Effect of the irrigation water type and other environmental parameters on CeO2 nanopesticide–clay colloid interactions†
Received
23rd September 2019
, Accepted 21st November 2019
First published on 21st November 2019
Abstract
In this work, the stability and aggregation behaviour of CeO2 nanoparticles (NPs) was investigated to predict their fate in the agricultural environment. For this, the aggregation kinetics of CeO2 NPs was studied under varying pH, ionic strength (IS), dissolved organic matter (DOM) and carbonate concentrations in the presence of clay. Furthermore, different types of irrigation water have been used to check the fate of CeO2 nanoparticles (NPs) in complex aqueous matrices. The results show that critical coagulation concentration (CCC) values obtained for CeO2 NPs, i.e. 26.5 mM and 7.9 mM for NaCl and CaCl2 respectively, drastically decreased to 16.2 mM and 1.87 mM in the presence of bentonite clay colloids, which may lead to their deposition within the soil matrix. However, the presence of bicarbonate ions (0.1–2 mM) along with DOM (1–20 mg L−1) may result in their stabilization and co-transport of CeO2 NPs with clay in water bodies having low ionic strength. It was also observed that the negative charge of a bentonite clay suspension was completely reversed with an increase in CeO2 concentration by 37.5 times. The critical charge reversal concentration value was 284.4 mg L−1 in Milli-Q water whereas values were observed to be 680 mg L−1 in synthetic-soft water, followed by natural river water (867 mg L−1) and synthetic-hard water (910 mg L−1). The synergistic effect of temperature and ionic strength was observed on the aggregation behaviour of CeO2 NPs in environmental water samples of varying composition.
Environmental significance
Extensive utilization of metal oxide nanoparticles (MONPs) in the agricultural sector is a serious concern and CeO2 nanoparticles are one of them. The stability studies of MONPs are important to predict the behaviour of MONPs in the agricultural environment. The stabilization of these nanoparticles in the environment enhances their transportation and bioavailability, while their aggregation leads to deposition in sediments. The stability of MONPs is governed by various environmental factors such as ionic strength, pH, organic matter, and natural colloids studied in this study. The results show that the ionic strength and clay colloids are the major driving factors which control the aggregation behaviour of CeO2 NPs in the soil environment.
|
1. Introduction
Nanotechnology applications in the field of agriculture have emerged in the last decade due to increased demand for crop production for human consumption as well as for animal feeding.1 There are many applications of these emerging technologies in the agricultural sector that have been reported so far.2 Hence, the use of nanotechnology in different forms such as nano-agrochemicals, nano-sensors, and nano-amendments in agriculture enables a direct route of exposure for nanoparticles (NPs) to the soil system. Various Metal Oxide Nanoparticles (MONPs) such as CuO, Ag, ZnO, and TiO2 NPs that possess fungicidal and bacterial properties are used as fungicidal agents.3–5 Use of Fe, Mn, Zn, and Mo as nano-fertilizers has also been reported.6,7 Furthermore, nano-CeO2 has also been explored for various agricultural applications. For example, Wu et al. investigated the capability of CeO2 to improve salinity tolerance in Arabidopsis by reducing ROS and –OH radicals that lack enzymatic scavenging pathways.8 The application of nano-ceria also showed a suppression of fusarium wilt and improved the chlorophyll content in tomato plants.9 The effect of CeO2 NPs on yield and growth of wheat has been studied, and the results showed that it had increased the plant growth, shoot biomass and grain yield by 9.0%, 12.7%, and 36.6%, respectively.10 An increase in growth and an enhanced photosynthesis rate of soybeans (Glycine max (L.) Merr.) by 54% have also been reported upon the application of bare nano-ceria at a concentration of 100 mg kg−1.11 Gui et al. also reported the enhanced growth of lettuce (Lactuca sativa) in soil treated with 100 mg kg−1 of CeO2 NPs.12 The CeO2 NPs were used as both a pesticide and growth promoter; however, the mechanism of action is not clear yet. Therefore, in our study, we have considered CeO2 NPs as a nano-pesticide due to their antifungal properties. Apart from the direct use of CeO2 NPs in the agriculture sector, anthropogenic sources such as their use in painting, biomedicine and as a catalyst also contribute towards the addition of CeO2 NPs in an agricultural soil environment.13,14
This expansion of the use of CeO2 NPs raises a concern about their behaviour and toxicity in the environment. Many phytotoxicity studies are going on to study the uptake and translocation of these NPs in the food chain,12,15–19 but still the knowledge of fate, transport, potential environmental risk, and bioavailability of the MONPs are not enough to understand their fate in the soil environment. Many previous studies have examined the fate of different MONPs under different conditions and explained the role of various parameters that govern the behaviour of NPs in the environment. For example, Fang et al. observed less retention of TiO2 NPs in soils having low ionic strength and larger grain size, while high retention was observed in soils with a higher clay content and salinity.20 Baalousha et al. studied the effect of pH and natural organic matter (NOM) on the aggregation of iron oxide nanoparticles and observed that a high concentration of natural organic matter caused the disaggregation of iron oxide nanoparticles.21 The disaggregation was caused due to the sorption of NOM on the surface of nanoparticles and increased surface charge. Keller et al. also observed that the electrophoretic mobility of MONPs in aqueous media highly depends on the ionic strength and presence of NOM.22 Quik et al. also showed that natural colloids play an important role in the aggregation of nanoparticles and their sedimentation. Apart from natural colloids, various water parameters like pH, ionic strength and the presence of organic matter also control the aggregation behaviour of nanoparticles in an aquatic environment.23 Fang et al. also studied the effect of similarly charged co-existing nanoparticles on the aggregation and stability of engineered nanoparticles in an aqueous environment and found that their co-existence enhanced the stability of both the NPs in the system, which increases their risk of exposure to the environment.
