DOI:
10.1039/C9QI01516G
(Review Article)
Inorg. Chem. Front., 2020,
7, 817-838
Tungsten oxide-based visible light-driven photocatalysts: crystal and electronic structures and strategies for photocatalytic efficiency enhancement
Received
21st November 2019
, Accepted 26th December 2019
First published on 30th December 2019
Abstract
Photocatalysis (PC) technology has received global attention due to its high potential of addressing both environmental and energy issues using only solar light as energy input. However, large-scale commercialization of PC technology is still far from expectation, which is primarily limited by low efficiency. The development of PC technology depends crucially on photocatalyst materials. In the past half century, TiO2 has been mostly investigated and developed as a benchmark photocatalyst. However, TiO2 responds intrinsically only to UV light, which has limited its efficient utilization of solar energy and restrained its applications to where UV light is not available, e.g., indoor air purification. The development of novel intrinsically visible light-driven photocatalysts has been a new trend of PC technology. Amongst the various visible-light responsive candidates, tungsten oxides (WOX, X ≤ 3) have attracted much attention due to their diversely tunable stoichiometries and structures, suitable band gaps, chemical stability and Earth-abundance. However, bare WOX exhibits comparatively low efficiency because of the fast recombination rate of photogenerated electrons and holes. Various strategies have been developed to enhance the photocatalytic efficiency of WOX, including the controls in the crystal phase, stoichiometry/oxygen-vacancy, active facet and morphology, elemental doping, loading of noble metal nanoparticles, hybridization with carbon materials and coupling with other semiconductors to construct heterojunctions. This review summarizes firstly the fundamentals of WOX (i.e., crystal and electronic structures and optical properties) and then highlights the strategies for the enhancement of the photocatalytic efficiency of WOX-based photocatalysts. The related synthesis methods are also briefly discussed. It is anticipated that this paper could offer a comprehensive understanding of WOX-based photocatalysts and serve as a guideline for future designs of highly active visible light-driven WOX-based photocatalysts.
Introduction
In the past half century, photocatalysis (PC) technology has attracted global attention due to its high potential of addressing both environmental and energy issues using only solar light as energy input. To date, PC technology has been widely applied to various fields (see Fig. 1), such as water splitting for H2 and O2 generation,1–6 CO2 reduction for fuels and to alleviate the global warming crisis,7,8 N2 fixation for ammonia,9 wastewater treatment,10,11 air purification,12,13 soil remediation,14 self-cleaning surfaces,15 anti-bacteria/virus,16,17etc. The merits of PC technology are fundamentally due to the strong redox ability of photocatalysts induced by proper illumination. However, large-scale commercialization of PC technology is still far from expectation, which is primarily limited by low efficiency.
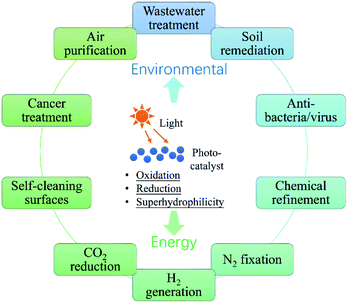 |
| Fig. 1 Applications of photocatalysis. | |
The development of PC technology depends crucially on photocatalyst materials, which are generally semiconductors composed of a valence band (VB) filled with electrons and a conduction band (CB) empty of electrons. When irradiated by photons with energy higher than or equal to the band gap (i.e., hv ≥ Eg), a photocatalyst could be excited with part of electrons jumping from the VB to the CB, leaving excited electrons and holes in the CB and VB, respectively (see Fig. 2(i)).18,19 The photogenerated electrons and holes, also called charge carriers, could transfer to the surface of the photocatalyst and then react with the adsorbed electron-acceptors (A) and electron-donors (D), respectively, initiating both photocatalytic reduction and oxidation reactions (Fig. 2(ii) and (iii)).18,19 However, before participating in photocatalytic redox reactions, the photogenerated electron–hole pairs might have recombined quickly in bulk or on the surface of the photocatalyst (Fig. 2(iv) and (v)), as they are subjected to a strong Coulomb force.20 This is one main reason for the low efficiency of photocatalysis.
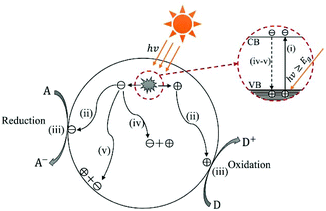 |
| Fig. 2 Schematic photoexcitation in a solid photocatalyst followed by deexcitation (A: electron-acceptor; D: electron-donor). | |
In the past several decades, TiO2 has been mostly investigated and developed as a benchmark photocatalyst.21,22 However, as a wide band gap semiconductor (3.0–3.2 eV), TiO2 responds intrinsically only to UV light that occupies merely 3–5% of the solar spectrum.23 This has limited its efficient utilization of solar energy and restrained its applications to where UV light is not available, e.g., indoor air purification. Although many efforts have been made to extend light absorption of TiO2,24–28 the efficiency of TiO2-based photocatalysts under visible light irradiation is still unsatisfactory. In recent years, the development of novel intrinsically visible light-driven photocatalysts has been a new trend of PC technology. To date, various narrow bandgap (<3.0 eV) semiconductors have been developed as visible-light sensitive photocatalysts, such as WO3,29 α-Fe2O3,30 CdS,31 BiVO4,32 Bi2WO6,33,34 Ag3PO4,35 g-C3N4,36etc. Amongst them, tungsten oxides (WOX, X ≤ 3) have received increasing interest (a publication survey is shown in Fig. 3) due to their easy preparation, diversely tuneable stoichiometries and structures, suitable band gaps (2.4–2.8 eV, responsive to ∼12% of the solar spectrum), strong photocatalytic oxidizing ability, high chemical stability, nontoxicity, and Earth-abundance.37 Furthermore, WOX possesses a much higher carrier mobility (10–12 cm2 V−1 s−1) than TiO2 (0.3 cm2 V−1 s−1) and a comparatively longer hole diffusion length (150–500 nm) when compared with α-Fe2O3 (2–4 nm), both of which are essential for the transfer and separation of photogenerated charge carriers.38,39 However, bare WOX exhibits a relatively low efficiency because of the fast recombination rate of photogenerated charge carriers. This may have a close relationship with the low CB level (0.3–0.7 V vs. NHE) of WOX that is not negative enough for the single-electron reduction of oxygen (e.g., O2 + e− = O2˙− (aq), −0.284 V; O2 + H+ + e− = HO2˙ (aq), −0.046 V vs. NHE), which in turn, however, is important for the scavenging of photogenerated electrons.40 As the photogenerated electrons could not be consumed efficiently, they accumulate and lead to the increased recombination rate of charge carriers.
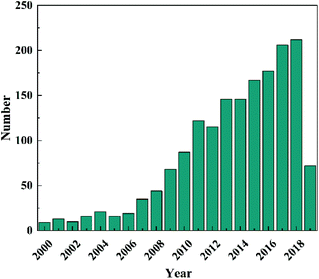 |
| Fig. 3 Publication survey in Web of Science using “tungsten oxide” and “photocatalytic” as keywords (since 2000). | |
In the literature, many strategies have been developed to improve the photocatalytic behaviour of WOX, such as the controls in the crystal phase,41–43 stoichiometry/oxygen-vacancy,38,44,45 active facet46–48 and morphology,49–51 elemental doping,52–54 loading of noble metal nanoparticles (NPs),55–57 hybridization with carbon materials58–60 and coupling with other semiconductors to construct heterojunctions.61–63 According to the basic PC processes (Fig. 2), any improvement in the following aspects can lead to an enhancement of the photocatalytic efficiency: (i) extend light absorption, (ii) facilitate charge transfer, (iii) inhibit the recombination of charge carriers, (iv) shorten the diffusion length for charge carriers, (v) increase the reactive sites on the surface of the photocatalyst, and (vi) increase the overall reaction surface area. The relationships between the various strategies and their possible resulted enhancements are summarized in Fig. 4.
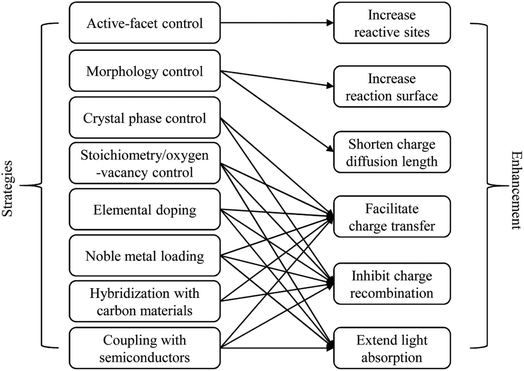 |
| Fig. 4 Relationship between the strategies and their main possible resulted enhancements. | |
Although there have been some review papers concerning WOX-based photocatalysts, they paid attention to only limited aspects, e.g., nanostructured WO3 thin films for photoelectrocatalytic (PEC) water oxidation64 and nanostructured-based WO3 for wastewater treatment.65 A comprehensive review regarding WOX-based photocatalysts, especially involving the advancements in the past few years, is still in need. This review summarizes firstly the fundamentals of WOX (i.e., crystal and electronic structures and optical properties) and then highlights the strategies for the improvement of the photocatalytic efficiency of WOX-based photocatalysts. It is anticipated that this paper could serve as a guideline for future designs of highly active visible light-driven WOX-based photocatalysts.
Fundamentals
Tungsten oxide (WOX) is a big family that is composed of stoichiometric WO3 and various sub-stoichiometric tungsten oxides (WO3−x, 0 < x ≤ 1). As tungsten oxide hydrates (WOX·nH2O) have a close relationship with their dehydrated counterparts and exhibit photocatalytic activity, they are considered herein as part of WOX-based photocatalysts. In this section, the crystal and electronic structures and optical properties of WOX and their hydrates are discussed.
Crystal structures
The ideal crystal structure of WO3 is identical to the cubic ReO3 type or ABO3 perovskite structure in the absence of an A cation, i.e., a three-dimensional network formed by corner-sharing WO6 octahedra.66 As illustrated in Fig. 5(a), the ideal cubic WO3 has W atoms at the corners of a cube, each surrounded by six octahedrally coordinated oxygens; four oxygens lie in a plane containing the W atoms and there is one oxygen above and one below this plane for each W atom; each oxygen is common to two octahedra, giving the formula of WO3. Cubic cavities with constant edges (about 2.7 Å, the length of O–O bonds) form the interstices of the network of WO6 octahedra (regular four-membered rings can be seen in the [100], [010] and [001] directions, respectively, as shown in Fig. 5(c)). The ideal cubic WO3 has never been observed experimentally.67 Bulk WO3 undergoes at least five reversible phase transitions upon heating or cooling (Fig. 6).68–71 This involves the tetragonal (t- or α-WO3),70,72 orthorhombic (o- or β-WO3),73 monoclinic I (m- or γ-WO3),71 triclinic (tr- or δ-WO3),68 and monoclinic II (ε-WO3) phases.66 All these phases of WO3 have a similar crystal structure to the ideal cubic WO3, however, with a lowered symmetry owing to three possible types of distortions: displacement of the W atom from its octahedron and distortion and tilting of WO6 octahedra.66 According to Corà et al., the lowering of the symmetry, especially by the off-central displacement of W atoms, results in an increase in the covalence between tungsten and its nearest oxygen, which thus leads to a more stable structure.67 At room temperature, m-WO3 has been reported as the most stable phase, with tr-WO3 also being observed.74 A hexagonal phase of WO3 is also frequently reported,75–77 which was firstly obtained by the dehydration of WO3·0.33H2O in 1979.78 The hexagonal h-WO3 is also build up from corner-sharing WO6 octahedra but their arrangement results in three- and six-membered rings in the ab-planes and leads to the formation of large hexagonal tunnels (∼5.6 Å) in the c-axis (see Fig. 5(c)). In the ac- and bc-planes, four-membered rings formed by WO6 octahedra, as in other phases, are also the case in h-WO3. According to Gerand et al., the unit cell of h-WO3 contains six molecules and shows lattice constants of a = 7.298 Å and c = 7.798 Å (Fig. 5(b)).78 More information about the crystal structures and lattice parameters of polymorphic WO3 can be found in Fig. 5(b) and (c).
