DOI:
10.1039/D1TC03614A
(Paper)
J. Mater. Chem. C, 2022,
10, 2711-2717
Facile synthesis, precise species control and chemical transformation of highly conducting organic metal chalcogenides CuxBHT (BHT = benzenehexathiol; x = 3, 4, and 5.5)†
Received
3rd August 2021
, Accepted 20th September 2021
First published on 23rd September 2021
Abstract
The design of conducting organic metal chalcogenides (OMCs) has attracted extensive attention for their applications in diverse areas. However, only a handful of OMCs exhibit appealing electrical transport properties due to the limited synthetic approaches. Herein, a facile and controllable synthetic approach for the preparation of highly crystalline benzenehexathiol (BHT)-based OMCs using Cu2O as a precursor is reported. Under heterogeneous conditions, a series of highly conducting organic metal chalcogenides CuxBHT (x = 3, 4, and 5.5) were precisely constructed via variation of the molar ratio between Cu2O and BHT. In particular, a fascinating chemical transformation phenomenon was discovered in this work. This is the first time that a phase transition has been observed in conducting OMCs, with the semiconducting species (Cu4BHT and Cu5.5BHT) being converted to metallic species (Cu3BHT) under delicate oxidation regulation. This work provides a prominent paradigm for constructing highly crystalline OMCs, and opens up the possibility of developing OMCs with different structural topologies through chemical transformation.
Introduction
Organic metal chalcogenides (OMCs) refer to a class of hybrid materials containing continuous M–X (X = S, Se, Te) networks and organic units covalently linked via chalcogen atoms.1,2 Owing to their highly tunable electronic structures and electrical transport properties, OMCs have received extensive attention in recent years.3,4 Based on their chemical and electronic structures, OMCs can be divided into two subclasses. The first type of OMCs has one-dimensional (1D) and two-dimensional (2D) organic units separated by inorganic cores, and there is weak electronic coupling between the inorganic and organic subunits. This species is the most reported one, which is usually constructed with ligands bearing only one chalcogen coordination group.5–7 Due to their 1D or 2D core–shell structures, highly anisotropic transport properties could be expected which leads to relatively inferior performance. This is especially evident for OMCs obtained as polycrystalline samples.8 This situation has changed obviously with the emergence of the 2nd generation OMCs constructed from benzenehexathiol (BHT), which display strong electronic coupling among the inorganic subunits as well as between the organic and inorganic subunits, while the organic units (conjugated molecular systems) are embedded in the inorganic networks. Apart from the commonly observed 2D honeycomb lattice containing discrete MS4 subunits separated by benzene rings,9,10 BHT displays great flexibility in constructing coordination polymers with different structural topologies. From the early reported Pb3BHT11 to the later Ag5BHT12 and CuxBHT (x = 3, 4, and 5.5),13,14 all these materials are OMCs with 2D or complex 3D M–S networks.
Compared with the 1st generation OMCs, BHT-based 2nd generation OMCs display superior electrical transport properties,15 as well as great potential for electrochemical catalysts,10,16 transparent electrodes17 and energy storage devices.18,19 Moreover, exotic quantum phenomena have been observed in Cu3BHT such as unconventional superconductivity and quantum spin liquid (QSL) behaviour.20,21 All these characteristics make these BHT-based OMCs extremely attractive especially the CuxBHT family. However, the synthetic methods that are crucial for crystallinity and phase purity control are less investigated. For Cu4BHT and Cu5.5BHT, it is supposed that unintentional oxidation of the ligand plays a critical role in the resulting species control according to their nonstoichiometric composition.13 But it remains a highly tricky process for the synthesis of Cu5.5BHT with sufficient phase purity through a homogeneous reaction. In addition, the tendency of Cu5.5BHT converting to Cu4BHT and even to Cu3BHT has been observed with the reaction prolonged. But how to achieve a controllable transformation among these CuxBHT members is still elusive. The solution to this conundrum will offer an alternative synthesis strategy for these OMCs and will provide valuable information about the chemical state of building units in the polymers.
