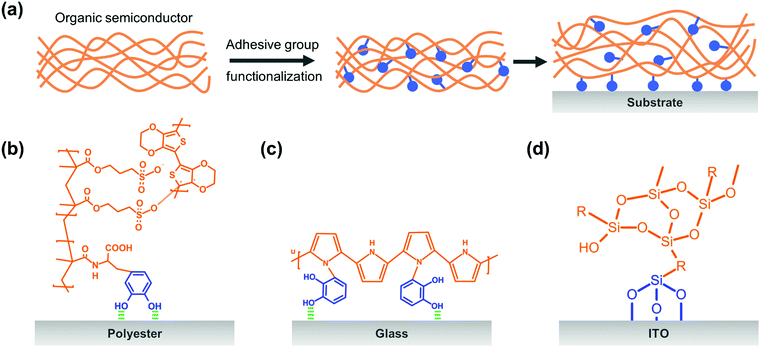 |
| Fig. 5 Direct modification of organic semiconductors with adhesive chemical groups. (a) A schematic diagram showing the process of functionalizing organic semiconductors with adhesive chemical groups to achieve strong adhesion to the supporting substrates. (b) Strong adhesion of organic conductive poly(N-methacryloyl-3,4-dihydroxyl-L-phenylalanineco-3-sulfopropyl methacrylate):poly(3,4-ethylenedioxythiophene) (PMS:PEDOT) with adhesive catechol groups to the polyester substrate. (c) Polypyrrole functionalized by catechol groups can achieve strong interfacial adhesion to glass. (d) Strong adhesion of semiconducting polymers on the ITO substrate by the covalent bonding between trimethoxysilane groups and hydroxyl groups on the substrate surface. | |
Although catechol groups can improve the interfacial adhesion of organic semiconductors to targeted substrates by the effective phenolic hydroxyl, the non-covalent and unstable interactions with the substrate cannot meet the demand for harsh conditions. Therefore, covalent-bonded siloxane groups have aroused broad attention for enhancing the interfacial adhesion of organic semiconductors. The formation of silicon–oxygen bonds between siloxane groups and the substrate surface provides a stronger interfacial adhesion in comparison with non-covalent interactions. As shown in Fig. 5d, the surface of the substrate was hydroxylated by a short-time oxygen plasma pre-treatment before the imprinting process. Then, the siloxane-derived tetraphenylbenzidine (DTMSTPD) modified with trimethoxysilane groups can covalently bond to the ITO substrate by reacting with the hydroxyl groups on the surface, achieving robust interfacial adhesion.50
3.3 Adhesive integrated agent
Most recently, Wang and his co-workers demonstrated a new molecular engineering strategy, namely, the adhesive-integrated-agent (AIA) strategy, to enhance the interfacial adhesion of organic semiconducting molecules to the supporting substrates.111 This AIA strategy is based on integrating the adhesive linkers with the semiconducting units into one chemical entity. The adhesive linker connects the two organic semiconducting units that are not much altered about their initial chemical structures, to exert the effects of each unit. As shown in Fig. 6a, gas-sensitive PDMS-Pt-L nanobelt arrays with extremely robust interfacial adhesion were constructed. The highly adhesive PDMS-Pt-L molecules were composed of gas-sensitive organoplatinum(II) units at two terminals linked by the adhesive PDMS backbones. The integration of polar organoplatinum(II) moieties and hydrophobic poly(dimethylsiloxane) backbones into one molecule can form ordered packing structures of PDMS-Pt-L to endow the fabricated nanobelt arrays with excellent adhesion and gas-sensing functions. The ultrahigh shear strength of the PDMS-Pt-L nanoribbon array is about 7.05 × 106 N m−2, and the tensile strength is about 5.07 × 106 N m−2, which is almost 5 times that of the Pt-L nanoribbon array, revealing the formation of tough adhesion between the PDMS-Pt-L nanoribbon array and the substrate. As excepted, the adhesion strength of PDMS-Pt-L arrays was higher than that of Pt-L arrays in both shear and tensile adhesion tests (Fig. 6b). Besides, PDMS-Pt-L arrays can be fabricated on flexible substrates to extend their application areas to wearable devices (Fig. 6c and d). The fabricated PDMS-Pt-L nanobelts exhibited robust adhesion and sensing performance even under aggressive ultrasonication, tape peeling, or repeated bending. This work offers a general paradigm to construct robust devices that can be further extended to other molecule-based devices.