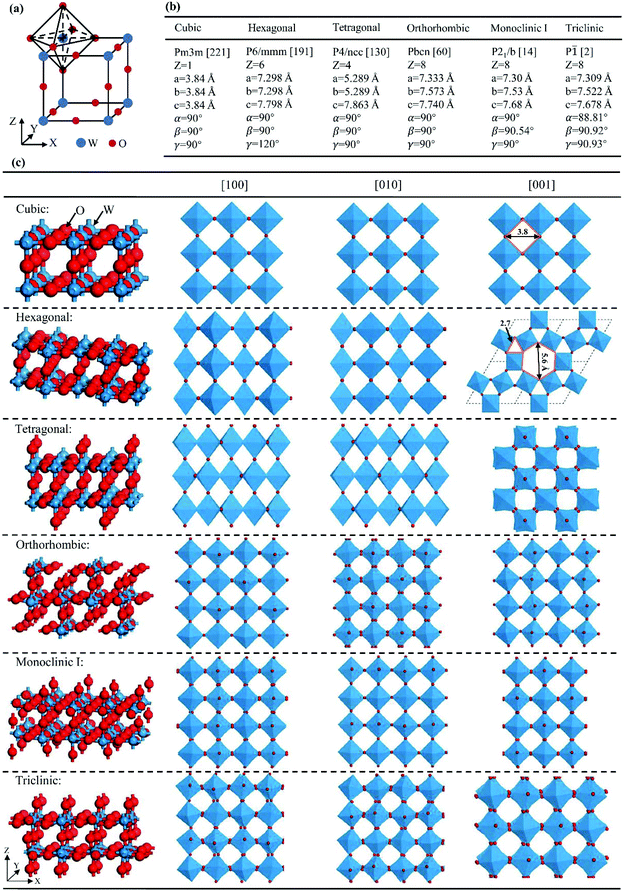 |
| Fig. 5 Crystal structures of polymorphic WO3: cubic WO3 and its WO6 octahedron (a), structural parameters (b), and stick–ball and polyhedral representations (c). | |
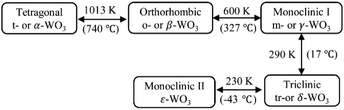 |
| Fig. 6 Classical phase transitions of bulk WO3 upon heating or cooling.68–71 | |
The lattice of WO3 can sustain considerable amounts of oxygen vacancies, however, this is accompanied by structural changes according to the degree of reduction. In a sub-stoichiometric WO3−x with a low degree of reduction (x < 0.01), single oxygen vacancies can be dispersed in the lattice in low concentration. However, with increasing x the lattice tends to eliminate single oxygen vacancies by a crystal shear (CS) mechanism, resulting in groups of edge-sharing WO6 octahedra arranged along some crystallographic planes (shear planes, SPs). For moderate x, these SPs are isolated and can be regarded as extended defects.79 With further increase in x, the SPs begin to interact and tend to align in parallel. If the SPs are all in parallel and equidistant, a crystal phase with a defined structure arises, which can be recognized as the Magnéli WnO3n−2 series.80 One such typical example is W20O58 (WO2.9), the structure of which is demonstrated in Fig. 7(a). When x ≥ 0.13, structures with pentagonal columns (PCs) become dominant. These structures contain two kinds of coordination polyhedra, viz., WO6 octahedra and WO7 pentagonal bipyramids. The WO7 bipyramids share their equatorial edges with five WO6 octahedra to form groups of PCs (generally parallel to the monoclinic b-axis). The variations of such structures depend on the modes of the linking of the PCs.81 One such typical example is W18O49 (WO2.72), as shown in Fig. 7(b). Other frequently investigated WO3−x with defined structures, also known as the Magnéli phases, include W32O84 (WO2.625), W3O8 (WO2.67), W17O47 (WO2.76), W5O14 (WO2.8), W25O73 (WO2.92), etc.82
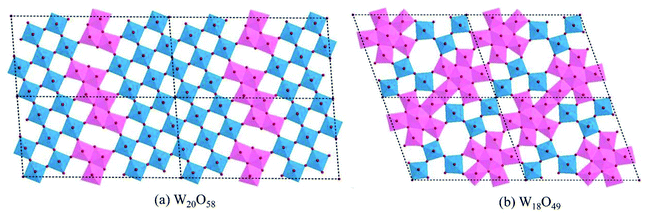 |
| Fig. 7 Idealized structures of W20O58 (a) and W18O49 (b) looking down from the [010] direction. | |
Hydrated tungsten oxides WOX·nH2O have been frequently obtained as intermediate products during the synthesis of WOX using a wet chemical route before annealing. The most widely investigated WOX·nH2O photocatalysts include monoclinic WO3·2H2O (dihydrate),83 orthorhombic WO3·H2O (monohydrate),84,85 cubic pyrochlore-type WO3·0.5H2O (hemihydrate),86 and orthorhombic WO3·0.33H2O.87 The crystal structures of WO3·nH2O are highly dependent on their water content. WO3·2H2O is built up from layers of corner-sharing WO5(OH2) octahedra and interlayer water molecules (Fig. 8(a)).88 Each WO5(OH2) octahedron consists of one W atom at the centre, one terminal oxygen, one coordinated water and four bridging oxygens, with which WO5(OH2) octahedra are connected to each other in the ac-planes forming neutral WO3·H2O layers. The interlayer water molecules connect with WO5(OH2) octahedra through hydrogen bonds.88 WO3·H2O can be obtained by removing the interlayer water from WO3·2H2O (Fig. 8(b)).89,90 WO3·0.5H2O is less documented and believed to have a structure of cubic pyrochlore-type, where the water molecules are presented in tunnels along the [110] direction, constructed by six-membered rings of corner-sharing WO6 octahedra (Fig. 8(c)).91 WO3·0.33H2O has been reported to consist of both WO6 and WO5(OH2) octahedra.92 A typical representation of orthorhombic WO3·0.33H2O is demonstrated in Fig. 8(d), where three- and six-membered rings are formed by corner-sharing WO6 and WO5(OH2) octahedra in the ab-planes.93
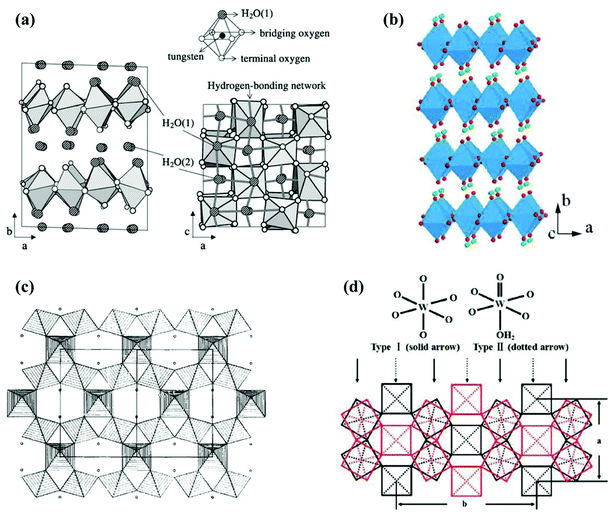 |
| Fig. 8 Crystal structures of tungsten oxide hydrates: monoclinic WO3·2H2O (a),88 orthorhombic WO3·H2O (green balls represent H atoms) (b),90 projection of cubic pyrochlore-type WO3·0.5H2O in the [110] direction (small circles represent water molecules) (c),91 and orthorhombic WO3·0.33H2O (d).93 Reprinted with permission from ref. 88, 90, 91 and 93. Copyright 2004 Elsevier, 2012 the Royal Society of Chemistry, 1989 Elsevier, and 2008 American Chemical Society, respectively. | |
Electronic band structures and optical properties
WO3 is an n-type semiconductor with an indirect bandgap Eg characterizing the energy difference between the VB (EVB) and the CB (ECV), as shown in Fig. 9(a). The VB of WO3 is formed by filled O 2p-orbitals while the CB is composed mainly of empty W 5d-orbitals.94 The relationships between EVB, ECB and Eg follow the equations: (1) ECB = χ − Ee − 0.5Eg and (2) Eg = EVB − ECB, where χ is Mulliken's electronegativity of the material (6.59 eV for WO3) and Ee is the energy of a free electron on the hydrogen scale (4.5 eV).85 This indicates that the positions of the VB and the CB for a specific material are influenced directly by the bandgap Eg. Bulk WO3 has a typical Eg of 2.6 eV at room temperature, corresponding to a light absorption threshold at 477 nm determined by λ = 1240/Eg and EVB and ECB at +3.39 eV and +0.79 eV, respectively.95 This implies that the photogenerated holes in the VB of WO3 is highly oxidizing, which is strong enough to decompose water (E(O2/H2O) = +1.23 V vs. NHE) and almost all organic compounds, and/or react with water and surface hydroxyl (OH−) to produce ˙OH (E(˙OH/OH−) = +1.99 V, E(˙OH/H2O) = +2.72 V vs. NHE).96 However, the photogenerated electrons in the CB of WO3 are comparatively weak, which are not negative enough to photo-reduce H+ for H2 (E(H+/H2) = 0 V vs. NHE) and oxygen to O2˙− (E(O2˙−/O2) = −0.284 V vs. NHE).97 The Eg of WO3 is obviously affected by the phase transitions, which in turn is a function of temperature. In general, Eg decreases and becomes increasingly diffuse as the temperature increases, indicating a redshift of the light absorption edge.66 At the nanoscale, especially when the particle size is close to or smaller than the exciton Bohr radius of the material (∼3 nm for WO3),98Eg might increase significantly with decreasing particle size owing to the quantum confinement (QC) effect.99 The particle size effect on Eg can be estimated by Brus’ equation,100 as shown in Fig. 9(b).101 It indicates that the Eg of WO3 would be increased up to ∼3.0 eV when the particle size is reduced to ∼3 nm, implying that WO3 quantum dots (QDs, sizes smaller than the exciton Bohr radius) need UV light for excitation. The ECB of WO3 QDs would be lifted upwards due to the expansion of the bandgap, resulting in enhanced photo-reducing ability. An experimental measurement of bandgap expansion and obviously uplifted ECB of WO3 QDs has been reported by Watanabe et al., where the WO3 QDs with sizes at the sub-nano scale show Eg values up to 3.7 eV and achieve single-electron reduction of molecular oxygen.102
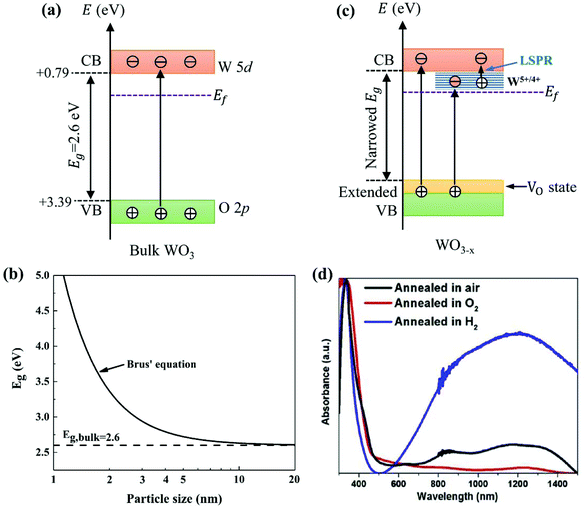 |
| Fig. 9 Electronic band structure of stoichiometric bulk WO3 (a), effect of the particle size on Eg for WO3 predicted by Brus’ equation with and ( and are the effective mass of an electron and a hole, respectively; m0 is the electron rest mass) (b),101 electronic band structure and electron excitation modes in sub-stoichiometric WO3−x (c), typical light absorptions of WOX with different extents of oxygen vacancies (d).38 Reprinted with permission from ref. 38. Copyright 2019 Elsevier. | |
Partial loss of oxygen from WO3 has similar consequences to the insertion of donors.103 Both experimental and simulation studies have revealed that the density of free charge carriers (ND) in WO3−x increases with the number of oxygen vacancies. As reported by Migas et al., ND increases from 2.90 × 1021 to 1.62 × 1022 cm−3 when the sub-stoichiometry is varied from WO2.92 to WO2.625.82 The introduction of oxygen vacancies would lead to partial reduction of WO3 (W6+ → W5+/4+) in order to match the charge balance. The presence of W5+/4+ creates new states closely below the CB of WO3 (W6+), as shown in Fig. 9(c). The injected electrons by oxygen vacancies would be firstly trapped in the W 5d-orbitals in the W5+/4+ sites, and then polarize the surrounding lattice to generate polarons.104 WO3−x has been reported to possibly absorb light ranging from the UV to near-infrared (NIR) regions due to three distinct modes of electron excitation: (i) VB-to-CB transition, (ii) VB-to-W5+/4+ state transition, and (iii) polaron-induced localized surface plasmon resonance (LSPR) (Fig. 9(c)).38 A typical light absorption of WO3−x was demonstrated by Kalanur et al., as shown in Fig. 9(d).38 The strong absorption in the visible and NIR regions (above 500 nm) has been ascribed to the third mode of electron excitation, i.e., the polaron-induced LSPR. The intensity of LSPR absorption has been proved to be correlated with ND, where a large ND generally leads to a strong LSPR absorption. Some recent reports also suggest that the oxygen deficiency results in the formation of oxygen-vacancy (VO) states above and partly overlap with the VB.105,106 This leads to an extension of the VB and narrows the band gap, which then expands the photo-response of WO3−x toward the longer wavelength range.
As for WOX·nH2O photocatalysts, they generally show smaller Eg than their dehydrated counterparts due to the weaker binding energy, thus exhibiting larger light absorption ranges.41,85 For instance, Ke et al. have synthesized WO3·H2O via a hydrothermal process and then obtained WO3 by calcining the as-synthesized sample at 500 °C.85 Their WO3·H2O and WO3 samples exhibit light absorption edges at 530 nm (Eg = 2.44 eV) and 472 nm (Eg = 2.64 eV), respectively.
Enhancement strategies
In order to improve the photocatalytic behaviours of WOX, many enhancement strategies have been reported. In this section, various enhancement strategies will be summarized from the aspects of the controls in the crystal phase, stoichiometry/oxygen-vacancy, active facet and morphology, elemental doping, loading of noble metal NPs, hybridization with carbon materials and coupling with other semiconductors to construct heterojunctions.
Phase control
Apart from m-WO3, nanostructured WO3 in hexagonal,42,48 orthorhombic107,108 and triclinic phases109,110 have also been reported to retain phase stability and exhibit photocatalytic activity at room temperature. Although some studies have reported that m-WO3 exhibits better photocatalytic behaviours than other phases of WO3,42,107 it is still difficult to make a conclusive comparison, for the photocatalytic efficiency of WOX is influenced simultaneously by many factors.
Recently, a phase junction photocatalyst constructed by different phases of the same semiconductor has attracted much attention due to its simplicity, controllability and great photocatalytic activity. WOX is a polymorphic semiconductor that consists of many crystal phases and abundant hydrates, which has offered great possibility to construct phase junctions between different phases. Some WOX-based phase junction photocatalysts have been reported, such as h-WO3·0.33H2O/c-WO3·0.5H2O,43 h-WO3/m-WO3
111 and o-WO3·0.33H2O/h-WO3.41 The enhancement mechanism depending on the phase junction is mainly due to the improved electron–hole separation between the different phases which show unequal band structures. A typical phase junction photocatalyst with the corresponding charge transfer mechanism is demonstrated in Fig. 10.
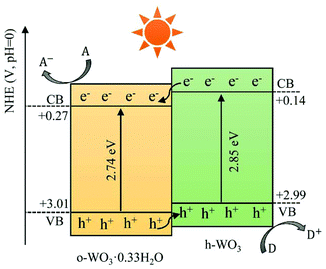 |
| Fig. 10 Schematic illustration of charge transfer and separation in a typical phase junction constructed by o-WO3·0.33H2O and h-WO3. | |
In general, a phase junction could be obtained by applying the intermediate synthesis conditions located between those for the synthesis of single-phase tungsten oxides. The mass ratio between the combined phases can be tuned by shifting the conditions toward the synthesis of the phase that is expected to increase. For instance, Li et al. have prepared a phase junction photocatalyst of o-WO3·0.33H2O/h-WO3via a hydrothermal method by adjusting the amount of NaCl (a capping agent).41 They obtained pure o-WO3·0.33H2O in the absence of NaCl and pure h-WO3 with the addition of 0.4 g NaCl. Their phase junction was obtained when NaCl was applied between 0.1 and 0.2 g and the mass ratio of o-WO3·0.33H2O to h-WO3 decreases when the amount of NaCl increases.