Herein, we successfully obtained all members of the CuxBHT (x = 3, 4, and 5.5) family through a facile heterogeneous reaction using Cu2O as the copper source. The identity and phase purity of the resulting OMCs can be easily controlled by modulating the molar ratio of Cu2O and BHT. Furthermore, we discovered that the chemical transformation can be realized within the CuxBHT family by using tris(4-bromophenyl)aminium hexachloroantimonate ((4-BrPh)3NSbCl6) as the oxidizing reagent. Under precise oxidation regulation with (4-BrPh)3NSbCl6, Cu5.5BHT can be converted to Cu4BHT, and even to Cu3BHT under further oxidation. This is the first time that such fascinating chemical transformation among the semiconducting OMCs (Cu5.5BHT to Cu4BHT), semiconducting to metallic OMCs (Cu5.5BHT, Cu4BHT to Cu3BHT), has been observed. These results provide a simple and controllable strategy for the synthesis of highly crystalline OMCs, and highlight the significance of chemical structure regulation as well as the electronic structure modulation of OMCs by a chemical method.
Results and discussion
Synthesis and characterization
As schemed in Fig. 1, CuxBHT series were prepared at 80 °C by modulating appropriate stoichiometric ratios between Cu2O and BHT. Under an argon atmosphere, when 1.5 equivalents of Cu2O (23.6 mg, 0.165 mmol) and BHT (30 mg, 0.11 mmol) were mixed in 30 mL of degassed ethanol and reacted for 72 h, a black Cu3BHT sample was obtained. Similarly, when 2 equivalents of Cu2O (31.5 mg, 0.22 mmol) and BHT (30 mg, 0.11 mmol) reacted for 48 h in 30 mL of degassed ethanol, a dark blue Cu4BHT was obtained. When 2.75 equivalents of Cu2O (43.3 mg, 0.303 mmol) and BHT (30 mg, 0.11 mmol) reacted for 48 h in 30 mL of degassed acetonitrile, a dark green Cu5.5BHT was obtained. The strong signal at ∼2490 cm−1 that can be ascribed to the S–H stretching vibration in BHT disappeared (Fig. S1, ESI†), indicating that all the thiol groups of BHT participated in the reaction with Cu2O. Besides, powder X-ray diffraction (PXRD), elemental analysis (EA), and inductively coupled plasma (ICP) were performed to confirm that the attained samples had the same components and structures as previously reported (Fig. S2–S4, see the ESI†).13,20 The self-assembly coordination and reaction kinetics between Cu2O and thiolate species are quite unique.8,22,23 We have observed that Cu3BHT can also be obtained by a long-term reaction of 1.5 equivalents of Cu2O with BHT, which is quite different from the previously reported CuCl2 (Fig. 1).16 Recent magnetic susceptibility measurements20 in Cu3BHT unveiled that the oxidation state of Cu is +2, and thus we infer that the Cu+ source is oxidized to Cu2+ gradually during the reaction process.