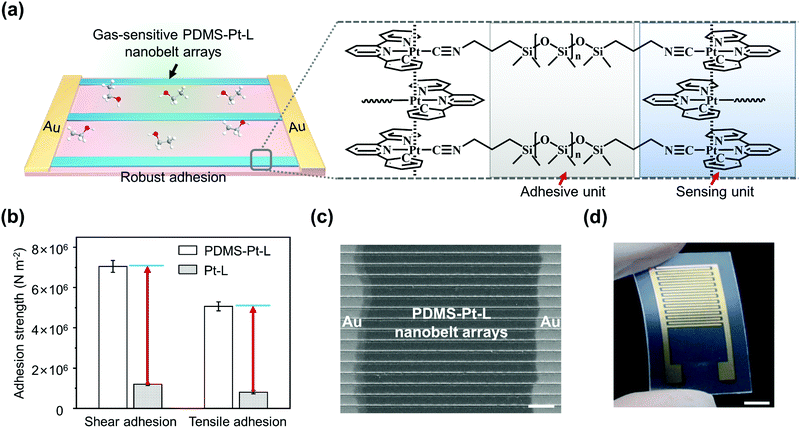 |
| Fig. 6 A bioinspired adhesive-integrated-agent strategy to design robust PDMS-Pt-L sensing arrays. (a) Schematic diagram showing the fabrication of robust gas-sensitive PDMS-Pt-L nanobelt arrays comprising adhesive units and organic sensing units in one chemical entity. (b) The adhesion strength of PDMS-Pt-L arrays is higher than that of Pt-L arrays in both shear and tensile tests. (c) The SEM image (scale bar: 30 μm) and (d) photograph (scale bar: 0.25 cm) of the wearable PDMS-Pt-L sensor. Reprinted from ref. 111 with permission from Wiley. | |
4. Physical mixing to enhance the interfacial adhesion of organic semiconductor devices
Physical mixing is another adhesion-enhancing strategy commonly used in the field of organic cell devices to enhance interfacial adhesion by simply mixing organic semiconductors with various adhesive additives (Fig. 7). To provide perovskite-based devices with mechanical adhesion and protection, a robust and highly conductive laminate electrode was designed by mixing a polymer embedded Ni mesh electrode with a silver-free transparent conducting adhesive (TCA).112 TCA was prepared by blending the conducting poly(3,4-ethylenedioxythiophene):poly(styrenesulfonate) (PEDOT:PSS) with a pressure-sensitive acrylic adhesive to achieve conduction between the perovskite and the Ni counter electrode wires. The TCA-laminate provides a simple alternative to the gold evaporated contact, which can be used in the perovskite cell mounted on conducting glass or in a reverse setup. In addition to mixing with acrylic adhesives, PEDOT:PSS can also be blended with polyethylene glycol adhesive additives to prepare a conductive paste.113 Then, a counter electrode for solar cells was prepared by coating the highly transparent and adhesive PEDOT:PSS on the FTO glass.
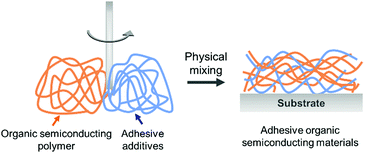 |
| Fig. 7 Physical mixing strategy for enhancing the interfacial adhesion of organic semiconductor devices. The organic semiconductors can be mixed with various adhesive additives to fabricate organic semiconducting materials. | |
Compared with chemical strategies, the physical mixing method is relatively simple for enhancing the interfacial adhesion of organic semiconductor devices without the need for elaborate molecule design and synthesis. Physical mixing can improve the mechanical and processing performance of organic semiconductors, reduce costs and expand the scope of use. However, compatibility is the basic condition of physical mixing to avoid macrophase separation. The good compatibility between the two phases is the premise of the good properties (especially mechanical and electrical properties) of the two-phase system blended products. The adhesive additives and organic semiconductors with good compatibility can more easily disperse during the blending process for beneficial effects on adhesive and electrical performance.