Stoichiometry/oxygen-vacancy control
In recent years, many efforts have been made to improve the photocatalytic efficiency of WOX by tuning its oxygen vacancies (e.g., number and distribution). Many studies have confirmed that the photocatalytic efficiency of WO3−x increases as the number of oxygen vacancies increases, due to enhanced optical absorption and reduced recombination rate of charge carriers. However, an over-abundance of oxygen vacancies (i.e., more than the optimal level) can act as recombination or trap centres for the photogenerated electrons and holes, thus lowering the photocatalytic activity.38,45 Some studies suggest that the distribution of oxygen vacancies (i.e., in bulk or on the surface) also matters to the photocatalytic activity, however, in different ways.44,45,112,113 Wang et al. have reported that bulk oxygen vacancies mainly promote visible light harvesting and slightly restrain the recombination of electrons and holes by narrowing the band gap, while the surface oxygen vacancies significantly increase the charge separation efficiency by lowering the VB edge.45
Oxygen vacancies in WO3 can be introduced by several means, including annealing under oxygen-deficient atmospheres (e.g., hydrogen45 and vacuum114), hydrogen peroxide treatment,112 etching and by specific routes.39,98,115 By annealing, which is the most common way, the extent and distribution of oxygen vacancies can be tuned by varying the temperature, duration and atmosphere. In general, the extent of oxygen vacancies increases as the reducibility of the atmosphere and the thermal treatment time increase. A moderate reductive atmosphere (e.g., 20% H2 in N2) is generally beneficial for generating surface oxygen vacancies, while thermal treatment under a highly reductive atmosphere (e.g., 100% H2) favours the generation of bulk oxygen vacancies. Oxygen vacancies generally propagate from the surface into the bulk as the thermal treatment proceeds. The oxygen vacancies can also be introduced by annealing in air, because, depending on the crystal structure and annealing temperature, the critical phase transition and nanoscale inhomogeneous deformation (during annealing) in the WO3 lattice can also create oxygen vacancies.105
Amongst the various oxygen-deficient tungsten oxides, W18O49 has attracted much attention due to its stable defect structure, strong LSPR absorption and good photocatalytic performance.116–118 W18O49 nanowires preferentially growing along the [010] direction have been frequently reported and applied to various photocatalytic reactions (e.g., CO2 reduction to CH4,119 degradation of organic dyes120 and H2 generation114). They have been synthesized by various methods, such as a solvothermal reaction followed by vacuum drying119 and solution combustion synthesis.120
Active-facet control
As photocatalytic reactions occur on the surface of the photocatalyst, the surface features (e.g., energy, atomic coordination and electronic structure) influence directly the overall photocatalytic reactivity. It has been widely accepted that high surface energy results in high photocatalytic reactivity due to the more active sites and stronger adsorption ability. From this point of view, {002} is the most active facet for both m- and o-WO3, for their surface energies follow the order: {002} (1.56 J m−2) > {020} (1.54 J m−2) > {200} (1.43 J m−2)121 and {002} (1.74 J m−2) > {020} = {200} (1.69 J m−2),122 respectively. This has been confirmed by several experimental measurements where both m- and o-WO3 with the preferentially exposed {002} facets exhibit better photocatalytic performance than their counterparts without the preferred orientation of the crystal facets.46,50,123,124 The properties of exposed facets have a close relationship with the crystal phase which they belong to. For h-WO3, both {002} and {200} have been reported to be active facets, the photocatalytic enhancement of which was ascribed to the increased charge separation efficiency.48,125 For o-WO3·0.33H2O, however, {020} was reported to be the most active facet, which has been said to be correlated with the unique W
O and O–H groups.47
Some studies suggest that the exposed facets have influences on the electronic structure, thus affecting the reduction and oxidation abilities of the photocatalyst. Xie et al. have prepared a quasi-cubic-like WO3 crystal with a nearly equal percentage of the {002}, {020} and {200} facets and a rectangular sheet-like WO3 crystal with the predominant {002} facet. Their study demonstrates that the former exhibits a deeper VB maximum, thus showing a much higher O2 evolution rate in photocatalytic water oxidation, while the latter exhibits an elevated CB minimum, and thus is able to photo-reduce CO2 to CH4.121
Hydrothermal/solvothermal synthesis has been commonly used to control the exposed facets of WOX. It is well established that solvents, impurities and additives in solution can substantially influence the ultimate shape of the crystals by controlling their growth rate in specific directions. For instance, Liang et al. have synthesized 2D ultra-thin m-WO3 nanosheets with more than 90% of the exposed {002} crystal facets via using a surfactant (Pluronic P123) as a capping agent.50 Since the surface energy of the {002} facet is much higher than that of the {020} and {200} facets, the polar groups of P123 preferentially adsorb onto the {002} facets, thus inhibiting their growth and finally promoting their exposure. Some inorganic salt anions, such as NO3−,121 BF4−,122 Cl− (ref. 46) and SO42−,48 have also been reported as effective capping agents for the preferential exposure of the {002} facets for m- and o-WO3 due to their preferential adsorption onto the {002} facets.
Morphology control
Morphology, mainly characterizing the features of shape and size, is one of the most important factors influencing the performance of photocatalysts. In general, a morphology that could offer a large specific surface area, large number of active sites, suitable pore features and short diffusion length for charge carriers is desirable.
Various unique morphologies of WOX photocatalysts have been reported in the literature; a brief summary can be found in Fig. 11. These morphologies can be classified into zero-dimensional (0D, e.g., spherical and pseudo-spherical NPs102,126–128), one-dimensional (1D, e.g., nanorods,120 nanowires,114 nanobelts,129 nanofibers130,131 and nanotubes23), two-dimensional (2D, e.g., nanoplatelets,132 nanoplates48 and nanosheets133) and three-dimensional (3D, e.g., porous interconnected structures,134,135 core–shell structures136–139 and hierarchical structures assembled by low-dimensional building blocks46,51,140–144) according to the dimensionality. A schematic illustration of simplified structures in different dimensionalities is demonstrated in Fig. 12(a). With a specific volume, particles in different shapes show different specific surface areas. A comparison of the specific surface area (S) between nanospheres, nanorods and nanosheets with different aspect ratios is demonstrated in Fig. 12(b). It indicates that, for both 1D nanorods and 2D nanosheets, the specific surface area increases as the aspect ratio increases. The 0D nanosphere shows the smallest specific surface area. It can be concluded that, if only considering the specific surface area, the preferability of morphology follows the order: 2D (high aspect ratio) > 1D (high aspect ratio) > 0D. However, it should always be kept in mind that a large specific surface area tends to result in severe agglomeration due to high specific surface energy, which in turn is not beneficial for the photocatalytic performance. Compromised strategies might be considered when developing efficient photocatalysts for practical applications. Apart from the shape, the size matters as well. In general, a smaller size results in a larger specific surface area and a shorter diffusion length for charge transfer, both of which are desirable for the enhancement of photocatalytic efficiency. When the particle size (at least in one dimension) is reduced to be close to or smaller than the exciton Bohr radius (∼3 nm for WO3), the band gap of the material would be increased significantly with the CB edge uplifted due to the strong QC effect.
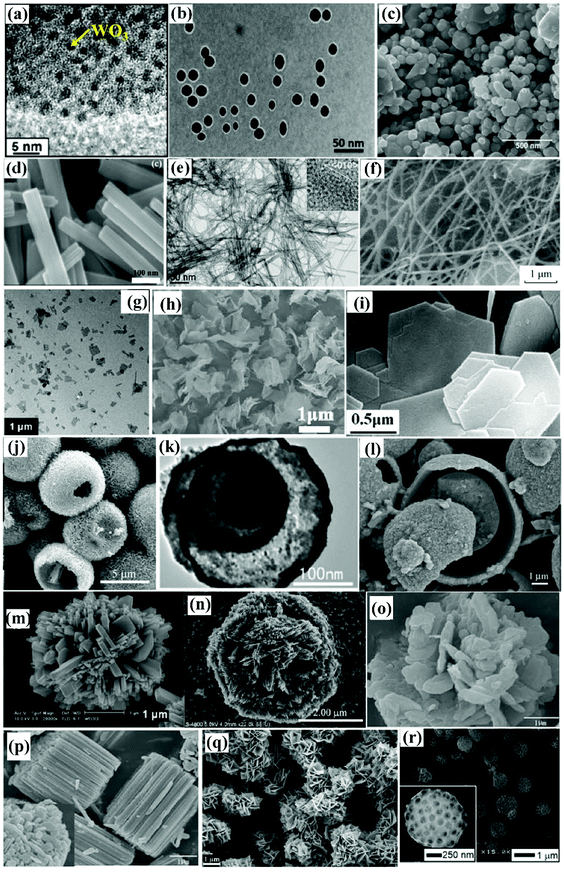 |
| Fig. 11 Typical morphologies of WOX photocatalysts from the literature: QDs (a),126 monodisperse nanoparticles (b),128 aggregated nanoparticles (c),42 nanorods (d),77 nanowires (e),98 nanofibers (f),131 nanosheets (g),133 nanosheets (h),50 nanoplates (i),48 hollow microspheres (j),137 multiple-shell hollow spheres (k),139 sphere-in-shell microstructures (l),138 hierarchical structures (m),141 flower-like microstructures (n),142 flower-like microstructures (o),143 cylindrical-stack microstructures (p),143 hierarchical structures (q),144 and 3D ordered macroporous structures (r).135 Reproduced with permission from ref. 42, 48, 50, 77, 98, 126, 128, 131, 133, 135, 137–139 and 141–144. Copyright 2010 the Royal Society of Chemistry, 2016, 2014 and 2017 Elsevier, 2018 American Chemical Society, 2015 Springer Nature, 2012 American Chemical Society, 2019, 2017 and 2008 Elsevier, 2012 and 2008 John Wiley and Sons, 2013, 2009, 2014, 2018 and 2013 Elsevier, respectively. | |
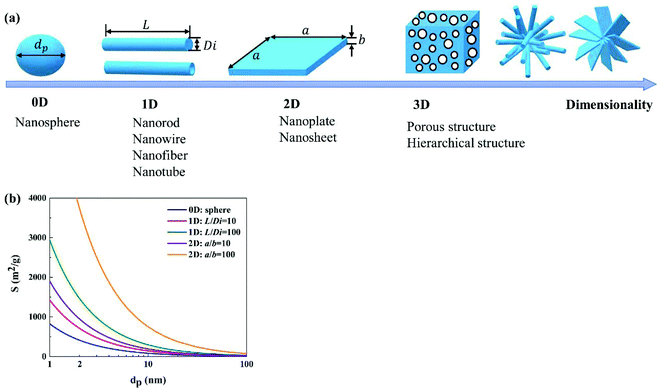 |
| Fig. 12 Schematic illustration of simplified morphologies in 0D, 1D, 2D and 3D (a), and the effect of the particle shape and size on the specific surface area for WO3 (b). | |
The morphology of WOX can be controlled by using a template-based method or a template-free method. With a template method, the shape and size of WOX are determined primarily by the structure of the template. Two such typical examples are WO3 QDs in macro/mesoporous silica102,126 and 3D ordered macroporous WO3.134,135 In a typical synthesis process, a precursor solution is simply introduced into template pores followed by particulation or chemical reactions for conversion to WOX. The template can be removed by using specific routes depending on the template material (e.g., calcination for removing the polymer template145) or just kept as a support for the WOX catalyst.102 With a template-free method, the shape and size of WOX can be tuned by varying the synthesis conditions (e.g., type and concentration of the capping agent, pH, and reaction temperature and time), which are influencing factors for the nucleation and growth rate of crystals in specific directions. For instance, Shukla et al. have synthesized monodisperse spherical WO3 NPs using cationic surfactants (i.e., cetylpyridinium chloride (CPyC), cetylpyridinium bromide (CPyB), hexadecyltrimethyl ammonium bromide (HTAC) and tetradecyltrimethyl ammonium bromide (TTAB)) as a capping agent.128 As these cationic surfactants adsorb non-selectively onto the surface of the WO3 nuclei, the final product of WO3 is in a spherical shape. When agents that can selectively adsorb onto specific faces of WO3 are applied, 1D and/or 2D structures might be obtained.
Elemental doping
Elemental doping is an effective way to tune the properties of photocatalysts. The incorporation of foreign ions into the lattice of WOX may result in changes in the crystal structure, morphology, electronic structure and optical properties depending on the nature and concentration of the dopant and the doping routes. The incorporated ions have two possible positions in the lattice of WOX, i.e., the W or O sites (substitution) and the interstice between WO6 octahedra (intercalation). The feasibility and extent of doping depend crucially on the differences in the radius and valence state between the dopant and host atoms. In general, a dopant with a similar radius to that of the host atom is easier for achieving doping and has a higher solubility in the host lattice, and simultaneously, resulting in lighter distortions of the host lattice and smaller changes in the morphology. Compared to anion doping (e.g., I− doping52), cation doping with low valence metal ions is much more frequently reported, such as Mo5+,146,147 Ta5+,148 Nb5+,149 Ti4+,150 Sn4+,151 Bi3+,152 Fe3+,153,154 Yb3+,155 Ce3+,156 La3+,156 Y3+,156 Co2+,157 Cu2+,158 Zn2+,159 Ni2+,160etc. In order to maintain the charge balance, oxygen vacancies are generally created when low valence metal ions are doped, which could result in extra benefit for the improvement of the photocatalytic performance. The doped metal ions on the surface of WOX could trap and localize electrons around them and enhance the photo-induced electron density on the active sites, so as to improve the electron-giving ability for photocatalytic reactions, e.g., CO2 reduction161 and N2 fixation.54
Elemental doping is commonly achieved by adding the starting material of the dopant (e.g. related ions or salts) into the precursor that is used for the synthesis of WOX. The extent of doping could be easily tuned by varying the addition amount of the dopant source. For instance, Wang et al. have performed Mo-doping into WO3·0.33H2O by adding Na2MoO4·2H2O into the Na2WO4·2H2O based precursor that is used for the hydrothermal synthesis of WO3·0.33H2O and modified the extent of doping by adjusting the stoichiometric ratio of Mo
:
W from 1% to 5%.161
Noble metal loading
In the past two decades, loading of noble metal NPs (e.g., Pt,162 Au163 and Ag164) has received increasing interest for the enhancement of the photocatalytic efficiency of WOX. WO3 has been thought to be unsuitable for the efficient oxidative decomposition of organic compounds in air or be limited to the reactions with strong electron acceptors, since its CB is not negative enough for the single-electron reduction of oxygen.40 In 2008, Abe et al. loaded Pt nanoparticles onto the surface of WO3 and found that the photogenerated electrons in Pt/WO3 could reduce O2 through multi-electron reduction ways (e.g., O2 + 2H+ + 2e− = H2O2 (aq), +0.682 V vs. NHE).40 In these processes, Pt works as an electron pool to accept the photogenerated electrons from WO3 and as a cocatalyst to facilitate the multi-electron reduction of O2 to produce H2O2. The study by Kim et al. has revealed that the reductive decomposition of H2O2 produced in situ from the reduction of O2 on the Pt/WO3 surface is another important path for the generation of ˙OH radicals, which is an important active species for the degradation of organic compounds.162 This enhancement mechanism has also been accepted for Au/WOX and Ag/WOX composites,56,165 the typical electron–hole transfer and separation process of which is demonstrated in Fig. 13(a).