The knowledge from previous studies suggests that the environmental behaviour of metal-based nano-pesticides plays a very crucial role in determining their fate, mobility, toxicity and bioavailability in the environment. The behaviour of these NPs in the soil environment is governed by their unique properties as well as the properties of soil (i.e., soil texture, organic matter, pH, the roughness of soil grains, etc.) and soil pore water chemistry where they are being applied.24–27 The clay fraction and organic matter of soil control most of its properties, i.e., water retention, ion exchange, metal adsorption–desorption, etc.28,29. Apart from this, clay colloids dispersed in soil pore water may also affect the aggregation behaviour of NPs in soil via hetero-aggregation, similar to natural colloids present in the aqueous environment.
However, these studies have examined the behaviour of NPs either under controlled conditions in the presence of individual parameters mentioned above or with most complex systems, i.e. natural environmental samples. Moreover, the behaviour of CeO2 NPs, in particular, is least studied. Therefore, in the present work, we have evaluated the sequential effect of various environmental parameters such as ionic strength, bicarbonate ions, dissolved organic matter (DOM), and inorganic clay colloids in combination, on the aggregation behaviour of CeO2 NPs under controlled environment. This study has also examined the aggregation kinetics of CeO2 NPs in different environmental water samples and data were interpreted based on experiments done in the controlled environment. The effect of temperature on the aggregation behaviour of NPs along with other environmental factors has also been considered, which was not addressed in previous studies. The effect of bicarbonate ions individually and in combination with organic matter and bentonite clay colloids has also been studied. Note that bicarbonate ions play an important role in controlling the speciation and pH of the ionic species and aquatic system respectively, which was also not addressed earlier.
Clay colloids, commonly present in natural aqueous systems, are negatively charged and have the property of adsorption of positively charged ions (toxic metals) and other reactive particles like metal oxides, etc.30 which may change or at enhanced metal accumulation (overloading of toxic metals) even reverse the overall surface charge in the colloidal system. To address such a situation, Critical Charge Reversal Concentration (CCRC) (i.e., concentration of MONPs required to reverse the surface charge of clay particles) was obtained. For that, change in the zeta potential of colloids as a function of CeO2 concentration was studied. On the other hand, the type of water, used in irrigation, can also dramatically change the fate of these nanoparticles; therefore, the aggregation behaviour and charge properties were studied in various irrigation water types, i.e., natural river water (NW), synthetic freshwater (SW), and synthetic hard water (HW).
Overall, this study reveals the importance of environmental parameters to understand the complete fate of CeO2 nanoparticles in complex systems.
2. Materials and methods
2.1 Materials
A CeO2 NP dispersion (10 wt%) and humic acid (HA) were purchased from Sigma-Aldrich. Sodium chloride, calcium chloride, hydrochloric acid (36%), sodium hydroxide and sodium bicarbonate were procured from Merck. Extra pure bentonite clay was obtained from Loba Chemie Pvt. Ltd.
SW and HW were prepared according to the protocol given by Smith et al.31 and physio–chemical parameters are provided in Section S1 of the ESI.† NW was collected from the Hooghly River, West Bengal, India and in situ water quality parameters such as pH, total dissolved solids, dissolved oxygen and conductivity were measured immediately (Section S2 of the ESI†). Water samples were filtered by using a 0.22-micron nylon filter membrane at the sampling location and stored at 4 °C before utilization.
2.2 CeO2 and bentonite NP suspension preparation
The amount of nano-CeO2 necessary to prepare a stock concentration of 1000 mg L−1 was sonicated in 100 mL of Milli-Q water at 25 °C for 30 minutes in a water bath.
To study the fate of CeO2 nanoparticles in the presence of environmentally relevant inorganic clay colloids, bentonite clay was used. To prepare the bentonite clay colloidal suspension, purchased clay powder was purified from soluble and coarse minerals to have better control of the ionic strength in the dispersions. For purification purpose, 5 g of clay was dispersed in 1 L Milli-Q water and stirred overnight to get a homogeneous solution. The solution was kept for 72 hours in the dark to settle; after that the sedimented fraction was rejected and the supernatant was centrifuged (Thermo Scientific Sorvall ST16R) for 30 minutes at 4800 rpm to get a homogenous nano-sized fraction of clay. Gravimetric analysis of the resultant supernatant was performed to obtain the concentration of the clay colloid suspension. The concentration of colloidal particles was estimated to be 800 mg L−1 and used as a stock for further experiments.
2.3 CeO2 and bentonite NP suspension characterization
The hydrodynamic diameter and zeta potential of NPs in solution were measured by using a Malvern Zetasizer (Nano ZS90). Field emission gun transmission electron microscopy (FEG-TEM) using a JEOL (JEM 2100F model) with a 200 kV electron source was used to confirm the primary size and morphology of CeO2 nanoparticles. CeO2 NPs were sonicated for 30 minutes and drop casted on a carbon-coated 300 mesh copper grid to obtain TEM images. Field emission scanning electron microscope (Carl Zeiss SUPRA 55VP FESEM) monographs were obtained by drop-casting the bentonite clay suspension on a silicon wafer, having a concentration of 80 mg L−1 dispersed in Milli-Q water.