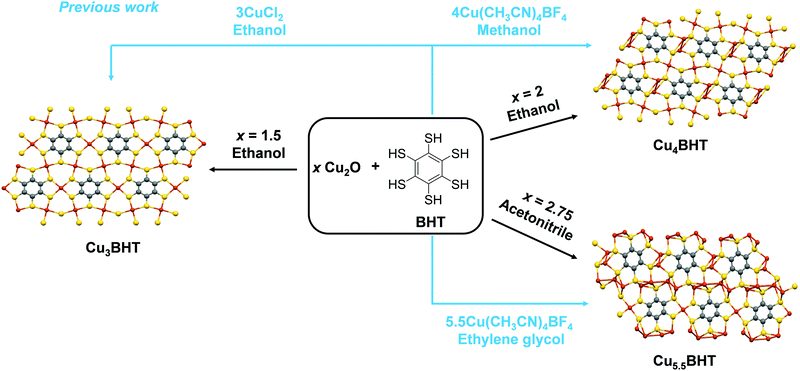 |
| Fig. 1 Comparison of the synthetic methods of CuxBHT (x = 3, 4, and 5.5). The schematic synthesis of CuxBHT using different metal sources and reaction solvents. The previously reported pathways (ref. 13 and 16) are indicated by blue lines and text. | |
Typically, PXRD data were collected to investigate the crystallinity of the obtained samples. As evidenced by sharp, well-isolated diffraction peaks, the crystallinity of Cu3BHT is much higher than that of the products obtained with Cu2+ salts as reactants (Fig. S2, ESI†). Meanwhile, the morphologies of CuxBHT (x = 3, 4, and 5.5) were characterized by scanning electron microscopy (SEM) and transmission electron microscopy (TEM) (Fig. 2). As indicated in the SEM images, all three samples displayed regular rod-like structures with a smooth surface and uniform size. The as-prepared nanorods are a few tens of nanometers in diameter and range from 500 nm (Cu3BHT) to 2 μm (Cu4BHT and Cu5.5BHT) in length (Fig. 2d–f), which are longer than the nanocrystals prepared with Cu2+ and [Cu(CH3CN)4]BF4 salts.13,16 In particular, the selected area electron diffraction (SAED) pattern of an individual nanorod revealed regularly spaced arrays of diffraction spots, which can be assigned very well to the respective crystal indices, as shown in Fig. 2g–i. All the PXRD, SEM and SAED results verified that the samples prepared with Cu2O as precursors are highly crystalline OMCs, which are expected to be applied in microelectronic devices based on nano-crystalline materials. The improved crystallinity can be attributed to three aspects. First, it is believed that temperature is a crucial parameter during the growth process of OMCs. Generally, higher temperatures will improve the crystallinity of the resulting materials as the coordination bonding is more reversible, and defects can be healed during the crystal growth process. Second, the slow nucleation and growth process are critically important to obtain highly crystalline OMCs. Compared to common Cu2+ sources, Cu(I) salts have a moderate reaction rate with organothiolates due to their more complicated d10 chemistry.24,25 Furthermore, BHT and Cu2O have poor solubility in reaction solvents, which reduces the reaction concentration and diffusion rate of precursors, thus resulting in improved crystallinity.
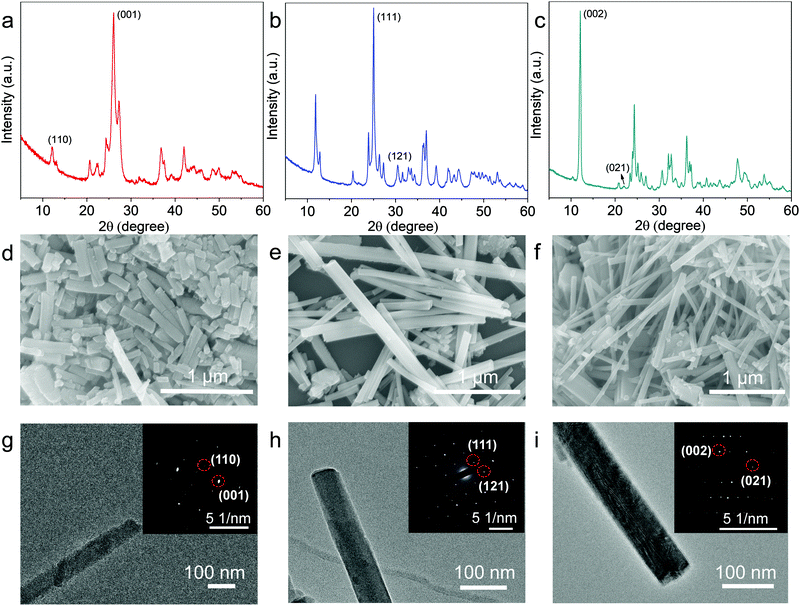 |