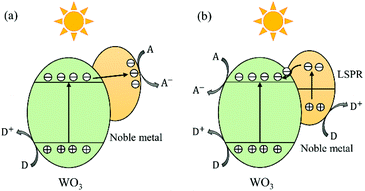 |
| Fig. 13 Schematic illustration of electron–hole transfer and separation in a noble metal loaded WO3 photocatalyst: the noble metal works as an electron pool (a) and the LSPR effect dominates (b). | |
The strong LSPR effect induced by noble metal NPs is another important factor contributing to the enhanced photocatalytic efficiency. Surface plasmon resonance (SPR) is a coherent oscillation of the surface conduction electrons excited by an electromagnetic radiation.166 For the case of LSPR, light interacts with particles much smaller than the incident wavelength.166 The plasmon frequency of a noble metal NP is correlated with its shape, size and proximity to other nanoparticles. Generally, decreasing the particle size can lead to a reduction in the plasmon frequency, i.e. resulting in a redshift of the plasmon resonance absorption.167 The noble metal NPs loaded on the WOX surface are generally smaller than 10 nm, corresponding to plasmon resonance absorptions in the visible and NIR regions. When the size of noble metal NPs is reduced to around 2 nm or less, the LSPR would disappear as the band structure becomes discontinuous and breaks down into discrete energy levels.167 Regarding the plasmonic enhancement in photocatalysis, two mechanisms have been frequently discussed: charge transfer and local electric field enhancement. The mechanism of charge transfer was firstly proposed by Tatsuma's group in 2004 for the study of Au- or Ag-loaded TiO2 systems.168 In this mechanism, the plasmon resonance excites electrons in noble metal NPs, which are then transferred to the CB of their adjacent semiconductors, namely the noble metal NPs act as electron-donors (see Fig. 13(b)). This charge transfer mechanism has also been accepted by some authors to explain the enhancement behaviour of noble metal/WOX photocatalysts.169 As for the mechanism of local electric field enhancement, studies have revealed that intense local electric fields near the surface of noble metal NPs could be generated by irradiating the NPs near their plasmon resonance frequency. Studies of electromagnetic simulations using the finite-difference time-domain (FDTD) method have shown that the electric field intensity of local plasmonic “hot spots” can reach as much as 1000 times that of the incident electric field.170 In these “hot spots”, the electron–hole pair generation rate is 1000 times that of the incident electromagnetic field. Thus, an increased amount of photoinduced charges is generated locally in the photocatalyst due to the local field enhancement of the plasmonic NPs. This local electric field mechanism has also been adopted by some authors to explain their developed efficient noble metal loaded WOX photocatalysts.
A uniform distribution of noble metal NPs on the surface of WOX is always desirable. An excess loading (i.e., more than the optimal level) may lead to agglomeration of the noble metal NPs, thus deteriorating the photocatalytic performance.169 The optimal loading depends on various factors. Even for the same noble metal, the optimal loading may vary significantly with the morphology of the as-prepared WOX.23,57 Nevertheless, the optimal loading of noble metal NPs on the WOX surface has always be reported to be less than 5 wt% in the literature.
A popular method for the deposition of noble metal NPs onto the WOX surface is photo-deposition. In a typical process, commercial or as-prepared WOX particles are added firstly into the aqueous solution of noble metal ions (e.g., AgNO3,171 H2PtCl6
57 and HAuCl4
56), which is then subjected to light irradiation for a certain period of time in the presence of an electron donor (generally methanol). The content and size of the loaded noble metal NPs could be tuned by varying the concentration of the noble metal ion in the solution and/or the intensity and time of the light irradiation. Apart from the in situ photo-deposition process, some authors prepared the noble metal colloidal solution firstly, and then immersed the WOX particles into the as-prepared noble metal colloidal solution. The final noble metal/WOX composite could be obtained by a post-heat treatment process.23
Hybridization with carbon materials
In the past decade, the coupling of WOX with carbon materials to form highly efficient composite photocatalysts has received increasing interest. Various carbon materials with unique structures have been adopted, such as graphene or reduced graphene oxide (RGO),58,172 carbon nanotube (CNT) or multi-walled carbon nanotube (MWCNT),59,173 carbon fiber172 and carbon nanodot.60 The carbon material is characterized by excellent electron mobility exceeding ∼15
000 m2 V−1 s−1, outstanding chemical and thermal stability and strong mechanical strength, which makes it a superior supporting matrix for photocatalysts. In a WOX/carbon hybrid under illumination, the photogenerated electrons produced in WOX could be transferred quickly to the carbon material through the interface, leaving photogenerated holes in WOX. The photogenerated electrons could then react with adsorbed electron-acceptors on the surface of the carbon material. In this way, efficient charge separation is achieved (see Fig. 14).
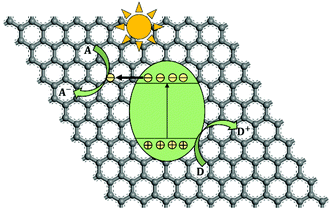 |
| Fig. 14 Schematic illustration of the charge transfer and separation in a WOX/carbon photocatalyst. | |
Amongst the various carbon materials, graphene and RGO (a single layer or multilayer of sp2 bonded carbon atoms with a honeycomb lattice structure) have attracted much attention due to their ultralight-weight and flexible feature and ultra-large specific surface area (∼2600 m2 g−1). RGO is usually obtained by the reduction of graphene oxide (GO), which is commonly prepared by the modified Hummers’ method, wherein graphite is used as a starting material and strongly oxidized during a grinding process.174,175 The oxidation introduces many oxygen-containing functional groups, such as epoxy, hydroxyl, carboxyl and carbonyl groups, on the carbon basal plane, making the obtained GO hydrophilic and easy to disperse stably in water. The oxygen-containing groups on the surface of GO are usually active sites for the growth or deposition of the WOX catalyst. According to the loading mechanism of WOX onto the surface of GO, the preparation of WOX/RGO composites can be categorized into two routes. One is that GO is added into the precursor that is used for the synthesis of WOX. With this route, WOX nucleates and grows on the surface of GO during the synthesis process (e.g., a hydrothermal treatment process).176 Another route is that WOX is firstly synthesized and then mixed with GO in solution followed by a specific treatment that allows the deposition of WOX onto the surface of GO. The GO in the as-prepared WOX/GO composite could then be reduced to RGO by a thermal decomposition or by specific reduction processes (e.g., a chemical reduction using hydrazine vapor at 90 °C for 24 h).177
Coupling with other semiconductors
Coupling WOX with other semiconductors having unequal band structures is an effective way to facilitate charge transfer and separation and to improve photo-induced redox ability. In the literature, various semiconductors (e.g., chalcogenides, halogenides, salts and carbon nitrides) have been reported to construct heterojunctions with WOX to form efficient photocatalysts, such as WOX/TiO2,178–180 WO3/Fe2O3,181,182 WO3/Cu2O,61,183 WO3/ZnO,96,184 WO3/CdS,185,186 WO3/Bi2S3,187,188 WO3/ZnIn2S2,189 WO3/AgI,190 WO3/BiOCl0.25Br0.75,191 WO3/BiOI,95 WO3/Ag3PO4,62,192 WO3/BiVO4,193,194 WO3/NiWO4,195 WO3/Bi2WO6,196,197 WOX/g-C3N4,198–200etc. For the ease of comparison, the electronic band structures of WO3 and its typical coupled semiconductors are summarized in Fig. 15. According to the mechanism of charge transfer, the WOX/semiconductor photocatalysts can be categorized into two groups: conventional type-II and Z-scheme (see Fig. 16). In a conventional type-II heterojunction, the photogenerated electrons transfer from the CB of the coupled semiconductor to that of WOX with the photogenerated holes migrating from the VB of WOX to that of the coupled semiconductor. Therefore, the photoreduction occurs on the surface of WOX while the photooxidation takes place on the surface of the coupled semiconductor. With this configuration, efficient spatial separation of electron–hole pairs could be obtained, however, the photo-oxidizing ability of the composite is decreased to some extent when compared to that of bare WOX. In a Z-scheme heterojunction, the photogenerated electrons from WOX recombine with the holes in the coupled semiconductor, while the holes in WOX and the electrons in the coupled semiconductor remain separated and reactive. In this case, the heterojunction retains the strong photo-oxidizing ability of WOX and possesses a higher photo-reducing ability imparted by the coupled semiconductor. As both types of the heterojunction have a staggered band structure, the charge transfer mechanism for a specific composite needs to be confirmed by experiment, e.g., detection of active species during a photocatalytic reaction. For some WOX/semiconductor systems (e.g., WO3/Cu2O, WO3/TiO2 and WO3/Fe2O3), the mechanism of charge transfer may alter depending on the synthesis routes and the applied photocatalytic reactions. For instance, Zhang et al. have prepared a WO3/Cu2O photoanode for PEC water splitting via a hydrothermal method followed by electrodeposition.183 The charge transfer in this heterojunction has been reported to follow the conventional type-II mode. However, in the study of Shi et al.61 where a WO3/Cu2O composite was synthesized using similar procedures but applied for CO2 reduction, the charge transfer was confirmed to follow the Z-scheme mechanism.
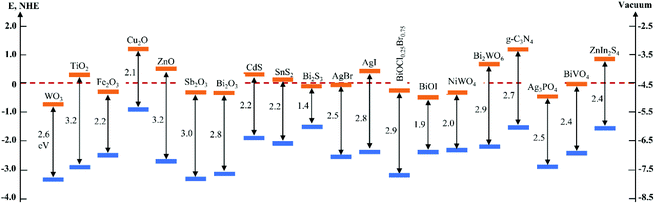 |
| Fig. 15 CB and VB energy levels of WO3 and a number of semiconductors. | |
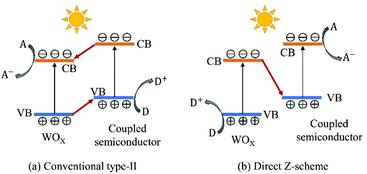 |
| Fig. 16 Schematic illustration of electron–hole transfer and separation in conventional type-II (a) and Z-scheme (b) WOX/semiconductor heterojunction photocatalysts. | |
Apart from the necessity of unequal band structures, intimate contact is another basic requirement for the efficient separation of charge carriers. In addition, the optimization of the contact surface area between WOX and the coupled semiconductor is also important to intensify the overall photocatalytic efficiency. This has been achieved by various unique morphology designs, such as the 0D/1D (e.g. Bi2WO6 NPs decorated on WO3 nanorods),197 0D/2D (e.g. BiVO4 NPs anchored on WO3 nanoplates),194 0D/3D (e.g. Ag3PO4 NPs dispersed in 3D ordered microporous WO3),201 1D/2D (e.g. W18O49 nanowires dispersed on g-C3N4 nanosheets)198 and 2D/2D (e.g. WO3 nanoplates on g-C3N4 nanosheets)63 structures, etc.
The construction of WOX/semiconductor heterojunctions could be achieved by a one-step or two-step preparation method. In a one-step method, the starting materials for both WOX and the coupled semiconductor are generally mixed in a precursor solution and then used for a synthesis process. For instance, Zhou et al. have prepared a WO3/BiWO6 composite via a one-step hydrothermal method using a precursor containing both Bi(NO3)3·5H2O and H2WO4.202 Compared to the one-step method, the two-step methods are more commonly adopted. In a two-step method, one semiconductor (i.e. WOX or the coupled semiconductor) is generally synthesized firstly and then mixed with the precursor solution that is used for the synthesis of the second semiconductor. The deposition and growth of the second semiconductor onto the surface of the first semiconductor could be achieved by using various synthesis methods. For instance, Ye and Wen have prepared a WO3/ZnIn2S4 composite by synthesizing firstly the WO3 nanorods via a hydrothermal process and then mixed the as-synthesized WO3 nanorods with the precursor solution containing In(NO3)3·4.5H2O, Zn(AC)2·6H2O and C3H7NO2S·HCl·H2O followed by another hydrothermal treatment, which allows the deposition and growth of ZnIn2S4 on the surface of WO3.189 The WOX and the coupled semiconductor could also be synthesized firstly and separately and then mixed in a solution followed by a specific treatment to allow intimate contact. For instance, Lara et al. have performed the coupling of WO3 and TiO2 by mixing the as-synthesized WO3 and TiO2 in a solution followed by a second-step hydrothermal treatment.179
Conclusions and perspectives
This review summarizes firstly the fundamentals of WOX (i.e. crystal and electronic structures and optical properties) and then highlights the strategies for the enhancement of the photocatalytic efficiency of WOX-based photocatalysts. These include the controls in the crystal phase, stoichiometry/oxygen-vacancy, active facet and morphology, elemental doping, loading of noble metal NPs, hybridization with carbon materials and coupling with other semiconductors to construct heterojunctions.
For nanostructured WOX, not only the monoclinic I phase but also the hexagonal, orthorhombic and triclinic phases could retain phase stability and exhibit photocatalytic activity at room temperature. Taking advantage of the polymorphic property of WOX, a phase junction could be formed to facilitate the transfer and separation of photogenerated charge carriers. Compared to stoichiometric WO3, oxygen-deficient WO3−x exhibits extended light absorption in the visible and NIR regions and possesses a higher density of free charge carriers, both of which are beneficial for improving the photocatalytic performance. {002} is the active facet of m- and o-WOX due to their high surface energy. A morphology with a high percentage of exposed active facets and large specific surface area is always desirable, which could be best achieved by a 2D structure with a high aspect ratio. The WOX with 3D hierarchical structures assembled by 1D and/or 2D building blocks and 3D ordered macroporous structures are also applausive for practical applications due to their high structural stability and less agglomeration. Doping WOX with low valence metal ions could extend light absorption, promote photo-induced electron–hole separation and improve photocatalytic redox ability due to the increased oxygen vacancies and the introduced defect band. By loading noble metal NPs onto the surface of WOX, it is possible to extend light absorption into the visible and NIR regions and provide large numbers of “hot electrons” to participate in photocatalytic reactions, which is due to the strong LSPR effect. Graphene is an ideal support for the WOX photocatalyst, for it provides an ultra-large specific surface area and serves as an excellent conductor for photogenerated electrons migrating from WOX. Coupling WOX with other semiconductors having unequal band structures could achieve efficient spatial charge separation via facilitating the charge transfer through the interfaces. By the construction of a Z-scheme heterojunction, the strong photo-oxidation ability of WOX can be retained while the photo-reduction ability could be enhanced because of the higher CB level of the coupled semiconductor.