5. Conclusions
In this perspective, we have introduced the basics of surface adhesion and summarized diverse adhesion enhancing strategies for organic semiconductor devices, including adhesive layer introduction, adhesive group functionalization, adhesive integrated agent, and physical mixing with adhesive additives. The adhesive layer introducing strategy is often used to enhance the interfacial adhesion via introducing adhesive molecular bridges, such as 3,4-(vinylenedioxy)thiophene, EDOT-acid, (3-aminopropyl)triethoxysilane, and primary amine groups between organic semiconductors and supporting substrates. This method requires the pre-treatment of targeted substrates with active chemical groups or polymers. To avoid the complex pre-treatment of targeted substrates, the adhesive group functionalization method is further developed to improve the interfacial adhesion of organic semiconductor devices. The adhesive groups, including trimethoxysilane, aldehyde, and catechol molecules, can form covalent bonding or physical interactions with substrate surfaces. Besides, the recently developed AIA strategy achieved by integrating adhesive linkers and organic semiconducting units into one chemical entity has greatly improved the interfacial adhesion of organic devices. Besides the above-mentioned chemical strategies, mixing organic semiconductors with adhesive additives, as a physical adhesion enhancing strategy, is relatively simple because there is no need for substrate pre-treatment or organic semiconductor modification. However, the electrical performance of organic semiconductors might have been attenuated due to the introduction of insulating adhesive additives.
Although some attempts have been successfully made in developing robust organic semiconductor devices, the issues regarding device mechanical stability remain largely unexplored. It is well known that a large number of organic semiconducting molecules have been discovered to date. The weak interfacial adhesion of these organic semiconductor devices remains to be addressed. Moreover, the adhesion strength of organic semiconductor devices was often qualitatively characterized by ultrasonication,45,47 tape peeling,111 repeated bending,111 and so on. The quantitative characterization method of interfacial adhesion strength has remained underdeveloped. Further studies will be necessary to develop new adhesion characterization means for specific adhesive modes and general strategies for diverse materials and applications.
Conflicts of interest
The authors declare no competing financial interest.
Acknowledgements
The work is supported by the National Natural Science Foundation of China (No. 22035008, 21972155, and 21988102) and the Key Research Program of the Chinese Academy of Sciences (XDPB24).
References
- J. E. Anthony, A. Facchetti, M. Heeney, S. R. Marder and X. Zhan, Adv. Mater., 2010, 22, 3876–3892 CrossRef CAS PubMed.
- C. Wang, H. Dong, L. Jiang and W. Hu, Chem. Soc. Rev., 2018, 47, 422–500 RSC.
- S. E. Root, S. Savagatrup, A. D. Printz, D. Rodriquez and D. J. Lipomi, Chem. Rev., 2017, 117, 6467–6499 CrossRef CAS PubMed.
- C. Wang, H. Dong, L. Jiang and W. Hu, Chem. Soc. Rev., 2018, 47, 422–500 RSC.
- Y. Huang, D. L. Elder, A. L. Kwiram, S. A. Jenekhe, A. K. Y. Jen, L. R. Dalton and C. K. Luscombe, Adv. Mater., 2021, 33, e1904239 CrossRef PubMed.
- J. E. Anthony, Angew. Chem., Int. Ed., 2008, 47, 452–483 CrossRef CAS PubMed.
- Y. Z. Lin, Y. F. Li and X. W. Zhan, Chem. Soc. Rev., 2012, 41, 4245–4272 RSC.
- H. E. Katz, Z. N. Bao and S. L. Gilat, Acc. Chem. Res., 2001, 34, 359–369 CrossRef CAS PubMed.
- A. Pron and P. Rannou, Prog. Polym. Sci., 2002, 27, 135–190 CrossRef CAS.