| Fig. 2 Characterization of CuxBHT. (a–c) PXRD patterns of Cu3BHT, Cu4BHT and Cu5.5BHT, respectively. (d–f) SEM images of Cu3BHT, Cu4BHT and Cu5.5BHT, respectively. (g–i) TEM images of Cu3BHT, Cu4BHT and Cu5.5BHT, respectively. Insets correspond to SAED patterns. | |
Charge transport properties of CuxBHT
Electrical property characterization will provide further evidence on the identity of these CuxBHTs. To investigate the charge transport properties of these OMCs, the variable-temperature conductivities of the pressed pellets of CuxBHT were analysed using a standard four-probe method. As shown in Fig. 3, the conductivities of all the CuxBHT samples are compared with the previously reported results and are significantly higher than those of the most reported OMCs,8,15,19,26 thus rendering them promising potential for applications in the microelectronics industry.27,28 Among these three OMCs, Cu3BHT features the highest electrical conductivity (215 to 286 S cm−1 with an average value of 244 S cm−1) compared with those of Cu4BHT (153 to 184 S cm−1 with an average value of 170 S cm−1) and Cu5.5BHT (144–166 S cm−1 with an average value of 155 S cm−1). According to Arrhenius fitting, the plots of ln
σ(T) versus the reciprocal of the temperature give the activation energy (Ea) of each sample (Fig. 3d). The Ea values for the electron hopping of these three OMCs at 300 K are in the sequence of Cu3BHT (5.74 meV) < Cu4BHT (9.17 meV) < Cu5.5BHT (14.7 meV), which explains the fact that the conductivities of CuxBHT members are in the sequence of Cu3BHT > Cu4BHT > Cu5.5BHT. With the temperature increasing from 300 K to 380 K, the Ea of all three OMCs continuously increases, which indicates the defects, most likely the grain boundaries between neighbouring CuxBHT nanocrystals that influence the charge transport behaviour.
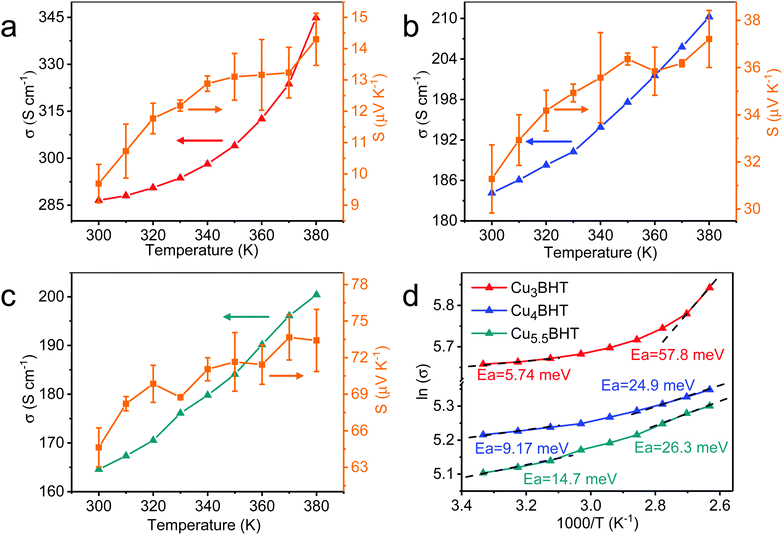 |
| Fig. 3 The charge transport properties of CuxBHT. (a–c) Curves of the variable-temperature electrical conductivity and Seebeck coefficient of Cu3BHT, Cu4BHT and Cu5.5BHT, respectively. (d) Plots of ln σ(T) versus 1000/T of CuxBHT. | |
It is obvious that the Ea of three OMCs appears quite small, especially in low temperature ranges. To figure out the origin of low Ea and observed high conductivities, three samples were subjected to ultraviolet photoelectron spectroscopy (UPS).