2.4 Preparation of background solutions
The required amount of NaCl, CaCl2, NaHCO3, and HA was added to Milli-Q water to prepare a stock concentration of 500 mM, 200 mM, 10 mM and 36 mg L−1 respectively and the pH of the solutions was adjusted to 6 by using 0.1 M NaOH and 0.1 M HCl. In the case of HA, the pH of the solution was first adjusted to 11 for the complete dissolution of HA and then the suspension was filtered with a 0.45-micron cellulose acetate filter membrane, and the solution was brought back to pH 6. A TOC analyzer was used to measure the concentration of organic matter (OM) in HA stock solution.
2.5 Experimental design
The Dynamic Light Scattering (DLS) technique was used to study the interaction of CeO2 NPs with bentonite clay colloids under varying environmental parameters. The aggregation kinetics of CeO2 in the presence and absence of bentonite clay colloids was studied by estimating the average hydrodynamic diameter as a function of time by using a Malvern Zetasizer (Nano ZS90). A disposable cuvette was used to take measurements. To study the effect of monovalent and divalent ions, different concentrations of NaCl and CaCl2 were added to a solution containing CeO2, mixed and placed immediately in the Zetasizer to start the measurements. The hydrodynamic diameter was measured at a fixed angle of 90° every 10 seconds continuously for 5 minutes. Similar measurements were taken in the presence of bentonite clay colloids.
Bicarbonates and organic matter are other important environmental factors that influence the fate of nanoparticles. Therefore the effect of environment relevant concentrations of bicarbonates and DOM that range from 0.1–5 mM and 1–10 mg L−1 respectively in natural water systems32,33 was also studied. NaHCO3 and HA were used to represent bicarbonates and DOM respectively and their effect on the aggregation of CeO2 in the presence and absence of bentonite was studied similarly to that mentioned above.
The aggregation kinetics of CeO2 in the presence of bentonite clay colloids in natural aqueous media i.e., NW, SW, and HW was also studied under varying temperature. Analysis of variance (ANOVA) was performed to understand the significant difference in the aggregation behaviour of CeO2 NPs under varying temperature and in different water samples.
CCRC has also been obtained in different aqueous solutions, i.e., Milli-Q, NW, SW, and HW. For that, the pHpzc (pH at which the zeta potential at the surface of a particle equals to zero) of bentonite clay colloids under the varying concentration of CeO2 NPs was estimated by using a Zetasizer along with an accessory, MPT-2 multi-purpose pH titrator (Malvern).
2.6 QA/QC
To confirm the QA and QC of the experiments, the aggregation kinetics of CeO2 in the presence of bentonite clay colloids without any salt was also studied similarly to that mentioned above in the experimental design. The effect of various environmental parameters (ionic strength, carbonates, and organic matter) on CeO2 and bentonite clay colloids individually was also studied. All the experiments were performed in triplicate. Until specified, the concentration of CeO2, clay colloids, pH, and temperature were kept constant throughout the experiment, i.e. 10 mg L−1, 80 mg L−1, 6, and 25 °C respectively.
3. Results and discussion
3.1 Characterization of CeO2 NPs and bentonite clay colloids
The hydrodynamic diameters of CeO2 and bentonite clay colloids were 110 ± 5 nm and 135 ± 8 nm respectively. The pHpzc curve shows that CeO2 has a point of zero charge at pH 8 and it is positively charged below the pHpzc value (Fig. 1), whereas bentonite clay colloids do not have any pHpzc value for the whole studied pH range (i.e., 3 to 10) and were permanently negatively charged which is the case with naturally present colloids in aqueous systems.34
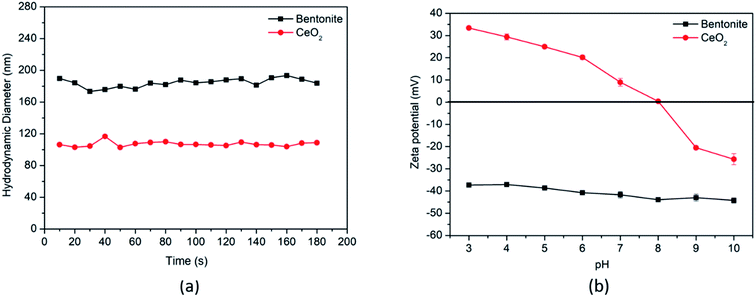 |
| Fig. 1 (a) Hydrodynamic diameter of CeO2 NPs and bentonite clay colloids as a function of time and (b) zeta potential as a function of pH. | |
The size and morphology of the CeO2 NPs were also analyzed using transmission electron microscopy (Fig. 2a). The micrograph shows the crystalline nature of the particles with a cubic shape. The particle size was observed to be <50 nm, which was smaller than the value measured by DLS (i.e. 110 ± 5 nm). This size difference can be explained by the fact that the DLS technique measures the hydrodynamic diameter of the particle or bi-particle homo-aggregates in the suspension, while TEM measures the projected size.