- Y. Yao, H. Dong and W. Hu, Adv. Mater., 2016, 28, 4513–4523 CrossRef CAS PubMed.
- H. L. Pan, Y. N. Li, Y. L. Wu, P. Liu, B. S. Ong, S. P. Zhu and G. Xu, J. Am. Chem. Soc., 2007, 129, 4112–4113 CrossRef CAS PubMed.
- L.-H. Chou, Y. Na, C.-H. Park, M. S. Park, I. Osaka, F. S. Kim and C.-L. Liu, Polymer, 2020, 191, 122208 CrossRef CAS.
- J. Kang, N. Shin, D. Y. Jang, V. M. Prabhu and D. Y. Yoon, J. Am. Chem. Soc., 2008, 130, 12273–12275 CrossRef CAS PubMed.
- Y. Gao, Y. Wu, Y. Liu, M. Lu, L. Yang, Y. Wang, W. W. Yu, X. Bai, Y. Zhang and Q. Dai, Nanoscale Horiz., 2020, 5, 1574–1585 RSC.
- A. W. Hains, Z. Liang, M. A. Woodhouse and B. A. Gregg, Chem. Rev., 2010, 110, 6689–6735 CrossRef CAS PubMed.
- Y. Lin, J. Wang, Z.-G. Zhang, H. Bai, Y. Li, D. Zhu and X. Zhan, Adv. Mater., 2015, 27, 1170–1174 CrossRef CAS PubMed.
- Y. Lin, F. Zhao, Q. He, L. Huo, Y. Wu, T. C. Parker, W. Ma, Y. Sun, C. Wang, D. Zhu, A. J. Heeger, S. R. Marder and X. Zhan, J. Am. Chem. Soc., 2016, 138, 4955–4961 CrossRef CAS PubMed.
- Y. Sun, L. Qiu, L. Tang, H. Geng, H. Wang, F. Zhang, D. Huang, W. Xu, P. Yue, Y.-S. Guan, F. Jiao, Y. Sun, D. Tang, C.-A. Di, Y. Yi and D. Zhu, Adv. Mater., 2016, 28, 3351–3358 CrossRef CAS PubMed.
- J. Ding, Z. Liu, W. Zhao, W. Jin, L. Xiang, Z. Wang, Y. Zeng, Y. Zou, F. Zhang, Y. Yi, Y. Diao, C. R. McNeill, C.-A. Di, D. Zhang and D. Zhu, Angew. Chem., Int. Ed., 2019, 58, 18994–18999 CrossRef CAS PubMed.
- X. Dai, Q. Meng, F. Zhang, Y. Zou, C.-A. Di and D. Zhu, J. Energy Chem., 2021, 62, 204–219 CrossRef.
- J. Kim, S. H. Kim, T. K. An, S. Park and C. E. Park, J. Mater. Chem. C, 2013, 1, 1272–1278 RSC.
- H. Sirringhaus, Adv. Mater., 2014, 26, 1319–1335 CrossRef CAS PubMed.
- D. Khim, A. Luzio, G. E. Bonacchini, G. Pace, M. J. Lee, Y. Y. Noh and M. Caironi, Adv. Mater., 2018, 30, e1705463 CrossRef PubMed.
- C. Wang, H. Dong, W. Hu, Y. Liu and D. Zhu, Chem. Rev., 2012, 112, 2208–2267 CrossRef CAS PubMed.
- X. Y. Cai and S. J. Su, Adv. Funct. Mater., 2018, 28, 1802558 CrossRef.
- M. Shibata, Y. Sakai and D. Yokoyama, J. Mater. Chem. C, 2015, 3, 11178–11191 RSC.
- Y. Chen and D. Ma, J. Mater. Chem., 2012, 22, 18718–18734 RSC.
- Y. Han, L. Bai, J. Lin, X. Ding, L. Xie and W. Huang, Adv. Funct. Mater., 2021, 31, 2105092 CrossRef CAS.
- C. Yu, J. H. He, X. F. Cheng, H. Z. Lin, H. T. Yu and J. M. Lu, Angew. Chem., Int. Ed., 2021, 60, 15328–15334 CrossRef CAS PubMed.