As illustrated in Fig. 4, all three samples displayed a clear Fermi edge near the top of the valence band, indicating that they are metallic conductors or degenerate semiconductors. As disclosed by band structure calculations, three networks exhibit substantial differences in their electronic structures. Cu3BHT has a metallic nature,20,21 while Cu4BHT and Cu5.5BHT behave as degenerate semiconductors.13 The differences in structural topology as well as the variation of hybridization between the d orbitals of the Cu ions and the π orbitals of the BHT ligands result in different band structures in the CuxBHT family. In addition, the variable-temperature Seebeck coefficient measurement displays that Cu3BHT has a small Seebeck coefficient ranging from 9 to 15 μV K−1 (Fig. 3a–c). Such a low Seebeck coefficient and the weak temperature dependence of conductivity in Cu3BHT are typically observed in metals or highly conducting polymers,29 which further demonstrates its metallic characteristic. In comparison, the Seebeck coefficients of Cu4BHT and Cu5.5BHT are 30–40 μV K−1 and 60–80 μV K−1, respectively, which are about 3∼6 times larger than that of Cu3BHT. The higher Seebeck coefficients suggest that Cu4BHT and Cu5.5BHT are more like degenerate semiconductors rather than metals, which is consistent with band structure calculation results.13
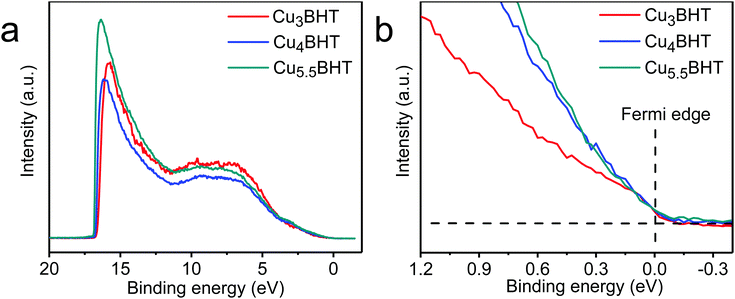 |
| Fig. 4 The UPS analysis of CuxBHT acquired at 300 K. The full spectra and the Fermi edge are shown in (a) and (b), respectively. | |
Oxidation regulation within the CuxBHT family
Enlightened by the knowledge that redox has a significant influence on the oxidation states of metal bis(dithiolene) species and their electrical transport properties,12 here, valence-variable (4-BrPh)3NSbCl6 is employed as the oxidizing reagent since it is known to better control the oxidation states of the metal bis(dithiolene) unit.15,30,31 In order to investigate the influence of the degree of oxidation on the final phases, different equivalents of (4-BrPh)3NSbCl6 were added to Cu4BHT and Cu5.5BHT using methanol as a reaction medium. After post-preparation modification, the crystallinity and composition of the oxidation products were identified by PXRD, EA and ICP measurements (see Fig. S5 and S6, ESI†). Surprisingly, under an argon atmosphere, when 1.5 equivalents of (4-BrPh)3NSbCl6 (29.4 mg, 0.036 mmol) were added to Cu5.5BHT (15 mg, 0.024 mmol) in 25 mL of CH3OH, a dark blue Cu4BHT sample was obtained at room temperature. When 2.5 equivalents of (4-BrPh)3NSbCl6 (49 mg, 0.06 mmol) were added to Cu5.5BHT (15 mg, 0.024 mmol) or 1 equivalent of (4-BrPh)3NSbCl6 (24 mg, 0.029 mmol) was added to Cu4BHT (15 mg, 0.029 mmol), a black Cu3BHT sample was obtained (Fig. 5 and Fig. S2, ESI†). Such oxidation regulation accompanied by the changes in topological structures is quite rare among the other coordination polymers ever reported.32–35 In addition, with the deepening oxidation degree, the colours of polycrystalline samples become gradually darker, varying from dark green, dark blue to black (Fig. 5). The visible difference in colours suggested that CuxBHT series have potential as light-harvesting or electrochromic materials.