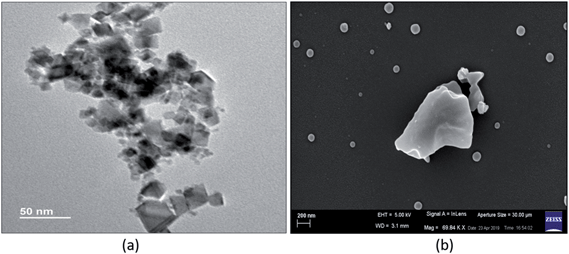 |
| Fig. 2 (a) TEM image of CeO2 NPs and (b) SEM image of bentonite clay colloids. | |
3.2 Effect of CeO2 concentration on the suspension stability and charge reversal of clay colloids
To obtain the CCRC value in a clay–CeO2 suspension system, CeO2 concentration was varied by keeping clay concentration constant i.e. 8 mg L−1 and pH titration of the clay–CeO2 suspension was performed for pHpzc. The results show that an increment in the CeO2 concentration resulted in increasing zeta potential of the clay–CeO2 suspension. The pHpzc of the suspension was found at pH = 4.4 in a 1
:
25 clay
:
CeO2 ratio (Fig. 3a). pHpzc further shifted toward the alkaline side as we increased the CeO2 concentration in the suspension. This is mainly due to the positive surface charge of CeO2 NPs (pHpzc = 8), which tries to balance the negative charge of clay surfaces and the oversaturation of clay colloids by CeO2 resulted in positive surface charge of the colloidal suspension.
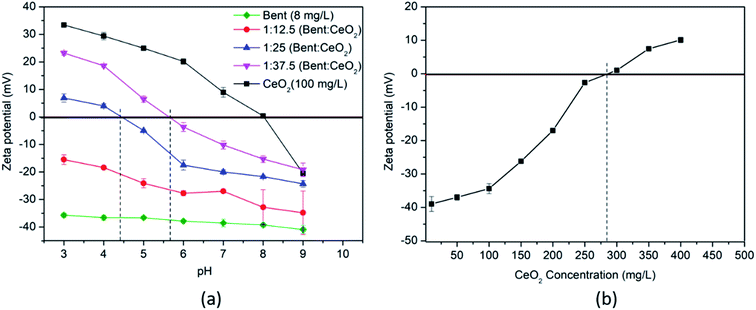 |
| Fig. 3 Zeta potential of the bentonite clay suspension (8 mg L−1) as a function of (a) CeO2 concentration and pH, and (b) CeO2 concentration. | |
To confirm the CCRC values obtained by varying the CeO2 concentration and pH, only the CeO2 concentration was varied (0–400 mg L−1) by keeping the clay concentration (8 mg L−1) and pH = 6 constant. The required concentration of CeO2 to have the charge reversal on the clay surface at 8 mg L−1 in Milli-Q water was found to be 284.4 mg L−1 at pH 6 (Fig. 3b) which nearly corresponds to a clay
:
CeO2 ratio of 1
:
37.5 at pH 5.8 (Fig. 3a). Therefore, to mimic near-natural conditions, 10-times higher concentration, i.e. 80 mg L−1, of clay colloids and nearly 25 times lower concentration, i.e. 10 mg L−1, of CeO2 NPs were used for further stability experiments.
3.3 Effect of ionic strength on the stability of CeO2 NPs in the absence and presence of bentonite clay colloids
The rate of aggregation for CeO2 NPs in the absence and presence of clay colloids was studied over a range of 5–30 mM NaCl and 1–10 mM CaCl2 at pH 6 where the zeta potential of CeO2 NPs and clay was +20 ± 2 mV and −38 ± 4 mV respectively (Fig. 1b). CCC values obtained for CeO2 NPs in the absence of clay colloids were found to be 26.5 mM and 7.9 mM for NaCl and CaCl2, respectively, showing higher aggregation in the system with increasing ionic strength and valency of ions. The observed results can be explained on the basis of the effect of the concentration and valency of ionic species on the inverse Debye-length (k−1) or double layer thickness and the effect of Debye length on electrostatic repulsion.35 The magnitude of k−1 is proportional to the concentration and the valence charge of the ions present in the solution.36,37 The Debye length decreases as the concentration and valence charge of ions increase (i.e. z = 1 for Na+ and z = 2 for Ca2+) which finally results in the reduction of electrostatic repulsion potential and leads to aggregation of the particles in the system as observed (Fig. 4a).