- Y. Zang, D. Huang, C. A. Di and D. Zhu, Adv. Mater., 2016, 28, 4549–4555 CrossRef CAS PubMed.
- K. Potje-Kamloth, Crit. Rev. Anal. Chem., 2002, 32, 121–140 CrossRef CAS.
- Y. Zang, F. Zhang, C.-A. Di and D. Zhu, Mater. Horiz., 2015, 2, 140–156 RSC.
- H. E. Katz, Z. Bao and S. L. Gilat, Acc. Chem. Res., 2001, 34, 359–369 CrossRef CAS PubMed.
- M. Eslamian, F. Mirab, V. K. Raghunathan, S. Majd and M. R. Abidian, Adv. Funct. Mater., 2021, 31, 2105358 CrossRef CAS PubMed.
- L. Yao, A. Rahmanudin, N. Guijarro and K. Sivula, Adv. Energy Mater., 2018, 8, 1802585 CrossRef.
- Y. Qian, X. Zhang, L. Xie, D. Qi, B. K. Chandran, X. Chen and W. Huang, Adv. Mater., 2016, 28, 9243–9265 CrossRef CAS PubMed.
- H.-N. Yang, S.-J. He, T. Zhang, J.-X. Man, Y. Zhao, N. Jiang, D.-K. Wang and Z.-H. Lu, Org. Electron., 2021, 88, 106014 CrossRef CAS.
- A. Giovannitti, C. B. Nielsen, D.-T. Sbircea, S. Inal, M. Donahue, M. R. Niazi, D. A. Hanifi, A. Amassian, G. G. Malliaras, J. Rivnay and I. McCulloch, Nat. Commun., 2016, 7, 13066 CrossRef CAS PubMed.
-
A. Dey, A. Singh, D. Das and P. K. Iyer, Organic Semiconductors: A New Future of Nanodevices and Applications, in Thin Film Structures in Energy Applications, ed. S. Babu Krishna Moorthy, Springer, Cham., 2015, pp. 97–128 Search PubMed.
- M. A. Fusella, R. Saramak, R. Bushati, V. M. Menon, M. S. Weaver, N. J. Thompson and J. J. Brown, Nature, 2020, 585, 379–382 CrossRef CAS PubMed.
- H. Yu and H. Aziz, Adv. Opt. Mater., 2019, 7, 1800923 CrossRef.
- D. H. Lee, H. D. Yun, E. D. Jung, J. H. Chu, Y. S. Nam, S. Song, S.-H. Seok, M. H. Song and S.-Y. Kwon, ACS Appl. Mater. Interfaces, 2019, 11, 21069–21077 CrossRef CAS PubMed.
- Y.-C. Chao, Y.-H. Liao, H.-L. Hsu, B.-H. Jiang, J.-C. Kao, T.-H. Lai, C.-P. Chen and R.-J. Jeng, ACS Appl. Energy Mater., 2019, 2, 833–843 CrossRef CAS.
- Z. Dai, S. K. Yadavalli, M. Chen, A. Abbaspourtamijani, Y. Qi and N. P. Padture, Science, 2021, 372, 618–622 CrossRef CAS PubMed.
- M. C. Tria, K.-S. Liao, N. Alley, S. Curran and R. Advincula, J. Mater. Chem., 2011, 21, 10261–10264 RSC.
- A. G. Sadekar, D. Mohite, S. Mulik, N. Chandrasekaran, C. Sotiriou-Leventis and N. Leventis, J. Mater. Chem., 2012, 22, 100–108 RSC.
- B. Wei, J. Liu, L. Ouyang, C. C. Kuo and D. C. Martin, ACS Appl. Mater. Interfaces, 2015, 7, 15388–15394 CrossRef CAS PubMed.
- Y. Ding, S. Dong, J. Han, D. He, Z. Zhao and R. H. Dauskardt, Adv. Mater. Interfaces, 2018, 5, 1701433 CrossRef.