However, the photocatalytic efficiency of WOX-based photocatalysts is still far from expectation, further improvement of which might rely on controls in synergistic effects. Combining different strategies (e.g. controls in the crystal phase, stoichiometry, active facet and morphology and coupling with other materials) in unique ways might lead to incredible results that could not be obtained with single strategies. To achieve the effective control of synergistic effects, the mechanisms of single strategies should be clear, some of which, however, are still vague. For instance, the charge transfer mechanism in the noble metal loaded system is still inconsistent. Furthermore, theoretical guidelines for the construction of Z-scheme heterojunctions are still lacking. The final aim of the development of efficient photocatalysts is to serve human beings by solving practical problems. However, the research of WOX-based photocatalysts is still limited to laboratories. The efforts contributing to exploit appropriate ways for practical applications of WOX-based photocatalysts, e.g., design of photo-reactors and integration with other technologies to extend applications, should be highlighted in the future.
Conflicts of interest
There are no conflicts to declare.
Acknowledgements
This work was partly supported by the Ministry of Science and Technology of China (2016YFB0303901-05). Y. Gao acknowledges the funding from the Changjiang Scholars Programs (T2015136). W. Wang acknowledges the National Natural Science Foundation of China (51772312, 21671197).
References
- A. A. Ismail and D. W. Bahnemann, Photochemical splitting of water for hydrogen production by photocatalysis: A review, Sol. Energy Mater. Sol. Cells, 2014, 128, 85–101 CrossRef CAS.
- H. Ahmad, S. K. Kamarudin, L. J. Minggu and M. Kassim, Hydrogen from photo-catalytic water splitting process: A review, Renewable Sustainable Energy Rev., 2015, 43, 599–610 CrossRef CAS.
- S. Sun, X. Zhang, X. Liu, L. Pan, X. Zhang and J. Zou, Design and Construction of Cocatalysts for Photocatalytic Water Splitting, Acta Phys.-Chim. Sin., 2020, 36, 1905007 Search PubMed.
- X. Xu, L. Pan, X. Zhang, L. Wang and J. Zou, Rational Design and Construction of Cocatalysts for Semiconductor-Based Photo-Electrochemical Oxygen Evolution: A Comprehensive Review, Adv. Sci., 2019, 6, 1801505 CrossRef PubMed.
- Y. C. Zhang, N. Afzal, L. Pan, X. Zhang and J. J. Zou, Structure-Activity Relationship of Defective Metal-Based Photocatalysts for Water Splitting: Experimental and Theoretical Perspectives, Adv. Sci., 2019, 6, 1900053 CrossRef PubMed.
- S. Sun, G. Shen, J. Jiang, W. Mi, X. Liu, L. Pan, X. Zhang and J. J. Zou, Boosting Oxygen Evolution Kinetics by Mn–N–C Motifs with Tunable Spin State for Highly Efficient Solar-Driven Water Splitting, Adv. Energy Mater., 2019, 9, 1901505 CrossRef.
- O. Ola and M. M. Maroto-Valer, Review of material design and reactor engineering on TiO2 photocatalysis for CO2 reduction, J. Photochem. Photobiol., C, 2015, 24, 16–42 CrossRef CAS.
- N. Shehzad, M. Tahir, K. Johari, T. Murugesan and M. Hussain, A critical review on TiO2 based photocatalytic CO2 reduction system: Strategies to improve efficiency, J. CO2 Util., 2018, 26, 98–122 CrossRef CAS.
- X. Chen, N. Li, Z. Kong, W. Ong and X. Zhao, Photocatalytic fixation of nitrogen to ammonia: state-of-the-art advancements and future prospects, Mater. Horiz., 2018, 5, 9–27 RSC.
- M. N. Chong, B. Jin, C. W. K. Chow and C. Saint, Recent developments in photocatalytic water treatment technology: A review, Water Res., 2010, 44, 2997–3027 CrossRef CAS PubMed.
- H. Zangeneh, A. A. L. Zinatizadeh, M. Habibi, M. Akia and M. Hasnain Isa, Photocatalytic oxidation of organic dyes and pollutants in wastewater using different modified titanium dioxides: A comparative review, J. Ind. Eng. Chem., 2015, 26, 1–36 CrossRef CAS.
- Y. Boyjoo, H. Sun, J. Liu, V. K. Pareek and S. Wang, A review on photocatalysis for air treatment: From catalyst development to reactor design, Chem. Eng. J., 2017, 310, 537–559 CrossRef CAS.
- Z. Shayegan, C. Lee and F. Haghighat, TiO2 photocatalyst for removal of volatile organic compounds in gas phase-A review, Chem. Eng. J., 2018, 334, 2408–2439 CrossRef CAS.
- M. Cheng, G. Zeng, D. Huang, C. Lai, P. Xu, C. Zhang and Y. Liu, Hydroxyl radicals based advanced oxidation processes (AOPs) for remediation of soils contaminated with organic compounds: A review, Chem. Eng. J., 2016, 284, 582–598 CrossRef CAS.
- S. Banerjee, D. D. Dionysiou and S. C. Pillai, Self-cleaning applications of TiO2 by photo-induced hydrophilicity and photocatalysis, Appl. Catal., B, 2015, 176–177, 396–428 CrossRef CAS.
- P. Ganguly, C. Byrne, A. Breen and S. C. Pillai, Antimicrobial activity of photocatalysts: Fundamentals, mechanisms, kinetics and recent advances, Appl. Catal., B, 2018, 225, 51–75 CrossRef CAS.
- C. Zhang, Y. Li, D. Shuai, Y. Shen and D. Wang, Progress and challenges in photocatalytic disinfection of waterborne Viruses: A review to fill current knowledge gaps, Chem. Eng. J., 2019, 355, 399–415 CrossRef CAS.
- A. L. Linsebigler, G. Lu and J. T. Yates, Photocatalysis on TiO2 Surfaces: Principles, Mechanisms, and Selected Results, Chem. Rev., 1995, 95, 735–758 CrossRef CAS.
- K. Nakata and A. Fujishima, TiO2 photocatalysis: Design and applications, J. Photochem. Photobiol., C, 2012, 13, 169–189 CrossRef CAS.
- J. Low, J. Yu, M. Jaroniec, S. Wageh and A. A. Al-Ghamdi, Heterojunction Photocatalysts, Adv. Mater., 2017, 29, 1601694 CrossRef PubMed.
- A. Fujishima, T. N. Rao and D. A. Tryk, Titanium dioxide photocatalysis, J. Photochem. Photobiol., C, 2000, 1, 1–21 CrossRef CAS.
- A. Fujishima, X. Zhang and D. Tryk, TiO2 photocatalysis and related surface phenomena, Surf. Sci. Rep., 2008, 63, 515–582 CrossRef CAS.
- Z. Zhao and M. Miyauchi, Nanoporous-Walled Tungsten Oxide Nanotubes as Highly Active Visible-Light-Driven Photocatalysts, Angew. Chem., Int. Ed., 2008, 47, 7051–7055 CrossRef CAS PubMed.
- H. Irie, Y. Watanabe and K. Hashimoto, Carbon-doped Anatase TiO2 Powders as a Visible-light Sensitive Photocatalyst, Chem. Lett., 2003, 32, 772–773 CrossRef CAS.
- T. Ohno, T. Mitsui and M. Matsumura, Photocatalytic Activity of S-doped TiO2 Photocatalyst under Visible Light, Chem. Lett., 2003, 32, 364–365 CrossRef CAS.
- V. Etacheri, C. Di Valentin, J. Schneider, D. Bahnemann and S. C. Pillai, Visible-light activation of TiO2 photocatalysts: Advances in theory and experiments, J. Photochem. Photobiol., C, 2015, 25, 1–29 CrossRef CAS.
- S. Sakthivel, M. Janczarek and H. Kisch, Visible Light Activity and Photoelectrochemical Properties of Nitrogen-Doped TiO2, J. Phys. Chem. B, 2004, 108, 19384–19387 CrossRef CAS.
- S. G. Ullattil, S. B. Narendranath, S. C. Pillai and P. Periyat, Black TiO2 Nanomaterials: A Review of Recent Advances, Chem. Eng. J., 2018, 343, 708–736 CrossRef CAS.
- P. Dong, G. Hou, X. Xi, R. Shao and F. Dong, WO3-based photocatalysts: morphology control, activity enhancement and multifunctional applications, Environ. Sci.: Nano, 2017, 4, 539–557 RSC.
- M. Mishra and D. Chun, α-Fe2O3 as a photocatalytic material: A review, Appl. Catal., A, 2015, 498, 126–141 CrossRef CAS.
- N. Bao, L. Shen, T. Takata and K. Domen, Self-Templated Synthesis of Nanoporous CdS Nanostructures for Highly Efficient Photocatalytic Hydrogen Production under Visible Light, Chem. Mater., 2008, 20, 110–117 CrossRef CAS.
- S. Tokunaga, H. Kato and A. Kudo, Selective Preparation of Monoclinic and Tetragonal BiVO4 with Scheelite Structure and Their Photocatalytic Properties, Chem. Mater., 2001, 13, 4624–4628 CrossRef CAS.
- H. Fu, C. Pan, W. Yao and Y. Zhu, Visible-Light-Induced Degradation of Rhodamine B by Nanosized Bi2WO6, J. Phys. Chem. B, 2005, 109, 22432–22439 CrossRef CAS PubMed.
- M. Shang, W. Wang, S. Sun, L. Zhou and L. Zhang, Bi2WO6 Nanocrystals with High Photocatalytic Activities under Visible Light, J. Phys. Chem. C, 2008, 112, 10407–10411 CrossRef CAS.
- Z. Yi, J. Ye, N. Kikugawa, T. Kako, S. Ouyang, H. Stuart-Williams, H. Yang, J. Cao, W. Luo, Z. Li, Y. Liu and R. L. Withers, An orthophosphate semiconductor with photooxidation properties under visible-light irradiation, Nat. Mater., 2010, 9, 559–564 CrossRef CAS PubMed.
- J. Wen, J. Xie, X. Chen and X. Li, A review on g-C3N4-based photocatalysts, Appl. Surf. Sci., 2017, 391, 72–123 CrossRef CAS.
- Z. Huang, J. Song, L. Pan, X. Zhang, L. Wang and J. Zou, Tungsten Oxides for Photocatalysis, Electrochemistry, and Phototherapy, Adv. Mater., 2015, 27, 5309–5327 CrossRef CAS PubMed.
- S. S. Kalanur, I. Yoo, I. Cho and H. Seo, Effect of oxygen vacancies on the band edge properties of WO3 producing enhanced photocurrents, Electrochim. Acta, 2019, 296, 517–527 CrossRef CAS.
- T. Soltani, A. Tayyebi and B. Lee, Sonochemical-driven ultrafast facile synthesis of WO3 nanoplates with controllable morphology and oxygen vacancies for efficient photoelectrochemical water splitting, Ultrason. Sonochem., 2019, 50, 230–238 CrossRef CAS PubMed.
- R. Abe, H. Takami, N. Murakami and B. Ohtani, Pristine Simple Oxides as Visible Light Driven Photocatalysts: Highly Efficient Decomposition of Organic Compounds over Platinum-Loaded Tungsten Oxide, J. Am. Chem. Soc., 2008, 130, 7780–7781 CrossRef CAS PubMed.
- Y. Li, Z. Tang, J. Zhang and Z. Zhang, Fabrication of vertical orthorhombic/hexagonal tungsten oxide phase junction with high photocatalytic performance, Appl. Catal., B, 2017, 207, 207–217 CrossRef CAS.
- D. B. Hernandez-Uresti, D. Sánchez-Martínez, A. Martínez-de La Cruz, S. Sepúlveda-Guzmán and L. M. Torres-Martínez, Characterization and photocatalytic properties of hexagonal and monoclinic WO3 prepared via microwave-assisted hydrothermal synthesis, Ceram. Int., 2014, 40, 4767–4775 CrossRef CAS.
- Y. Kong, H. Sun, X. Zhao, B. Gao and W. Fan, Fabrication of hexagonal/cubic tungsten oxide homojunction with improved photocatalytic activity, Appl. Catal., A, 2015, 505, 447–455 CrossRef CAS.
- R. Zhang, F. Ning, S. Xu, L. Zhou, M. Shao and M. Wei, Oxygen vacancy engineering of WO3 toward largely enhanced photoelectrochemical water splitting, Electrochim. Acta, 2018, 274, 217–223 CrossRef CAS.
- Y. Wang, J. Cai, M. Wu, J. Chen, W. Zhao, Y. Tian, T. Ding, J. Zhang, Z. Jiang and X. Li, Rational construction of oxygen vacancies onto tungsten trioxide to improve visible light photocatalytic water oxidation reaction, Appl. Catal., B, 2018, 239, 398–407 CrossRef CAS.
- J. Zhang, P. Zhang, T. Wang and J. Gong, Monoclinic WO3 nanomultilayers with preferentially exposed (002) facets for photoelectrochemical water splitting, Nano Energy, 2015, 11, 189–195 CrossRef CAS.
- Y. Li, Z. Tang, J. Zhang and Z. Zhang, Exposed facet and crystal phase tuning of hierarchical tungsten oxide nanostructures and their enhanced visible-light-driven photocatalytic performance, CrystEngComm, 2015, 17, 9102–9110 RSC.
- X. Wang, H. Fan and P. Ren, Effects of exposed facets on photocatalytic properties of WO3, Adv. Powder Technol., 2017, 28, 2549–2555 CrossRef CAS.
- M. Aslam, I. M. I. Ismail, S. Chandrasekaran and A. Hameed, Morphology controlled bulk synthesis of disc-shaped WO3 powder and evaluation of its photocatalytic activity for the degradation of phenols, J. Hazard. Mater., 2014, 276, 120–128 CrossRef CAS PubMed.