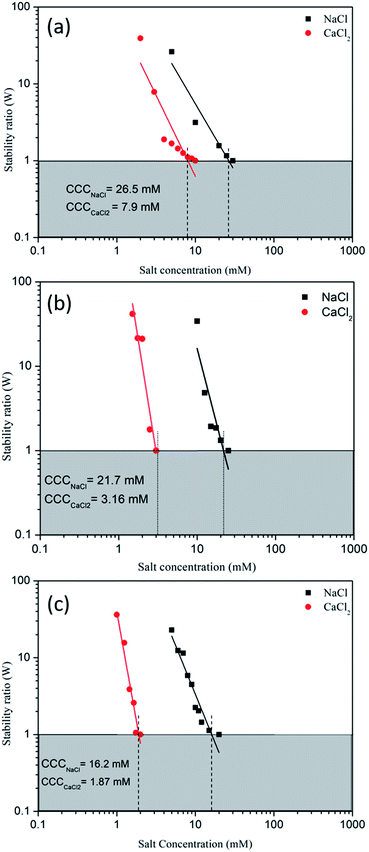 |
| Fig. 4 Stability of the NP suspension as a function of NaCl and CaCl2 concentration: (a) CeO2 NPs, (b) bentonite clay colloids, and (c) CeO2 NPs in the presence of clay colloids. | |
In the presence of clay colloids, the CCC value has shifted from 26.5 mM to 16.2 mM and 7.9 mM to 1.87 mM for NaCl and CaCl2 respectively (Fig. 4). As inferred from the obtained CCC values, in the presence of clay colloids, it can be ruled out that the homo-aggregation of CeO2 NPs and bentonite clay colloids cannot be the only possible phenomenon, because the CCC value obtained individually for both CeO2 NPs and bentonite clay colloids was higher as compared to the CCC value obtained in the combination of CeO2 NPs and bentonite clay colloids. The decrease in the CCC value for both the salts in the presence of bentonite clay colloids can be attributed to the hetero-aggregation in the system that can be seen from TEM images (Fig. 5). Hence hetero-aggregation can be another mechanism that resulted in a decrease of the CCC value of these particles. Hetero-aggregation of CeO2 NPs in the presence of clay colloids takes place due to collision between NPs and clay colloids. Though both faces and edges of clay particles contribute to hetero-aggregation in the system. But, as the faces of clay particles were negatively charged and CeO2 NPs possess positive charge on their surface at pH 6 and considering the high surface area of faces to edges of clay particles, it can be speculated that clay faces are the primary cause of hetero-aggregation in the system.38,39 Further details of CCC calculation have been provided in Section S3 of the ESI.†
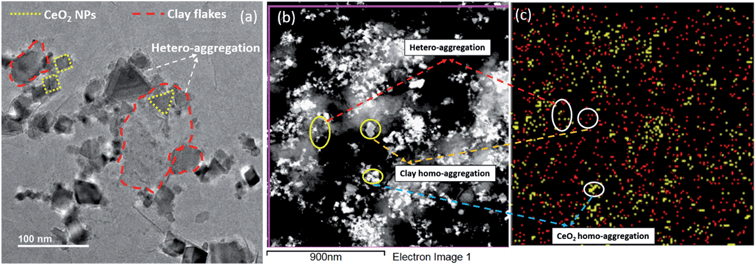 |
| Fig. 5 (a) TEM images of CeO2 in the presence of clay colloids showing hetero-aggregation, (b) STEM image of CeO2 in the presence of clay colloids showing hetero-aggregation, and (c) elemental mapping of CeO2 in the presence of clay colloids showing hetero-aggregation. | |
3.4 Effect of bicarbonates and organic matter on the stability of CeO2 NPs in the absence and presence of bentonite clay colloids
The complex matrix effect on the stability and aggregation of the CeO2 NPs at a concentration of 10 mg L−1 in the absence and presence of clay colloids was studied. The presence of bicarbonate ions induces a negative charge in the system causing CeO2 NPs to aggregate while the effect is opposite due to increased repulsion in the presence of negatively charged clay particles.
The results show the aggregation of CeO2 NPs at a concentration >0.1 mM bicarbonates in the absence of clay colloids while the presence of clay colloids reduces the effect of bicarbonate ions on the aggregation of CeO2 NPs. This behaviour may be attributed to the faster covering of the CeO2 surface with negatively charged bicarbonate ions, which can create electrostatic charge repulsion between clay and CeO2 particles (Fig. 6).
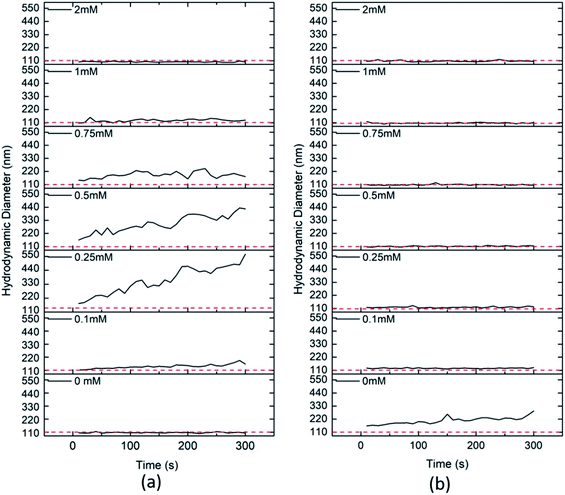 |
| Fig. 6 Aggregation kinetics of CeO2 NPs with different concentrations of bicarbonates (a) in the absence of clay colloids and (b) in the presence of clay colloids. The red dotted lines represent the initial size of blank CeO2 NPs as a reference to compare the aggregation. | |
On the other hand, the presence of different environment relevant concentrations of HA, i.e. 1, 3, 5, 10 and 20 mg L−1, generally stabilizes all the particles in the absence of clay colloids. The presence of clay colloids without HA initially caused aggregation because of electrostatic attraction between oppositely charged particles. But, the addition of humic acid even at a very low concentration, i.e. 1 mg L−1, stabilizes both the particles (Fig. 7). The stabilization can be attributed to the attachment of humic acid to the particle surface via adsorption or hydrophobic interaction, which increases the steric repulsion and electrostatic repulsion between the particles, thus providing a stabilizing effect.40
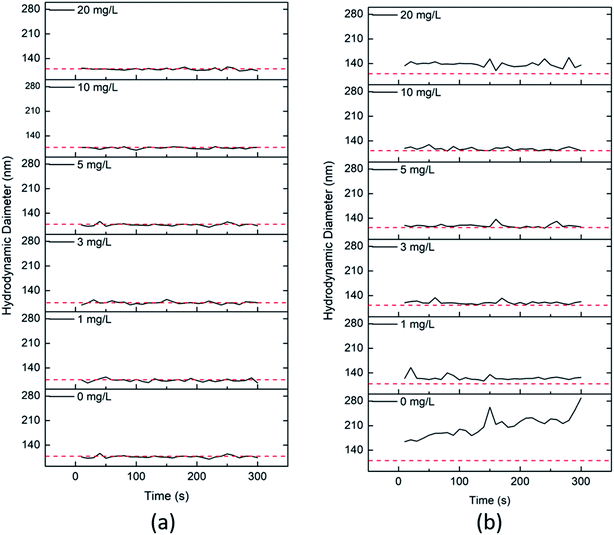 |
| Fig. 7 Aggregation kinetics of CeO2 NPs with different concentrations of humic acid (a) in the absence of clay colloids and (b) in the presence of clay colloids. The red dotted lines represent the initial size of blank CeO2 NPs as a reference to compare the aggregation. | |
The presence of both i.e. HA at a concentration of 1 mg L−1 and bicarbonate ions with varying environment relevant concentrations together represents a more complex system where a lower concentration of bicarbonate ions (i.e. <0.5 mM) resulted in the aggregation of the CeO2 nanoparticles in the absence of clay and at higher concentration stabilization was observed (Fig. 8a), whereas in the presence of clay colloids, the system got stabilized in the whole concentration range (i.e. 0.1–2 mM) of bicarbonate ions (Fig. 8b).