- J. H. Yun, I. Lee, T.-S. Kim, M. J. Ko, J. Y. Kim and H. J. Son, J. Mater. Chem. A, 2015, 3, 22176–22182 RSC.
- N. Haberkorn, S. A. L. Weber, R. Berger and P. Theato, ACS Appl. Mater. Interfaces, 2010, 2, 1573–1580 CrossRef CAS PubMed.
- W. Zhang, Z. H. Pan, F. K. Yang and B. X. Zhao, Adv. Funct. Mater., 2015, 25, 1588–1597 CrossRef CAS.
- M. Kim, M. F. Butler, I. Pramudya, C. Lee, S. Kim and H. Chung, Chem. Mater., 2019, 31, 8358–8365 CrossRef CAS.
- E. Istif, D. Mantione, L. Vallan, G. Hadziioannou, C. Brochon, E. Cloutet and E. Pavlopoulou, ACS Appl. Mater. Interfaces, 2020, 12, 8695–8703 CrossRef CAS PubMed.
- Y. Li, H. Meng, T. Liu, Y. Xiao, Z. Tang, B. Pang, Y. Li, Y. Xiang, G. Zhang, X. Lu, G. Yu, H. Yan, C. Zhan, J. Huang and J. Yao, Adv. Mater., 2019, 31, 1904585 CrossRef CAS PubMed.
- G. Yue, J. Wu, Y. Xiao, J. Lin, M. Huang, L. Fan and Y. Yao, Chin. Sci. Bull., 2013, 58, 559–566 CrossRef CAS.
- L. Migliaccio, D. Altamura, F. Scattarella, C. Giannini, P. Manini, F. Gesuele, M. G. Maglione, P. Tassini and A. Pezzella, Adv. Electron. Mater., 2019, 5, 1800585 CrossRef.
- M. L. Hammock, O. Knopfmacher, T. N. Ng, J. B. Tok and Z. Bao, Adv. Mater., 2014, 26, 6138–6144 CrossRef CAS PubMed.
- D. Meng, R. Wang, J. B. Lin, J. L. Yang, S. Nuryyeva, Y. C. Lin, S. Yuan, Z. K. Wang, E. Zhang, C. Xiao, D. Zhu, L. Jiang, Y. Zhao, Z. Li, C. Zhu, K. N. Houk and Y. Yang, Adv. Mater., 2021, 33, e2006120 CrossRef PubMed.
- E. K. Lee, C. H. Park, J. Lee, H. R. Lee, C. Yang and J. H. Oh, Adv. Mater., 2017, 29, 1605282 CrossRef PubMed.
- X. Ren, K. Pei, B. Peng, Z. Zhang, Z. Wang, X. Wang and P. K. Chan, Adv. Mater., 2016, 28, 4832–4838 CrossRef CAS PubMed.
- Y. Zang, F. Zhang, D. Huang, C. A. Di and D. Zhu, Adv. Mater., 2015, 27, 7979–7985 CrossRef CAS PubMed.
- X. Wu, S. Mao, J. Chen and J. Huang, Adv. Mater., 2018, 30, e1705642 CrossRef PubMed.
- W. Zhao, J. Jie, Q. Wei, Z. Lu, R. Jia, W. Deng, X. Zhang and X. Zhang, Adv. Funct. Mater., 2019, 29, 1902494 CrossRef.
- A. Baldan, Int. J. Adhes. Adhes., 2012, 38, 95–116 CrossRef CAS.
- S. G. Croll, Prog. Org. Coat., 2020, 148, 105847 CrossRef CAS.
- D. Tabor, Abstr. Pap. Am. Chem. Soc., 1974, 16 Search PubMed.
- X. Jin, L. Heepe, J. Strueben, R. Adelung, S. N. Gorb and A. Staubitz, Macromol. Rapid Commun., 2014, 35, 1551–1570 CrossRef CAS PubMed.
- W. Zhang, R. Wang, Z. Sun, X. Zhu, Q. Zhao, T. Zhang, A. Cholewinski, F. Yang, B. Zhao, R. Pinnaratip, P. K. Forooshani and B. P. Lee, Chem. Soc. Rev., 2020, 49, 433–464 RSC.