- Y. Liang, Y. Yang, C. Zou, K. Xu, X. Luo, T. Luo, J. Li, Q. Yang, P. Shi and C. Yuan, 2D ultra-thin WO3 nanosheets with dominant {002} crystal facets for high-performance xylene sensing and methyl orange photocatalytic degradation, J. Alloys Compd., 2019, 783, 848–854 CrossRef CAS.
- Z. Wang, D. Chu, L. Wang, L. Wang, W. Hu, X. Chen, H. Yang and J. Sun, Facile synthesis of hierarchical double-shell WO3 microspheres with enhanced photocatalytic activity, Appl. Surf. Sci., 2017, 396, 492–496 CrossRef CAS.
- J. O. Tijani, O. Ugochukwu, L. A. Fadipe, M. T. Bankole, A. S. Abdulkareem and W. D. Roos, Photocatalytic degradation of local dyeing wastewater
by iodine-phosphorus co-doped tungsten trioxide nanocomposites under natural sunlight irradiation, J. Environ. Manage., 2019, 236, 519–533 CrossRef CAS PubMed.
- S. S. Kalanur, I. Yoo, I. S. Cho and H. Seo, Niobium incorporated WO3 nanotriangles: Band edge insights and improved photoelectrochemical water splitting activity, Ceram. Int., 2019, 45, 8157–8165 CrossRef CAS.
- Z. Ying, S. Chen, S. Zhang, T. Peng and R. Li, Efficiently enhanced N2 photofixation performance of sea-urchin-like W18O49 microspheres with Mn-doping, Appl. Catal., B, 2019, 254, 351–359 CrossRef CAS.
- Y. Liu, Z. Zhang, Y. Fang, B. Liu, J. Huang, F. Miao, Y. Bao and B. Dong, IR-Driven strong plasmonic-coupling on Ag nanorices/W18O49 nanowires heterostructures for photo/thermal synergistic enhancement of H2 evolution from ammonia borane, Appl. Catal., B, 2019, 252, 164–173 CrossRef CAS.
- D. P. DePuccio, P. Botella, B. O. Rourke and C. C. Landry, Degradation of Methylene Blue Using Porous WO3, SiO2-WO3, and Their Au-Loaded Analogs: Adsorption and Photocatalytic Studies, ACS Appl. Mater. Interfaces, 2015, 7, 1987–1996 CrossRef CAS PubMed.
- H. Widiyandari, A. Purwanto, R. Balgis, T. Ogi and K. Okuyama, CuO/WO3 and Pt/WO3 nanocatalysts for efficient pollutant degradation using visible light irradiation, Chem. Eng. J., 2012, 180, 323–329 CrossRef CAS.
- L. Tie, C. Yu, Y. Zhao, H. Chen, S. Yang, J. Sun, S. Dong and J. Sun, Fabrication of WO3 nanorods on reduced graphene oxide sheets with augmented visible light photocatalytic activity for efficient mineralization of dye, J. Alloys Compd., 2018, 769, 83–91 CrossRef CAS.
- W. Zhu, Z. Li, C. He, S. Faqian and Y. Zhou, Enhanced photodegradation of sulfamethoxazole by a novel WO3-CNT composite under visible light irradiation, J. Alloys Compd., 2018, 754, 153–162 CrossRef CAS.
- J. Zhang, Y. Ma, Y. Du, H. Jiang, D. Zhou and S. Dong, Carbon nanodots/WO3 nanorods Z-scheme composites: Remarkably enhanced photocatalytic performance under broad spectrum, Appl. Catal., B, 2017, 209, 253–264 CrossRef CAS.
- W. Shi, X. Guo, C. Cui, K. Jiang, Z. Li, L. Qu and J. Wang, Controllable synthesis of Cu2O decorated WO3 nanosheets with dominant (0 0 1) facets for photocatalytic CO2 reduction under visible-light irradiation, Appl. Catal., B, 2019, 243, 236–242 CrossRef CAS.
- X. Liu, J. Xu, Z. Ni, R. Wang, J. You and R. Guo, Adsorption and visible-light-driven photocatalytic properties of Ag3PO4/WO3 composites: A discussion of the mechanism, Chem. Eng. J., 2019, 356, 22–33 CrossRef CAS.
- J. Fu, Q. Xu, J. Low, C. Jiang and J. Yu, Ultrathin 2D/2D WO3/g-C3N4 step-scheme H2-production photocatalyst, Appl. Catal., B, 2019, 243, 556–565 CrossRef CAS.
- T. Zhu, M. N. Chong and E. S. Chan, Nanostructured Tungsten Trioxide Thin Films Synthesized for Photoelectrocatalytic Water Oxidation: A review, ChemSusChem, 2014, 7, 2974–2997 CrossRef CAS PubMed.
- M. B. Tahir, G. Nabi, M. Rafique and N. R. Khalid, Nanostructured-based WO3 photocatalysts: recent development, activity enhancement, perspectives and applications for wastewater treatment, Int. J. Environ. Sci. Technol., 2017, 14, 2519–2542 CrossRef CAS.
- P. M. Woodward, A. W. Sleight and T. Vogt, Ferroelectric Tungsten Trioxide, J. Solid State Chem., 1997, 131, 9–17 CrossRef CAS.
- F. Corà, A. Patel, N. M. Harrison, R. Dovesi and C. R. A. Catlow, An ab Initio Hartree–Fock Study of the Cubic and Tetragonal Phases of Bulk Tungsten Trioxide, J. Am. Chem. Soc., 1996, 118, 12174–12182 CrossRef.
- R. Diehl, G. Brandt and E. Salje, The crystal structure of triclinic WO3, Acta Crystallogr., Sect. B: Struct. Crystallogr. Cryst. Chem., 1978, 34, 1105–1111 CrossRef.
- E. Salje, The orthorhombic phase of WO3, Acta Crystallogr., Sect. B: Struct. Crystallogr. Cryst. Chem., 1977, 33, 574–577 CrossRef.
- W. L. Kehl, R. G. Hay and D. Wahl, The Structure of Tetragonal Tungsten Trioxide, J. Appl. Phys., 1952, 23, 212–215 CrossRef CAS.
- S. Tanisaki, Crystal Structure of Monoclinic Tungsten Trioxide at Room Temperature, J. Phys. Soc. Jpn., 1960, 15, 573–581 CrossRef CAS.
- K. R. Locherer, I. P. Swainson and E. K. H. Salje, Transition to a new tetragonal phase of WO3: crystal structure and distortion parameters, J. Phys.: Condens. Matter, 1999, 11, 4143–4156 CrossRef CAS.
- T. Vogt, P. M. Woodward and B. A. Hunter, The High-Temperature Phases of WO3, J. Solid State Chem., 1999, 144, 209–215 CrossRef CAS.
- H. Zheng, J. Z. Ou, M. S. Strano, R. B. Kaner, A. Mitchell and K. Kalantar-zadeh, Nanostructured Tungsten Oxide-Properties, Synthesis, and Applications, Adv. Funct. Mater., 2011, 21, 2175–2196 CrossRef CAS.
- S. Adhikari, R. Swain, D. Sarkar and G. Madras, Wedge-like WO3 architectures for efficient electrochromism and photoelectrocatalytic activity towards water pollutants, Mol. Catal., 2017, 432, 76–87 CrossRef CAS.
- K. Hayat, M. A. Gondal, M. M. Khaled, Z. H. Yamani and S. Ahmed, Laser induced photocatalytic degradation of hazardous dye (Safranin-O) using self synthesized nanocrystalline WO3, J. Hazard. Mater., 2011, 186, 1226–1233 CrossRef CAS PubMed.
- S. Yao, F. Qu, G. Wang and X. Wu, Facile hydrothermal synthesis of WO3 nanorods for photocatalysts and supercapacitors, J. Alloys Compd., 2017, 724, 695–702 CrossRef CAS.
- B. Gerand, G. Nowogrocki, J. Guenot and M. Figlarz, Structural Study of a New Hexagonal Form of Tungsten Trioxide, J. Solid State Chem., 1979, 29, 429–434 CrossRef CAS.
- B. G. Hyde, A. N. Bagshaw, S. Andersson and M. O'Keeffe, Some Defect Structures in Crystalline Solids, Annu. Rev. Mater. Sci., 1974, 4, 43–49 CrossRef CAS.
- A. Magnéli, Structures of the ReO3-type with recurrent dislocations of atoms: ‘homologous series’ of molybdenum and tungsten oxides, Acta Crystallogr., 1953, 6, 495–500 CrossRef.
- M. Lundberg, M. Sundberg and A. Magneli, The “Pentagonal Column” as a Building Unit in Crystal and Defect Structures of Some Groups of Transition Metal Compounds, J. Solid State Chem., 1982, 44, 32–40 CrossRef CAS.
- D. B. Migas, V. L. Shaposhnikov and V. E. Borisenko, Tungsten oxides. II. The metallic nature of Magnéli phases, J. Appl. Phys., 2010, 108, 093714 CrossRef.
- X. Fang, M. Yao, L. Guo, Y. Xu, W. Zhou, M. Zhuo, C. Shi, L. Liu, L. Wang, X. Li and W. Chen, One-Step, Solventless, and Scalable Mechanosynthesis of WO3·2H2O Ultrathin Narrow Nanosheets with Superior UV-Vis-Light-Driven Photocatalytic Activity, ACS Sustainable Chem. Eng., 2017, 5, 10735–10743 CrossRef CAS.
- Q. Zeng, Y. Zhao, J. Zhao, X. Hao, Y. Lu, J. Guo, Y. Song, F. Gao and Z. Huang, Studies on fabrication of urchin-like WO3·H2O hollow spheres and their photocatalytic properties, Cryst. Res. Technol., 2013, 48, 334–343 CrossRef CAS.
- J. Ke, H. Zhou, J. Liu, X. Duan, H. Zhang, S. Liu and S. Wang, Crystal transformation of 2D tungstic acid H2WO4 to WO3 for enhanced photocatalytic water oxidation, J. Colloid Interface Sci., 2018, 514, 576–583 CrossRef CAS PubMed.
- X. Wang, X. Meng, M. Zhong, F. Wu and J. Li, Hydrothermal synthesis of WO3·0.5H2O microtubes with excellent photocatalytic properties, Appl. Surf. Sci., 2013, 282, 826–831 CrossRef CAS.
- X. Song, C. Wang, W. Wang, X. Zhang, N. Hou and H. Yu, A Dissolution-Regeneration Route to Synthesize Blue Tungsten Oxide Flowers and their Applications in Photocatalysis and Gas Sensing, Adv. Mater. Interfaces, 2016, 3, 1500417 CrossRef.
- Y. Tanaka, M. Miyayama, M. Hibino and T. Kudo, Preparation and proton conductivity of WO3·2H2O/epoxy composite films, Solid State Ionics, 2004, 171, 33–39 CrossRef CAS.
- L. Li, J. Zhao, Y. Wang, Y. Li, D. Ma, Y. Zhao, S. Hou and X. Hao, Oxalic acid mediated synthesis of WO3·H2O nanoplates and self-assembled nanoflowers under mild conditions, J. Solid State Chem., 2011, 184, 1661–1665 CrossRef CAS.
- J. Yang, W. Li, J. Li, D. Sun and Q. Chen, Hydrothermal synthesis and photoelectrochemical properties of vertically aligned tungsten trioxide (hydrate) plate-like arrays fabricated directly on FTO substrates, J. Mater. Chem., 2012, 22, 17744 RSC.
- J. R. Gunter, M. Amberg and H. Schmalle, Direct synthesis and single crystal structure determination of cubic pyrochlore-type tungsten trioxide hemihydrate, WO3·0.5H2O, Mater. Res. Bull., 1989, 24, 289–292 CrossRef.
- S. S. Kalanur and H. Seo, Intercalation of barium into monoclinic tungsten oxide nanoplates for enhanced photoelectrochemical water splitting, Chem. Eng. J., 2019, 355, 784–796 CrossRef CAS.
- L. Zhou, J. Zou, M. Yu, P. Lu, J. Wei, Y. Qian, Y. Wang and C. Yu, Green Synthesis of Hexagonal-Shaped WO3·0.33H2O Nanodiscs Composed of Nanosheets, Cryst. Growth Des., 2008, 8, 3993–3998 CrossRef CAS.
- S. Cong, F. Geng and Z. Zhao, Tungsten Oxide Materials for Optoelectronic Applications, Adv. Mater., 2016, 28, 10518–10528 CrossRef CAS PubMed.
- J. Luo, X. Zhou, L. Ma and X. Xu, Enhanced visible-light-driven photocatalytic activity of WO3/BiOI heterojunction photocatalysts, J. Mol. Catal. A: Chem., 2015, 410, 168–176 CrossRef CAS.
- R. Lei, H. Zhang, H. Ni, R. Chen, H. Gu and B. Zhang, Novel ZnO nanoparticles modified WO3 nanosheet arrays for enhanced photocatalytic properties under solar light illumination, Appl. Surf. Sci., 2019, 463, 363–373 CrossRef CAS.
- X. Chen, S. Shen, L. Guo and S. S. Mao, Semiconductor-based Photocatalytic Hydrogen Generation, Chem. Rev., 2010, 110, 6503–6570 CrossRef CAS PubMed.
- T. Paik, M. Cargnello, T. R. Gordon, S. Zhang, H. Yun, J. D. Lee, H. Y. Woo, S. J. Oh, C. R. Kagan, P. Fornasiero and C. B. Murray, Photocatalytic Hydrogen Evolution from Substoichiometric Colloidal WO3−x Nanowires, ACS Energy Lett., 2018, 3, 1904–1910 CrossRef CAS.
- S. K. Gullapalli, R. S. Vemuri and C. V. Ramana, Structural transformation induced changes in the optical properties of nanocrystalline tungsten oxide thin films, Appl. Phys. Lett., 2010, 96, 171903 CrossRef.
- L. Brus, Electronic wave functions in semiconductor clusters: experiment and theory, J. Phys. Chem., 1986, 90, 2555–2560 CrossRef CAS.
- W. Yu, J. Chen, T. Shang, L. Chen, L. Gu and T. Peng, Direct Z-scheme g-C3N4-WO3 photocatalyst with atomically defined junction for H2 production, Appl. Catal., B, 2017, 219, 693–704 CrossRef CAS.
- H. Watanabe, K. Fujikata, Y. Oaki and H. Imai, Band-gap expansion of tungsten oxide quantum dots synthesized in sub-nano porous silica, Chem. Commun., 2013, 49, 8477–8479 RSC.
- A. Polaczek, M. Pekala and Z. Obuszko, Magnetic susceptibility and thermoelectric power of tungsten intermediary oxides, J. Phys.: Condens. Matter, 1994, 6, 7909–7919 CrossRef CAS.