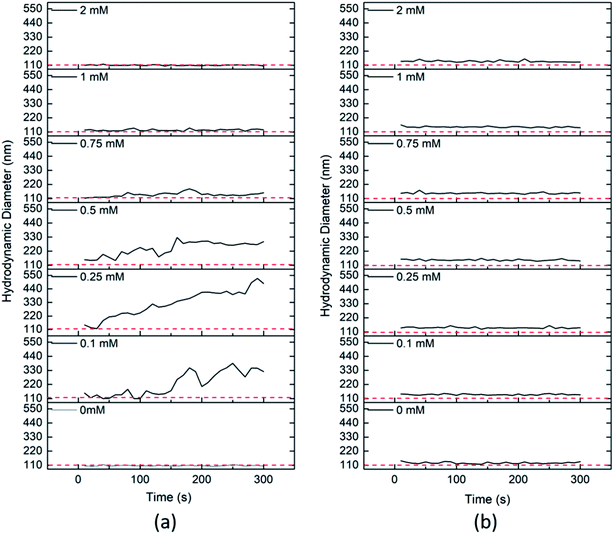 |
| Fig. 8 Aggregation kinetics of CeO2 NPs with humic acid (1 mg L−1) and different concentrations of bicarbonates (a) in the absence of clay colloids and (b) in the presence of clay colloids. The red dotted lines represent the initial size of blank CeO2 NPs as a reference to compare the aggregation. | |
The suspended particulate matter (e.g. clay colloids), bicarbonates and organic matter which are commonly found in natural surface water systems at average concentrations of 80–100 mg L−1, 0.1–5 mM, and 1–10 mg L−1 respectively32,33,41 were logical parameters to test the behaviour of NPs in the environment and used in the present study. The results show that under the above-mentioned environmental scenario, the applied CeO2 may get stabilized and further pose a threat to the aquatic environment because of the possibility to migrate and may become more bioavailable.
3.5 Effect of various types of irrigation water
3.5.1 Effect of CeO2 concentration on the charge reversal of clay colloids in complex aqueous media.
To see the behaviour of CeO2 NPs in natural aquatic matrices, NW, SW and HW were used to get the CCRC or required concentration of metal oxides above which natural clay colloids can behave as positively charged particles. As indicated in Fig. 9, the estimated CCRC values were found to be 680 mg L−1 for SW, 867 mg L−1 for NW, and 910 mg L−1 for HW. The order of CCRC values, i.e. SW < NW < HW, can be attributed to the presence of bicarbonate ions at concentration in the same order, i.e. SW (0.3 mM) < NW (1.2 mM) < HW (2 mM). As seen earlier, bicarbonate ions may attach faster to the surface of CeO2 NPs causing electrostatic repulsion and stabilization in the system. Therefore, a higher concentration of CeO2 is required to reach the CCRC in the case of HW. Data reveal that under natural environmental conditions, the application of CeO2 NPs at higher concentration in the powder form may result in the release of these particles in a high amount which in-turn may cause the charge reversal of clay particles in close proximity, leading to nutrient loss and desorption of adsorbed metal ions from the surface of clay.
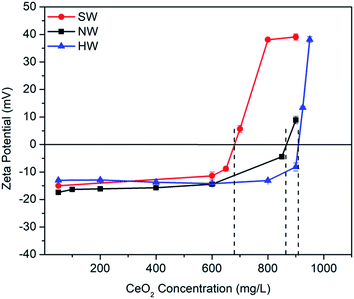 |
| Fig. 9 Zeta potential of the clay suspension (8 mg L−1) as a function of CeO2 concentration in different types of irrigation water. | |
3.5.2 Aggregation kinetics of CeO2 NPs in different aqueous samples.
The aggregation kinetics of the colloidal suspension in different environmental water samples was also determined at varying temperatures (15 °C, 25 °C and 35 °C). A statistically significant difference (p < 0.05) was observed in the aggregation behaviour of CeO2 NPs under varying ionic strength of different water samples and temperature. The result shows that the aggregation of the colloidal suspension increases with increasing IS of SW, HW and NW, independent of temperature. This is mainly due to the fact that an increase in ionic strength decreases the effective repulsion between the charged colloidal particles, resulting in faster aggregation as we have seen earlier. Maximum aggregation was found in the case HW and NW due to the presence of a high concentration of divalent cations (i.e. 1.23 mM and 0.46 mM of Ca2+ and 0.41 mM and 0.22 mM of Mg2+ for HW and NW respectively (ESI Tables 1 and 2†)). A significant effect of temperature on aggregation was observed (p < 0.05) at high ionic strength.42 An increase in temperature resulted in a slight increase in aggregation due to an increase in the collision frequency between the particles, which leads to aggregation in the case of particles having low surface potential (Section S4 of the ESI†).43 Data reveal that under natural environmental conditions, the application of bulk CeO2 may result in the release and co-transport of these particles into the environment as we can see that in the case of SW there is not much aggregation of these particles (i.e. particle size reaches a maximum of 500 nm) (Fig. 10). So, they may tend to remain suspended and get co-transported in the natural water system where the ionic strength is not very high.