- C. Cui and W. Liu, Prog. Polym. Sci., 2021, 116, 101388 CrossRef CAS.
- Z. Gu, S. Li, F. Zhang and S. Wang, Adv. Sci., 2016, 3, 1500327 CrossRef PubMed.
- B. Silvi and A. Savin, Nature, 1994, 371, 683–686 CrossRef CAS.
- R. J. Gillespie and E. A. Robinson, J. Comput. Chem., 2007, 28, 87–97 CrossRef CAS PubMed.
- S. B. Zhu and M. R. Philpott, J. Chem. Phys., 1994, 100, 6961–6968 CrossRef CAS.
- M. A. Lantz, H. J. Hug, R. Hoffmann, P. J. A. van Schendel, P. Kappenberger, S. Martin, A. Baratoff and H. J. Guntherodt, Science, 2001, 291, 2580–2583 CrossRef CAS PubMed.
- W. Xu, W. Tian, L. Meng, F. Cao and L. Li, Adv. Energy Mater., 2021, 11, 2003500 CrossRef CAS.
- C. Sanchez, P. Belleville, M. Popall and L. Nicole, Chem. Soc. Rev., 2011, 40, 696–753 RSC.
- S. Sterman and J. G. Marsden, Ind. Eng. Chem., 1966, 58, 33 CrossRef CAS.
- I. Langmuir, Science, 1921, 54, 59–67 CrossRef CAS PubMed.
- R. G. Horn and D. T. Smith, Science, 1992, 256, 362–364 CrossRef CAS.
- R. G. Horn, D. T. Smith and A. Grabbe, Nature, 1993, 366, 442–443 CrossRef CAS.
- Y. Andersson, D. C. Langreth and B. I. Lundqvist, Phys. Rev. Lett., 1996, 76, 102–105 CrossRef CAS PubMed.
- F. London, Trans. Faraday Soc., 1937, 33, 8–26 RSC.
- X. Du, Y. Li, Y.-L. Xia, S.-M. Ai, J. Liang, P. Sang, X.-L. Ji and S.-Q. Liu, Int. J. Mol. Sci., 2016, 17, 144 CrossRef PubMed.
- E. R. Johnson and G. A. DiLabio, Chem. Phys. Lett., 2006, 419, 333–339 CrossRef CAS.
- D. Li, J. Chun, D. Xiao, W. Zhou, H. Cai, L. Zhang, K. M. Rosso, C. J. Mundy, G. K. Schenter and J. J. De Yoreo, Proc. Natl. Acad. Sci. U. S. A., 2017, 114, 7537–7542 CrossRef CAS PubMed.
- T. M. Gwon, J. H. Kim, G. J. Choi and S. J. Kim, J. Mater. Sci., 2016, 51, 6897–6912 CrossRef CAS.
- S. N. Gorb and V. L. Popov, Philos. Trans. R. Soc., A, 2002, 360, 211–225 CrossRef CAS PubMed.
-
M. Haq, A. Khomenko and G. L. Cloud, Cham, 2015, 4, 199–204.
- W. C. Puspitasari, F. Ahmad, S. Ullah, P. Hussain, P. S. M. Megat-Yusoff and P. J. Masset, Prog. Org. Coat., 2019, 127, 181–193 CrossRef CAS.
- K.-i. Mori and Y. Abe, Int. J. Lightweight Mater. Manuf., 2018, 1, 1–11 Search PubMed.
- W.-S. Kim, I.-H. Yun, J.-J. Lee and H.-T. Jung, Int. J. Adhes. Adhes., 2010, 30, 408–417 CrossRef CAS.
- J. Sun, F. Zhao, Y. Yao, Z. Jin, X. Liu and Y. Huang, Appl. Surf. Sci., 2017, 412, 424–435 CrossRef CAS.
- R. Libanori, D. Carnelli, N. Rothfuchs, M. R. Binelli, M. Zanini, L. Nicoleau, B. Feichtenschlager, G. Albrecht and A. R. Studart, Bioinspiration Biomimetics, 2016, 11, 036004 CrossRef CAS PubMed.