- S. K. Deb, Opportunities and challenges in science and technology of WO3 for electrochromic and related applications, Sol. Energy Mater. Sol. Cells, 2008, 92, 245–258 CrossRef CAS.
- Y. Li, Z. Tang, J. Zhang and Z. Zhang, Defect Engineering of Air-Treated WO3 and Its Enhanced Visible-Light-Driven Photocatalytic and Electrochemical Performance, J. Phys. Chem. C, 2016, 120, 9750–9763 CrossRef CAS.
- G. Hai, J. Huang, L. Cao, Y. Jie, J. Li, X. Wang and G. Zhang, Influence of oxygen deficiency on the synthesis of tungsten oxide and the photocatalytic activity for the removal of organic dye, J. Alloys Compd., 2017, 690, 239–248 CrossRef CAS.
- B. Ahmed, S. Kumar, A. K. Ojha, P. Donfack and A. Materny, Facile and controlled synthesis of aligned WO3 nanorods and nanosheets as an efficient photocatalyst material, Spectrochim. Acta, Part A, 2017, 175, 250–261 CrossRef CAS PubMed.
- J. Z. Ou, R. A. Rani, S. Balendhran, A. S. Zoolfakar, M. R. Field, S. Zhuiykov, A. P. O'Mullane and K. Kalantar-zadeh, Anodic formation of a thick three-dimensional nanoporous WO3 film and its photocatalytic property, Electrochem. Commun., 2013, 27, 128–132 CrossRef CAS.
- X. Gao, X. Su, C. Yang, F. Xiao, J. Wang, X. Cao, S. Wang and L. Zhang, Hydrothermal synthesis of WO3 nanoplates as highly sensitive cyclohexene sensor and high-efficiency MB photocatalyst, Sens. Actuators, B, 2013, 181, 537–543 CrossRef CAS.
- Y. F. Rao, W. Chu and Y. R. Wang, Photocatalytic oxidation of carbamazepine in triclinic-WO3 suspension: Role of alcohol and sulfate radicals in the degradation pathway, Appl. Catal., A, 2013, 468, 240–249 CrossRef CAS.
- Y. Lu, G. Liu, J. Zhang, Z. Feng, Z. Li and C. Li, Fabrication of a monoclinic/hexagonal junction in WO3 and its enhanced photocatalytic degradation of rhodamine B, Chin. J. Catal., 2016, 37, 349–358 CrossRef CAS.
- Y. Liu, J. Li, H. Tang, W. Li, Y. Yang, Y. Li and Q. Chen, Enhanced photoelectrochemical performance of plate-like WO3 induced by surface oxygen vacancies, Electrochem. Commun., 2016, 68, 81–85 CrossRef CAS.
- J. Wang, Z. Chen, G. Zhai and Y. Men, Boosting photocatalytic activity of WO3 nanorods with tailored surface oxygen vacancies for selective alcohol oxidations, Appl. Surf. Sci., 2018, 462, 760–771 CrossRef CAS.
- Z. Lou, Q. Gu, L. Xu, Y. Liao and C. Xue, Surfactant-Free Synthesis of Plasmonic Tungsten Oxide Nanowires with Visible-Light-Enhanced Hydrogen Generation from Ammonia Borane, Chem. – Asian J., 2015, 10, 1291–1294 CrossRef CAS PubMed.
- J. Meng, Q. Lin, T. Chen, X. Wei, J. Li and Z. Zhang, Oxygen vacancy regulation on tungsten oxides with specific exposed facets for enhanced visible-light-driven photocatalytic oxidation, Nanoscale, 2018, 10, 2908–2915 RSC.
- B. Bhuyan, B. Paul, S. S. Dhar and S. Vadivel, Facile hydrothermal synthesis of ultrasmall W18O49 nanoparticles and studies of their photocatalytic activity towards degradation of methylene blue, Mater. Chem. Phys., 2017, 188, 1–7 CrossRef CAS.
- X. Zhao, S. Huang, Y. Liu, Q. Liu and Y. Zhang, In situ preparation of highly stable polyaniline/W18O49 hybrid nanocomposite as efficient visible light photocatalyst for aqueous Cr(VI) reduction, J. Hazard. Mater., 2018, 353, 466–475 CrossRef CAS PubMed.
- W. Wei, Y. Yao, Q. Zhao, Z. Xu, Q. Wang, Z. Zhang and Y. Gao, Oxygen defect-induced localized surface plasmon resonance at the WO3−x quantum dot/silver nano-wire interface: SERS and photocatalysis, Nanoscale, 2019, 11, 5535–5547 RSC.
- G. Xi, S. Ouyang, P. Li, J. Ye, Q. Ma, N. Su, H. Bai and C. Wang, Ultrathin W18O49 Nanowires with Diameters below 1 nm: Synthesis, Near-Infrared Absorption, Photoluminescence, and Photochemical Reduction of Carbon Dioxide, Angew. Chem., Int. Ed., 2012, 51, 2395–2399 CrossRef CAS PubMed.
- P. Chen, M. Qin, D. Zhang, Z. Chen, B. Jia, Q. Wan, H. Wu and X. Qu, Combustion synthesis and excellent photocatalytic degradation properties of W18O49, CrystEngComm, 2015, 17, 5889–5894 RSC.
- Y. P. Xie, G. Liu, L. Yin and H. Cheng, Crystal facet-dependent photocatalytic oxidation and reduction reactivity of monoclinic WO3 for solar energy conversion, J. Mater. Chem., 2012, 22, 6746–6751 RSC.
- D. Zhang, S. Wang, J. Zhu, H. Li and Y. Lu, WO3 nanocrystals with tunable percentage of (001)-facet exposure, Appl. Catal., B, 2012, 123–124, 398–404 CrossRef CAS.
- S. Wang, H. Chen, G. Gao, T. Butburee, M. Lyu, S. Thaweesak, J. Yun, A. Du, G. Liu and L. Wang, Synergistic crystal facet engineering and structural control of WO3 films exhibiting unprecedented photoelectrochemical performance, Nano Energy, 2016, 24, 94–102 CrossRef CAS.
- Y. Guo, X. Quan, N. Lu, H. Zhao and S. Chen, High Photocatalytic Capability of Self-Assembled Nanoporous WO3 with Preferential Orientation of (002) Planes, Environ. Sci. Technol., 2007, 41, 4422–4427 CrossRef CAS PubMed.
- Y. Li, Z. Tang, Z. Zhang and J. Zhang, Enhanced photocatalytic performance of tungsten oxide through tuning exposed facets and introducing oxygen vacancies, J. Alloys Compd., 2017, 708, 358–366 CrossRef CAS.
- D. Tanaka, Y. Oaki and H. Imai, Enhanced photocatalytic activity of quantum-confined tungsten trioxide nanoparticles in mesoporous silica, Chem. Commun., 2010, 46, 5286–5288 RSC.
- A. Fakhri and S. Behrouz, Photocatalytic properties of tungsten trioxide (WO3) nanoparticles for degradation of Lidocaine under visible and sunlight irradiation, Sol. Energy, 2015, 112, 163–168 CrossRef CAS.
- S. Shukla, S. Chaudhary, A. Umar, G. R. Chaudhary, S. K. Kansal and S. K. Mehta, Surfactant functionalized tungsten oxide nanoparticles with enhanced photocatalytic activity, Chem. Eng. J., 2016, 288, 423–431 CrossRef CAS.
- N. Zhang, C. Chen, Z. Mei, X. Liu, X. Qu, Y. Li, S. Li, W. Qi, Y. Zhang, J. Ye, V. A. L. Roy and R. Ma, Monoclinic Tungsten Oxide with {100} Facet Orientation and Tuned Electronic Band Structure for Enhanced Photocatalytic Oxidations, ACS Appl. Mater. Interfaces, 2016, 8, 10367–10374 CrossRef CAS PubMed.
- C. Sui, J. Gong, T. Cheng, G. Zhou and S. Dong, Fabrication of tungsten oxide microfibers with photocatalytic activity by electrospunning from PVA/H3PW12O40 gel, Appl. Surf. Sci., 2011, 257, 8600–8604 CrossRef CAS.
- F. A. Ofori, F. A. Sheikh, R. Appiah-Ntiamoah, X. Yang and H. Kim, A Simple Method of Electrospun Tungsten Trioxide Nanofibers with Enhanced Visible-Light Photocatalytic Activity, Nano-Micro Lett., 2015, 7, 291–297 CrossRef CAS PubMed.
- H. Zhang, J. Yang, D. Li, W. Guo, Q. Qin, L. Zhu and W. Zheng, Template-free facile preparation of monoclinic WO3 nanoplates and their high photocatalytic activities, Appl. Surf. Sci., 2014, 305, 274–280 CrossRef CAS.
- M. R. Waller, T. K. Townsend, J. Zhao, E. M. Sabio, R. L. Chamousis, N. D. Browning and F. E. Osterloh, Single-Crystal Tungsten Oxide Nanosheets: Photochemical Water Oxidation in the Quantum Confinement Regime, Chem. Mater., 2012, 24, 698–704 CrossRef CAS.
- K. Villa, S. Murcia-López, T. Andreu and J. R. Morante, Mesoporous WO3 photocatalyst for the partial oxidation of methane to methanol using electron scavengers, Appl. Catal., B, 2015, 163, 150–155 CrossRef CAS.
- A. B. D. Nandiyanto, O. Arutanti, T. Ogi, F. Iskandar, T. O. Kim and K. Okuyama, Synthesis of spherical macroporous WO3 particles and their high photocatalytic performance, Chem. Eng. Sci., 2013, 101, 523–532 CrossRef CAS.
- Z. Chen, J. Wang, G. Zhai, W. An and Y. Men, Hierarchical yolk-shell WO3 microspheres with highly enhanced photoactivity for selective alcohol oxidations, Appl. Catal., B, 2017, 218, 825–832 CrossRef CAS.
- J. Yu, L. Qi, B. Cheng and X. Zhao, Effect of calcination temperatures on microstructures and photocatalytic activity of tungsten trioxide hollow microspheres, J. Hazard. Mater., 2008, 160, 621–628 CrossRef CAS PubMed.
- J. Yu, H. Yu, H. Guo, M. Li and S. Mann, Spontaneous Formation of a Tungsten Trioxide Sphere-in-Shell Superstructure by Chemically Induced Self-Transformation, Small, 2008, 4, 87–91 CrossRef CAS PubMed.
- G. Xi, Y. Yan, Q. Ma, J. Li, H. Yang, X. Lu and C. Wang, Synthesis of Multiple-Shell WO3 Hollow Spheres by a Binary Carbonaceous Template Route and Their Applications in Visible-Light Photocatalysis, Chem. – Eur. J., 2012, 18, 13949–13953 CrossRef CAS PubMed.
- S. Yao, X. Zhang, F. Qu, A. Umar and X. Wu, Hierarchical WO3 nanostructures assembled by nanosheets and their applications in wastewater purification, J. Alloys Compd., 2016, 689, 570–574 CrossRef CAS.
- S. K. Biswas and J. Baeg, A facile one-step synthesis of single crystalline hierarchical WO3 with enhanced activity for photoelectrochemical solar water oxidation, Int. J. Hydrogen Energy, 2013, 38, 3177–3188 CrossRef CAS.
- J. Yu and L. Qi, Template-free fabrication of hierarchically flower-like tungsten trioxide assemblies with enhanced visible-light-driven photocatalytic activity, J. Hazard. Mater., 2009, 169, 221–227 CrossRef CAS PubMed.
- Q. Li, L. Wang, D. Chu, X. Yang and Z. Zhang, Cylindrical stacks and flower-like tungsten oxide microstructures: Controllable synthesis and photocatalytic properties, Ceram. Int., 2014, 40, 4969–4973 CrossRef CAS.
- R. M. Fernández-Domene, R. Sánchez-Tovar, B. Lucas-Granados, C. S. García-Zamora and J. García-Antón, Customized WO3 nanoplatelets as visible-light photoelectrocatalyst for the degradation of a recalcitrant model organic compound (methyl orange), J. Photochem. Photobiol., A, 2018, 356, 46–56 CrossRef.
- M. Sadakane, K. Sasaki, H. Kunioku, B. Ohtani, R. Abe and W. Ueda, Preparation of 3-D ordered macroporous tungsten oxides and nano-crystalline particulate tungsten oxides using a colloidal crystal template method, and their structural characterization and application as photocatalysts under visible light irradiation, J. Mater. Chem., 2010, 20, 1811–1818 RSC.
- N. Zhang, A. Jalil, D. Wu, S. Chen, Y. Liu, C. Gao, W. Ye, Z. Qi, H. Ju, C. Wang, X. Wu, L. Song, J. Zhu and Y. Xiong, Refining Defect States in W18O49 by Mo Doping: A Strategy for Tuning N2 Activation towards Solar-Driven Nitrogen Fixation, J. Am. Chem. Soc., 2018, 140, 9434–9443 CrossRef CAS PubMed.
- S. S. Kalanur and H. Seo, Influence of molybdenum doping on the structural, optical and electronic properties of WO3 for improved solar water splitting, J. Colloid Interface Sci., 2018, 509, 440–447 CrossRef CAS PubMed.
- S. S. Kalanur and H. Seo, Aligned nanotriangles of tantalum doped tungsten oxide for improved photoelectrochemical
water splitting, J. Alloys Compd., 2019, 785, 1097–1105 CrossRef CAS.
- W. Mu, X. Xie, X. Li, R. Zhang, Q. Yu, K. Lv, H. Wei and Y. Jian, Characterizations of Nb-doped WO3 nanomaterials and their enhanced photocatalytic performance, RSC Adv., 2014, 4, 36064–36070 RSC.
- S. S. Kalanur, I. Yoo and H. Seo, Fundamental investigation of Ti doped WO3 photoanode and their influence on photoelectrochemical water splitting activity, Electrochim. Acta, 2017, 254, 348–357 CrossRef CAS.
- F. Mehmood, J. Iqbal, T. Jan, W. Ahmed, W. Ahmed, A. Arshad, Q. Mansoor, S. Z. Ilyas, M. Ismail and I. Ahmad, Effect of Sn doping on the structural, optical, electrical and anticancer properties of WO3 nanoplates, Ceram. Int., 2016, 42, 14334–14341 CrossRef CAS.
- S. S. Kalanur, I. Yoo, K. Eom and H. Seo, Enhancement of photoelectrochemical water splitting response of WO3 by Means of Bi doping, J. Catal., 2018, 357, 127–137 CrossRef.