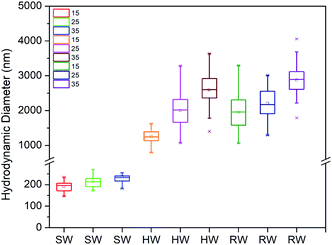 |
| Fig. 10 Aggregation of CeO2 NPs in the presence of bentonite clay colloids in environmental water samples as a function of temperature (*where, 15, 25 and 35 are temperatures in °C and SW stands for synthetic soft water, HW for synthetic hard water and RW stands for river water). | |
4. Conclusion
Considering the exposure assessment of metal oxides as an emerging nanopesticide in the agricultural environment, this study has included the sequential and combined effect of ionic strength, bentonite clay colloids, bicarbonate ion concentrations and organic matter on the aggregation behaviour of CeO2 NPs. The obtained results were used to explain the aggregation behaviour of CeO2 NPs in environmental water samples as well. The impact of bicarbonate ions and temperature on the aggregation kinetics of CeO2 NPs in environmental water samples was considered for the first time. The results showed that ionic strength was the major driving factor for the aggregation of CeO2 NPs under different environmental conditions. The aggregation was higher at high ionic strength as the CCC value obtained for the divalent salt (7.9 mM) was less than that of the monovalent salt (26.5 mM). In the presence of bentonite clay colloid, the CCC value was further decreased for both divalent (1.8 mM) and monovalent salts (16.2 mM) due to hetero-aggregation in the presence of clay colloids. Besides, bicarbonate ions at a concentration of 0.5 mM also caused aggregation of CeO2 NPs in the system, but an increased concentration of bicarbonates (0.75 mM) caused restabilization of the CeO2 NPs. However, the steric repulsion in the presence of HA caused stabilization of CeO2 NPs at a concentration of 1 mg L−1, but the stabilization effect of HA was reduced in the presence of bicarbonate ions at a concentration of 0.1 mM in the system, whereas the presence of clay colloids in combination with bicarbonate ions and HA dominated the effect of both bicarbonates and HA and caused stabilization of CeO2 NPs in the system even at 0.1 mM concentration of bicarbonate ions where aggregation was observed in the absence of clay colloids. However, the presence of a high concentration of CeO2 (>37.5 times the clay concentration) caused charge reversal in the system due to the adsorption of CeO2 NPs on the surface of clay colloids. Similar results were obtained for the aggregation kinetics of CeO2 NPs in the environmental water samples. The aggregation of CeO2 followed a sequence as: NW > HW > SW, which was directly correlated with the ionic strength of different water samples. A significant effect of temperature was observed on the aggregation kinetics of CeO2 NPs at higher ionic strength because an increase in temperature affects the kinetic energy of the particles in the system. So, we can conclude that in the soils having higher ionic strength in the presence of clay and organic matter, the CeO2 NPs may remain bound to soil. But, if they enter different water bodies due to surface runoff or infiltration, where ionic strength is lower than the CCC value of the CeO2 particles, there is a higher tendency that these formulations get stabilized and can become more mobile and bioavailable. The application of these NPs in high concentration can be more dangerous as it can result in charge reversal of the naturally present clay colloids in the environment, which in turn can result in nutrient loss and desorption of adsorbed metal ions on the clay surface. The obtained results are still not enough to determine the complete fate of nanopesticides in the environment but they dictate the importance of various environmental parameters in determining the fate of agricultural nanocomposites.
Conflicts of interest
No conflicts to declare.
Acknowledgements
This research was funded by the Science and Engineering Research Board (SERB), India – Ramanujan Fellowship grant (SB/S2/RJN-006/2016) and ECR project grant (ECR/2017/000707). We want to acknowledge the Indian Institute of Science Education and Research Kolkata's Central Instrumentation Facility for sample analysis (FESEM and TEM).
References
- B. Sekhon, Nanotechnology in agri-food production: An overview, Nanotechnol., Sci. Appl., 2014, 7, 31–53 CrossRef PubMed.
- S. Mukhopadhyay, Nanotechnology in agriculture: Prospects and constraints, Nanotechnol., Sci. Appl., 2014, 7, 63–71 CrossRef CAS PubMed.
- C. Dimkpa, J. McLean, D. Britt and A. Anderson, Antifungal activity of ZnO nanoparticles and their interactive effect with a biocontrol bacterium on growth antagonism of the plant pathogen Fusarium graminearum, BioMetals, 2013, 26, 913–924 CrossRef CAS PubMed.
- W. H. Elmer and J. C. White, The use of metallic oxide nanoparticles to enhance growth of tomatoes and eggplants in disease infested soil or soilless medium, Environ. Sci.: Nano, 2016, 3, 1072–1079 RSC.
- L. He, Y. Liu, A. Mustapha and M. Lin, Antifungal activity of zinc oxide nanoparticles against Botrytis cinerea and Penicillium expansum, Microbiol. Res., 2011, 166(3), 207–215 CrossRef CAS PubMed.