- J. F. Maguire, P. L. Talley and M. Lupkowski, J. Adhesion, 1994, 45, 269–290 CrossRef CAS.
- A. Faghihnejad, K. E. Feldman, J. Yu, M. V. Tirrell, J. N. Israelachvili, C. J. Hawker, E. J. Kramer and H. Zeng, Adv. Funct. Mater., 2014, 24, 2322–2333 CrossRef CAS.
- H. R. Brown, Annu. Rev. Mater. Sci., 1991, 21, 463–489 CrossRef CAS.
- M. Rinaldi and N. Casagli, Geomorphology, 1999, 26, 253–277 CrossRef.
- D.-M. Drotlef, L. Stepien, M. Kappl, W. J. P. Barnes, H.-J. Butt and A. del Campo, Adv. Funct. Mater., 2013, 23, 1137–1146 CrossRef CAS.
- M. Varenberg and S. Gorb, J. R. Soc., Interface, 2008, 5, 383–385 CrossRef CAS PubMed.
- E. W. Hart, Acta Metall., 1967, 15, 351–355 CrossRef CAS.
- I. Khan and B. T. Poh, J. Polym. Environ., 2011, 19, 793–811 CrossRef CAS.
- J. Annett and G. L. W. Cross, Nature, 2016, 535, 271–275 CrossRef CAS PubMed.
- Y. Shang, C. Wu, C. Hang, H. Lu and Q. Wang, Adv. Mater., 2020, 32, 2000189 CrossRef CAS PubMed.
- Y. Jiang, T. Liu and Y. Zhou, Adv. Funct. Mater., 2020, 30, 2006213 CrossRef CAS.
- J. Kim, Y. Lee, M. Kang, L. Hu, S. Zhao and J.-H. Ahn, Adv. Mater., 2021, 33, 2005858 CrossRef CAS PubMed.
- A. Inoue, H. Yuk, B. Lu and X. Zhao, Sci. Adv., 2020, 6, eaay5394 CrossRef CAS PubMed.
- J. Saiz-Poseu, J. Mancebo-Aracil, F. Nador, F. Busque and D. Ruiz-Molina, Angew. Chem., Int. Ed., 2019, 58, 696–714 CrossRef CAS PubMed.
- Z. Zheng, S. Bian, Z. Li, Z. Zhang, Y. Liu, X. Zhai, H. Pan and X. Zhao, Carbohydr. Polym., 2020, 249, 116826 CrossRef CAS PubMed.
- J. Shin, J. S. Lee, C. Lee, H.-J. Park, K. Yang, Y. Jin, J. H. Ryu, K. S. Hong, S.-H. Moon, H.-M. Chung, H. S. Yang, S. H. Um, J.-W. Oh, D.-I. Kim, H. Lee and S.-W. Cho, Adv. Funct. Mater., 2015, 25, 3814–3824 CrossRef CAS.
- W. Zhang, Z. Pan, F. K. Yang and B. Zhao, Adv. Funct. Mater., 2015, 25, 1588–1597 CrossRef CAS.
- Z. Wang, X. Jiang, K. Huang, L. Ning, J. Zhang, F. Zhang, J. Yang, Y. Wu, X. Chen, Y. Yi, X. Shi, Y. Chen and S. Wang, Adv. Mater., 2021, 2106067 CrossRef CAS PubMed.
- D. Bryant, P. Greenwood, J. Troughton, M. Wijdekop, M. Carnie, M. Davies, K. Wojciechowski, H. J. Snaith, T. Watson and D. Worsley, Adv. Mater., 2014, 26, 7499–7504 CrossRef CAS PubMed.
- Y. Li, X. Li, S. Zhang, L. Liu, N. Hamad, S. R. Bobbara, D. Pasini and F. Cicoira, Adv. Funct. Mater., 2020, 30, 2002853 CrossRef CAS.
Footnote |
† Z. W. and W. W. contributed equally to this work. |
|
This journal is © The Royal Society of Chemistry 2022 |
Click here to see how this site uses Cookies. View our privacy policy here.