- F. Mehmood, J. Iqbal, T. Jan and Q. Mansoor, Structural, Raman and photoluminescence properties of Fe doped WO3 nanoplates with anti cancer and visible light driven photocatalytic activities, J. Alloys Compd., 2017, 728, 1329–1337 CrossRef CAS.
- H. Song, Y. Li, Z. Lou, M. Xiao, L. Hu, Z. Ye and L. Zhu, Synthesis of Fe-doped WO3 nanostructures with high visible-light-driven photocatalytic activities, Appl. Catal., B, 2015, 166–167, 112–120 CrossRef CAS.
- S. L. Liew, Z. Zhang, T. W. G. Goh, G. S. Subramanian, H. L. D. Seng, T. S. A. Hor, H. K. Luo and D. Z. Chi, Yb-doped WO3 photocatalysts for water oxidation with visible light, Int. J. Hydrogen Energy, 2014, 39, 4291–4298 CrossRef CAS.
- L. Xu, D. Gu, X. Chang, L. Chai, Z. Li, X. Jin and S. Sun, Rare-earth-doped tungsten oxide microspheres with highly enhanced photocatalytic activites, Ceram. Int., 2017, 43, 10263–10269 CrossRef CAS.
- F. Mehmood, J. Iqbal, T. Jan, A. Gul, Q. Mansoor and R. Faryal, Structural, photoluminescence, electrical, anti cancer and visible light driven photocatalytic characteristics of Co doped WO3 nanoplates, Vib. Spectrosc., 2017, 93, 78–89 CrossRef CAS.
- F. Mehmood, J. Iqbal, A. Gul, W. Ahmed and M. Ismail, Facile synthesis of 2-D Cu doped WO3 nanoplates with structural, optical and differential anti cancer characteristics, Physica E, 2017, 88, 188–193 CrossRef CAS.
- X. F. Cheng, W. H. Leng, D. P. Liu, J. Q. Zhang and C. N. Cao, Enhanced photoelectrocatalytic performance of Zn-doped WO3 photocatalysts for nitrite ions degradation under visible light, Chemosphere, 2007, 68, 1976–1984 CrossRef CAS PubMed.
- F. Mehmood, J. Iqbal, M. Ismail and A. Mehmood, Ni doped WO3 nanoplates: An excellent photocatalyst and novel nanomaterial for enhanced anticancer activities, J. Alloys Compd., 2018, 746, 729–738 CrossRef CAS.
- H. Wang, L. Zhang, K. Wang, X. Sun and W. Wang, Enhanced photocatalytic CO2 reduction to methane over WO3·0.33H2O via Mo doping, Appl. Catal., B, 2019, 243, 771–779 CrossRef CAS.
- J. Kim, C. W. Lee and W. Choi, Platinized WO3 as an Environmental Photocatalyst that Generates OH Radicals under Visible Light, Environ. Sci. Technol., 2010, 44, 6849–6854 CrossRef CAS PubMed.
- H. W. Choi, E. J. Kim and S. H. Hahn, Photocatalytic activity of Au-buffered WO3 thin films prepared by RF magnetron sputtering, Chem. Eng. J., 2010, 161, 285–288 CrossRef CAS.
- J. Chen, Y. Ren, T. Hu, T. Xu and Q. Xu, Fabrication and application of substoichiometric tungsten oxide with tunable localized surface plasmon resonances, Appl. Surf. Sci., 2019, 465, 517–525 CrossRef CAS.
- S. Sun, W. Wang, S. Zeng, M. Shang and L. Zhang, Preparation of ordered mesoporous Ag/WO3 and its highly efficient degradation of acetaldehyde under visible-light irradiation, J. Hazard. Mater., 2010, 178, 427–433 CrossRef CAS PubMed.
- K. A. Willets and R. P. Van Duyne, Localized surface plasmon resonance spectroscopy and sensing, Annu. Rev. Phys. Chem., 2007, 58, 267–297 CrossRef CAS PubMed.
- W. Hou and S. B. Cronin, A Review of Surface Plasmon Resonance-Enhanced Photocatalysis, Adv. Funct. Mater., 2013, 23, 1612–1619 CrossRef CAS.
- Y. Tian and T. Tatsuma, Plasmon-induced photoelectrochemistry at metal nanoparticles supported on nanoporous TiO2, Chem. Commun., 2004, 1810–1811 RSC.
- J. Ding, L. Zhang, Q. Liu, W. Dai and G. Guan, Synergistic effects of electronic structure of WO3 nanorods with the dominant {001} exposed facets combined with silver size-dependent on the visible-light photocatalytic activity, Appl. Catal., B, 2017, 203, 335–342 CrossRef CAS.
- F. Le, D. W. Brandl, Y. A. Urzhumov, H. Wang, J. Kundu, N. J. Halas, J. Aizpurua and P. Nordlander, Metallic Nanoparticle Arrays: A Common Substrate for Both Surface-Enhanced Raman Scattering and Surface-Enhanced Infrared Absorption, ACS Nano, 2008, 2, 707–718 CrossRef CAS PubMed.
- W. Zhu, J. Liu, S. Yu, Y. Zhou and X. Yan, Ag loaded WO3 nanoplates for efficient photocatalytic degradation of sulfanilamide and their bactericidal effect under visible light irradiation, J. Hazard. Mater., 2016, 318, 407–416 CrossRef CAS PubMed.
- N. Lu, Z. Zhang, Y. Wang, B. Liu, L. Guo, L. Wang, J. Huang, K. Liu and B. Dong, Direct evidence of IR-driven hot electron transfer in metal-free plasmonic W18O49/Carbon heterostructures for enhanced catalytic H2 production, Appl. Catal., B, 2018, 233, 19–25 CrossRef CAS.
- T. A. Saleh and V. K. Gupta, Functionalization of tungsten oxide into MWCNT and its application for sunlight-induced degradation of rhodamine B, J. Colloid Interface Sci., 2011, 362, 337–344 CrossRef CAS PubMed.
- W. Zhu, F. Sun, R. Goei and Y. Zhou, Facile fabrication of RGO-WO3 composites for effective visible light photocatalytic degradation of sulfamethoxazole, Appl. Catal., B, 2017, 207, 93–102 CrossRef CAS.
- S. Prabhu, L. Cindrella, O. J. Kwon and K. Mohanraju, Green synthesis of rGO-WO3 composite and its efficient photoelectrochemical water splitting, Int. J. Hydrogen Energy, 2017, 42, 29791–29796 CrossRef CAS.
- L. Fu, T. Xia, Y. Zheng, J. Yang, A. Wang and Z. Wang, Preparation of WO3-reduced graphene oxide nanocomposites with enhanced photocatalytic property, Ceram. Int., 2015, 41, 5903–5908 CrossRef CAS.
- X. Li, S. Yang, J. Sun, P. He, X. Xu and G. Ding, Tungsten oxide nanowire-reduced graphene oxide aerogel for high-efficiency visible light photocatalysis, Carbon, 2014, 78, 38–48 CrossRef CAS.
- H. Khan, M. G. Rigamonti, G. S. Patience and D. C. Boffito, Spray dried TiO2/WO3 heterostructure for photocatalytic applications with residual activity in the dark, Appl. Catal., B, 2018, 226, 311–323 CrossRef CAS.
- M. A. Lara, C. Jaramillo-Páez, J. A. Navío, P. Sánchez-Cid and M. C. Hidalgo, Coupling of WO3 with anatase TiO2 sample with high {001} facet exposition: Effect on the photocatalytic properties, Catal. Today, 2019, 328, 142–148 CrossRef CAS.
- L. Pan, J. Zhang, X. Jia, Y. Ma, X. Zhang, L. Wang and J. Zou, Highly efficient Z-scheme WO3−x quantum dots/TiO2 for photocatalytic hydrogen generation, Chin. J. Catal., 2017, 38, 253–259 CrossRef CAS.
- A. Memar, C. M. Phan and M. O. Tade, Photocatalytic activity of WO3/Fe2O3 nanocomposite photoanode, Int. J. Hydrogen Energy, 2015, 40, 8642–8649 CrossRef CAS.
- Y. Li, J. Feng, H. Li, X. Wei, R. Wang and A. Zhou, Photoelectrochemical splitting of natural seawater with α-Fe2O3/WO3 nanorod arrays, Int. J. Hydrogen Energy, 2016, 41, 4096–4105 CrossRef CAS.
- J. Zhang, H. Ma and Z. Liu, Highly efficient photocatalyst based on all oxides WO3/Cu2O heterojunction for photoelectrochemical water splitting, Appl. Catal., B, 2017, 201, 84–91 CrossRef CAS.
- A. K. L. Sajjad, S. Sajjad, A. Iqbal and N. Ryma, ZnO/WO3 nanostructure as an efficient visible light catalyst, Ceram. Int., 2018, 44, 9364–9371 CrossRef CAS.
- Z. Lou, M. Zhu, X. Yang, Y. Zhang, M. Whangbo, B. Li and B. Huang, Continual injection of photoinduced electrons stabilizing surface plasmon resonance of non-elemental-metal plasmonic photocatalyst CdS/WO3−x for efficient hydrogen generation, Appl. Catal., B, 2018, 226, 10–15 CrossRef CAS.
- L. Zhang, H. Zhang, B. Wang, X. Huang, Y. Ye, R. Lei, W. Feng and P. Liu, A facile method for regulating the charge transfer route of WO3/CdS in high-efficiency hydrogen production, Appl. Catal., B, 2019, 244, 529–535 CrossRef CAS.
- C. Liu, Y. Yang, W. Li, J. Li, Y. Li and Q. Chen, Construction of novel Bi2S3 nanobelt @ WO3 nanoplate arrays on FTO glass with high photoelectrochemical activity, Int. J. Hydrogen Energy, 2016, 41, 5878–5886 CrossRef CAS.
- J. Rong, T. Zhang, F. Qiu, X. Rong, X. Zhu and X. Zhang, Preparation of hierarchical micro/nanostructured Bi2S3-WO3 composites for enhanced photocatalytic performance, J. Alloys Compd., 2016, 685, 812–819 CrossRef CAS.
- L. Ye and Z. Wen, ZnIn2S4 nanosheets decorating WO3 nanorods core-shell hybrids for boosting visible-light photocatalysis hydrogen generation, Int. J. Hydrogen Energy, 2019, 44, 3751–3759 CrossRef CAS.
- T. Wang, W. Quan, D. Jiang, L. Chen, D. Li, S. Meng and M. Chen, Synthesis of redox-mediator-free direct Z-scheme AgI/WO3 nanocomposite photocatalysts for the degradation of tetracycline with enhanced photocatalytic activity, Chem. Eng. J., 2016, 300, 280–290 CrossRef CAS.
- Y. L. Qi, Y. F. Zheng and X. C. Song, Synthesis and enhanced visible light photocatalytic activity of WO3-BiOClxBr1−x heterojunctions with tunable energy band structure, Ceram. Int., 2017, 43, 12302–12310 CrossRef CAS.
- E. Grilla, A. Petala, Z. Frontistis, I. K. Konstantinou, D. I. Kondarides and D. Mantzavinos, Solar photocatalytic abatement of sulfamethoxazole over Ag3PO4/WO3 composites, Appl. Catal., B, 2018, 231, 73–81 CrossRef CAS.
- S. Xu, D. Fu, K. Song, L. Wang, Z. Yang, W. Yang and H. Hou, One-dimensional WO3/BiVO4 heterojunction photoanodes for efficient photoelectrochemical water splitting, Chem. Eng. J., 2018, 349, 368–375 CrossRef CAS.
- Y. Liu, B. R. Wygant, K. Kawashima, O. Mabayoje, T. E. Hong, S. Lee, J. Lin, J. Kim, K. Yubuta, W. Li, J. Li and C. B. Mullins, Facet effect on the photoelectrochemical performance of a WO3/BiVO4 heterojunction photoanode, Appl. Catal., B, 2019, 245, 227–239 CrossRef CAS.
- J. Zhu, W. Li, J. Li, Y. Li, H. Hu and Y. Yang, Photoelectrochemical activity of NiWO4/WO3 heterojunction photoanode under visible light irradiation, Electrochim. Acta, 2013, 112, 191–198 CrossRef CAS.
- M. Gui, W. Zhang, Y. Chang and Y. Yu, One-step hydrothermal preparation strategy for nanostructured WO3/Bi2WO6 heterojunction with high visible light photocatalytic activity, Chem. Eng. J., 2012, 197, 283–288 CrossRef CAS.
- Y. Peng, Q. Chen, D. Wang, H. Zhou and A. Xu, Synthesis of one-dimensional WO3-Bi2WO6 heterojunctions with enhanced photocatalytic activity, CrystEngComm, 2015, 17, 569–576 RSC.
- Y. Xiao, X. Tao, G. Qiu, Z. Dai, P. Gao and B. Li, Optimal synthesis of a direct Z-scheme photocatalyst with ultrathin W18O49 nanowires on g-C3N4 nanosheets for solar-driven oxidation reactions, J. Colloid Interface Sci., 2019, 550, 99–109 CrossRef CAS PubMed.
- J. Chen, X. Xiao, Y. Wang and Z. Ye, Fabrication of hierarchical sheet-on-sheet WO3/g-C3N4 composites with enhanced photocatalytic activity, J. Alloys Compd., 2019, 777, 325–334 CrossRef CAS.
- Z. Huang, J. Song, X. Wang, L. Pan, K. Li, X. Zhang, L. Wang and J. Zou, Switching charge transfer of C3N4/W18O49 from type-II to Z-scheme by interfacial band bending for highly efficient photocatalytic hydrogen evolution, Nano Energy, 2017, 40, 308–316 CrossRef CAS.
- H. Xu, H. Zhao, Y. Xu, Z. Chen, L. Huang, Y. Li, Y. Song, Q. Zhang and H. Li, Three-dimensionally ordered macroporous WO3 modified Ag3PO4 with enhanced visible light photocatalytic performance, Ceram. Int., 2016, 42, 1392–1398 CrossRef CAS.
- H. Zhou, Z. Wen, J. Liu, J. Ke, X. Duan and S. Wang, Z-scheme plasmonic Ag decorated WO3/Bi2WO6 hybrids for enhanced photocatalytic abatement of chlorinated-VOCs under solar light irradiation, Appl. Catal., B, 2019, 242, 76–84 CrossRef CAS.
|
This journal is © the Partner Organisations 2020 |
Click here to see how this site uses Cookies. View our privacy policy here.