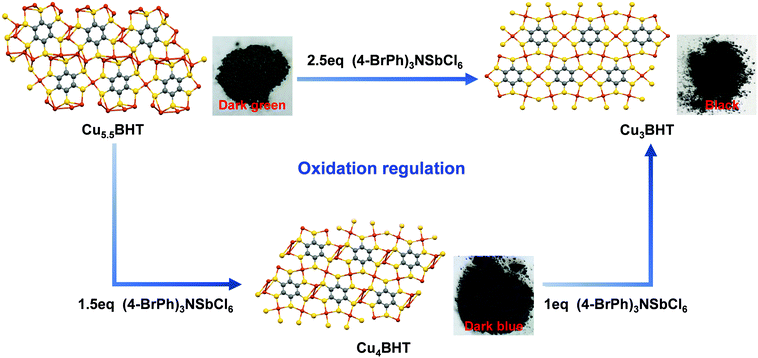 |
| Fig. 5 Oxidation regulation within the CuxBHT family. Schematic illustration of phase transition in CuxBHT species upon delicate oxidation regulation with (4-BrPh)3NSbCl6. Photograph shows the colours of each CuxBHT species. | |
During oxidation, the Cu–BHT networks lose electrons and trigger structural conversions, and the entire system remains electrically neutral. After oxidation, the elemental composition of the oxidized products was analysed by X-ray photoelectron spectroscopy (XPS) (Fig. S7, ESI†). The Cu (2p) region exhibited a strong satellite peak, suggesting that the valence state of Cu in the remaining filtrate is +2. In the high-resolution Sb 3d XPS spectrum, two sets of peaks with binding energies of ∼540.4 and ∼531.1 eV were observed and assigned, respectively, to the 3d3/2 and 3d5/2 levels of Sb, revealing that [SbCl6]− was reduced to [SbCl6]3−. On the basis of the charge balance, when Cu5.5BHT loses 3 electrons, Cu4BHT was generated, while Cu3BHT was obtained when it loses 5 electrons. Similarly, Cu4BHT can transform into Cu3BHT after losing 2 electrons, so Cu4BHT is more like an intermediate state of transition from Cu5.5BHT to Cu3BHT. But it is a controversial issue to give a clear definition of whether the oxidation process is metal-centred or ligand-centred. We can infer that the copper bis(dithiolene) coordination unit, as an indivisible moiety, acts as an electron reservoir that can undergo complex redox switching as a whole. Thus, the ligand and the metal here cooperate in a synergistic manner, and their interplay facilitates the redox switching process. Therefore, the uniqueness of the oxidation states of BHT and the flexibility of the coordination geometries of Cu(I) ions result in rich members in the CuxBHT family. Additionally, Cu5.5BHT and Cu4BHT can be regarded as the reaction precursors of Cu3BHT employing valence-variable [SbCl6]− as an oxidant. With this in mind, we hypothesized that the structural transformation induced by oxidation could be a promising synthetic shortcut to prepare the desired OMCs. Since the structural transformations in the CuxBHT family are accompanied by the changes in the optical, electrical and magnetic properties, they are expected to be employed for advanced functional applications such as sensors or electrochromic devices.
Conclusions
In summary, three highly conducting OMCs – Cu3BHT, Cu4BHT and Cu5.5BHT – have been effectively prepared and characterized. By modulating the suitable feed ratios between Cu2O and BHT, a series of CuxBHT (x = 3, 4, and 5.5) materials can be precisely generated, which has been evidenced by the combined analysis of EA, PXRD, SEM and SAED measurements. Here, the binary oxide Cu2O as a metal source not only exhibits rich coordination capacity in constructing OMCs with different structural topologies, but also possesses moderate thiophilicity towards BHT, thereby obtaining a series of highly crystalline OMCs. It is particularly noteworthy that this synthetic pathway is facile and straightforward yet allows precise phase purity control. This discovery provides the possibility of producing new types of highly conducting OMCs. Most importantly, we demonstrate for the first time that the fantastic chemical transformation within the CuxBHT family can be realized under delicate oxidation regulation, in which both the semiconducting Cu5.5BHT and Cu4BHT can be converted to metallic Cu3BHT. This interesting phenomenon highlights the importance of structural transformation for the control of electronic structures of OMCs. Considering the tailorable chemical structures and excellent performance of CuxBHT species, we believe that these OMCs have substantial potential for applications in the future.
Conflicts of interest
There are no conflicts to declare.
Acknowledgements
The authors acknowledge the financial support from the National Key R&D Program of China (Grant No. 2017YFA0204701), the National Natural Science Foundation of China (Grant 22071256), and the Chinese Academy of Sciences (QYZDY-SSW-SLH024).
Notes and references
- W. P. Su, M. C. Hong, J. B. Weng, Y. C. Liang, Y. J. Zhao, R. Cao, Z. Y. Zhou and A. S. C. Chan, Inorg. Chim. Acta, 2002, 331, 8–15 CrossRef.
- H. Yan, J. N. Hohman, F. H. Li, C. Jia, D. Solis-Ibarra, B. Wu, J. E. P. Dahl, R. M. K. Carlson, B. A. Tkachenko, A. A. Fokin, P. R. Schreiner, A. Vailionis, T. R. Kim, T. P. Devereaux, Z.-X. Shen and N. A. Melosh, Nat. Mater., 2017, 16, 349–355 CrossRef.
- O. Veselska and A. Demessence, Coord. Chem. Rev., 2018, 355, 240–270 CrossRef.
- Y. Li, X. Jiang, Z. Fu, Q. Huang, G.-E. Wang, W.-H. Deng, C. Wang, Z. Li, W. Yin, B. Chen and G. Xu, Nat. Commun., 2020, 11, 261 CrossRef PubMed.
- D. V. P. Massote and M. S. C. Mazzoni, Appl. Phys. Lett., 2016, 109, 133104 CrossRef.
- Y. Zhang, T. Xia, K. M. Yu, F. Zhang, H. Yang, B. Liu, Y. An, Y. Yin and X. Chen, ChemPlusChem, 2014, 79, 559–563 CrossRef.
- C. Lavenn, N. Guillou, M. Monge, D. Podbevsek, G. Ledoux, A. Fateeva and A. Demessence, Chem. Commun., 2016, 52, 9063–9066 RSC.
- K. H. Low, V. A. Roy, S. S. Chui, S. L. Chan and C. M. Che, Chem. Commun., 2010, 46, 7328–7330 RSC.
- T. Kambe, R. Sakamoto, K. Hoshiko, K. Takada, M. Miyachi, J. H. Ryu, S. Sasaki, J. Kim, K. Nakazato, M. Takata and H. Nishihara, J. Am. Chem. Soc., 2013, 135, 2462–2465 CrossRef.
- A. J. Clough, J. W. Yoo, M. H. Mecklenburg and S. C. Marinescu, J. Am. Chem. Soc., 2015, 137, 118–121 CrossRef PubMed.
- D. L. Turner, T. P. Vaid, P. W. Stephens, K. H. Stone, A. G. DiPasquale and A. L. Rheingold, J. Am. Chem. Soc., 2008, 130, 14–15 CrossRef.
- X. Huang, H. Li, Z. Tu, L. Liu, X. Wu, J. Chen, Y. Liang, Y. Zou, Y. Yi, J. Sun, W. Xu and D. Zhu, J. Am. Chem. Soc., 2018, 140, 15153–15156 CrossRef CAS PubMed.
- X. Huang, Y. Qiu, Y. Wang, L. Liu, X. Wu, Y. Liang, Y. Cui, Y. Sun, Y. Zou, J. Zhu, W. Fang, J. Sun, W. Xu and D. Zhu, Angew. Chem., Int. Ed., 2020, 59, 22602–22609 CrossRef CAS PubMed.
- X. Huang, P. Sheng, Z. Tu, F. Zhang, J. Wang, H. Geng, Y. Zou, C. A. Di, Y. Yi, Y. Sun, W. Xu and D. Zhu, Nat. Commun., 2015, 6, 7408 CrossRef CAS.
- T. Kambe, R. Sakamoto, T. Kusamoto, T. Pal, N. Fukui, K. Hoshiko, T. Shimojima, Z. Wang, T. Hirahara, K. Ishizaka, S. Hasegawa, F. Liu and H. Nishihara, J. Am. Chem. Soc., 2014, 136, 14357–14360 CrossRef CAS.
- X. Huang, H. Yao, Y. Cui, W. Hao, J. Zhu, W. Xu and D. Zhu, ACS Appl. Mater. Interfaces, 2017, 9, 40752–40759 CrossRef CAS.
- Z. W. Jin, J. Yan, X. Huang, W. Xu, S. Y. Yang, D. B. Zhu and J. Z. Wang, Nano Energy, 2017, 40, 376–381 CrossRef CAS.
- Z. Wu, D. Adekoya, X. Huang, M. J. Kiefel, J. Xie, W. Xu, Q. Zhang, D. Zhu and S. Zhang, ACS Nano, 2020, 14, 12016–12026 CrossRef CAS.
- H. Banda, J. H. Dou, T. Chen, N. J. Libretto, M. Chaudhary, G. M. Bernard, J. T. Miller, V. K. Michaelis and M. Dinca, J. Am. Chem. Soc., 2021, 143, 2285–2292 CrossRef CAS.
- X. Huang, S. Zhang, L. Liu, L. Yu, G. Chen, W. Xu and D. Zhu, Angew. Chem., Int. Ed., 2018, 57, 146–150 CrossRef CAS.
- T. Takenaka, K. Ishihara, M. Roppongi, Y. Miao, Y. Mizukami, T. Makita, J. Tsurumi, S. Watanabe, J. Takeya, M. Yamashita, K. Torizuka, Y. Uwatoko, T. Sasaki, X. Huang, W. Xu, D. Zhu, N. Su, J. G. Cheng, T. Shibauchi and K. Hashimoto, Sci. Adv., 2021, 7, eabf3996 CrossRef CAS PubMed.
- N. Arisnabarreta, P. Paredes-Olivera, F. P. Cometto and E. M. Patrito, J. Phys. Chem. C, 2019, 123, 17283–17295 CrossRef CAS.
- L. Wu, Y. Jiao, K. Zhang, F. Wu, W. Zhao, M. Sun, A. Xie and W. Dong, J. Mater. Chem. C, 2019, 7, 11621–11631 RSC.
- O. Veselska and A. Demessence, Coord. Chem. Rev., 2018, 355, 240–270 CrossRef CAS.
- O. Veselska, L. Cai, D. Podbevsek, G. Ledoux, N. Guillou, G. Pilet, A. Fateeva and A. Demessence, Inorg. Chem., 2018, 57, 2736–2743 CrossRef CAS PubMed.
- Y. Cui, J. Yan, Z. Chen, J. Zhang, Y. Zou, Y. Sun, W. Xu and D. Zhu, Adv. Sci., 2019, 6, 1802235 CrossRef PubMed.
- K. T. Butler, C. H. Hendon and A. Walsh, ACS Appl. Mater. Interfaces, 2014, 6, 22044–22050 CrossRef CAS.
- E. A. Dolgopolova, A. J. Brandt, O. A. Ejegbavwo, A. S. Duke, T. D. Maddumapatabandi, R. P. Galhenage, B. W. Larson, O. G. Reid, S. C. Ammal, A. Heyden, M. Chandrashekhar, V. Stavila, D. A. Chen and N. B. Shustova, J. Am. Chem. Soc., 2017, 139, 5201–5209 CrossRef CAS.
- N. Massonnet, A. Carella, A. de Geyer, J. Faure-Vincent and J. P. Simonato, Chem. Sci., 2015, 6, 412–417 RSC.
- J. F. Berry, F. A. Cotton, L. M. Daniels, C. A. Murillo and X. Wang, Inorg. Chem., 2003, 42, 2418–2427 CrossRef CAS PubMed.
- G. W. Cowell, A. Ledwith, A. C. White and H. J. Woods, J. Chem. Soc. B, 1970, 227–231 RSC.
- J. J. Vittal, Coord. Chem. Rev., 2007, 251, 1781–1795 CrossRef CAS.
- G. K. Kole and J. J. Vittal, Chem. Soc. Rev., 2013, 42, 1755–1775 RSC.
- T. H. Kim, Y. W. Shin, J. H. Jung, J. S. Kim and J. Kim, Angew. Chem., Int. Ed., 2008, 47, 685–688 CrossRef CAS.
- K. J. Lee, J. H. Lee, S. Jeoung and H. R. Moon, Acc. Chem. Res., 2017, 50, 2684–2692 CrossRef CAS.
Footnote |
† Electronic supplementary information (ESI) available. See DOI: 10.1039/d1tc03614a |
|
This journal is © The Royal Society of Chemistry 2022 |
Click here to see how this site uses Cookies. View our privacy policy here.