- R. Liu and R. Lal, Potentials of engineered nanoparticles as fertilizers for increasing agronomic productions, Sci. Total Environ., 2015, 514, 131–139 CrossRef CAS PubMed.
- C. Monreal, M. Derosa, S. Mallubhotla, P. S. Bindraban and C. Dimkpa, Nanotechnologies for Increasing the Crop Use Efficiency of Fertilizer-Micronutrients, Biol. Fertil. Soils, 2015, 52, 423–437 CrossRef.
- H. Wu, L. Shabala, S. Shabala and J. Giraldo, Hydroxyl radical scavenging by cerium oxide nanoparticles improves Arabidopsis salinity tolerance by enhancing leaf mesophyll potassium retention, Environ. Sci.: Nano, 2018, 5, 1567–1583 RSC.
- I. O. Adisa, V. L. Reddy Pullagurala, S. Rawat, J. A. Hernandez-Viezcas and C. O. Dimkpa, Role of Cerium Compounds in Fusarium Wilt Suppression and Growth Enhancement in Tomato (Solanum lycopersicum), J. Agric. Food Chem., 2018, 66, 5959–5970 CrossRef CAS PubMed.
- C. M. Rico, S. C. Lee, R. Rubenecia, A. Mukherjee, J. Hong, J. R. Peralta-Videa and J. L. Gardea-Torresdey, Cerium Oxide Nanoparticles Impact Yield and Modify Nutritional Parameters in Wheat (Triticum aestivum L.), J. Agric. Food Chem., 2014, 62, 9669–9675 CrossRef CAS PubMed.
- Z. Cao, C. Stowers, L. Rossi, W. Zhang, L. Lombardini and X. Ma, Physiological effects of cerium oxide nanoparticles on the photosynthesis and water use efficiency of Soybean (Glycine max (L.) Merr.), Environ. Sci.: Nano, 2017, 4, 1086–1094 RSC.
- X. Gui, Z. Zhang, S. Liu, Y. Ma, P. Zhang, X. He, Y. Li, J. Zhang, H. Li, R. Yukui, L. Liu and W. Cao, Fate and Phytotoxicity of CeO2 Nanoparticles on Lettuce Cultured in the Potting Soil Environment, PLoS One, 2015, 10(8), e0134261 CrossRef PubMed.
- A. A. Keller, S. McFerran, A. Lazareva and S. Suh, Global life cycle releases of engineered nanomaterials, J. Nanopart. Res., 2013, 15, 1–17 CrossRef.
- J. Yin, Y. Wang and L. M. Gilbertson, Opportunities to advance sustainable design of nano-enabled agriculture identified through a literature review, Environ. Sci.: Nano, 2018, 5, 11–26 RSC.
- J. Hong, J. Peralta-videa, C. Rico, S. Sahi, M. N. Viveros, J. Bartonjo, L. Zhao and J. Gardea-Torresdey, Evidence of Trans location and Physiological Impacts of Foliar Applied CeO2 Nanoparticles on Cucumber (Cucumis sativus) Plants, Environ. Sci. Technol., 2014, 48(8), 4376–4385 CrossRef CAS PubMed.
- C. Layet, M. Auffan, C. Santaella, C. Chevassus-Rosset, M. Montes, P. Ortet, M. Barakat, C. Blanche, S. Legros, M. Bravin, B. Angeletti, I. Kieffer, O. Proux, J.-L. Hazemann and E. Doelsch, Evidence that Soil Properties and Organic Coating Drive the Phytoavailability of Cerium Oxide Nanoparticles, Environ. Sci.
Technol., 2017, 51(17), 9756–9764 CrossRef CAS PubMed.
- Y. Ma, X. He, P. Zhang, Z. Zhang, Y. Ding, J. Zhang, G. Wang, C. Xie, W. Luo, J. Zhang, L. Zheng, Z. Chai and K. Yang, Xylem and Phloem Based Transport of CeO2 Nanoparticles in Hydroponic Cucumber Plants, Environ. Sci. Technol., 2017, 51, 5215–5221 CrossRef CAS PubMed.
- J. Li, R. V. Tappero, A. S. Acerbo, H. Yan, Y. Chu, G. V. Lowry and J. M. Unrine, Effect of CeO2 nanomaterial surface functional groups on tissue and subcellular distribution of Ce in tomato (Solanum lycopersicum), Environ. Sci.: Nano, 2019, 6, 273–285 RSC.
- M. Rui, C. Ma, J. C. White, Y. Hao, Y. Wang, X. Tang, J. Yang, F. Jiang, A. Ali, Y. Rui, W. Cao, G. Chen and B. Xing, Metal oxide nanoparticles alter peanut (Arachis hypogaea L.) physiological response and reduce nutritional quality: a life cycle study, Environ. Sci.: Nano, 2018, 5, 2088–2102 RSC.
- J. Fang, X.-q. Shan, B. Wen, J.-m. Lin and G. Owens, Stability of titania nanoparticles in soil suspensions and transport in saturated homogeneous soil columns, Environ. Pollut., 2009, 157, 1101–1109 CrossRef CAS PubMed.
- M. Baalousha, Aggregation and disaggregation of iron oxide nanoparticles: Influence of particle concentration, pH and natural organic matter, Sci. Total Environ., 2009, 407, 2093–2101 CrossRef CAS PubMed.
- A. A. Keller, H. Wang, D. Zhou, H. S. Lenihan, G. Cherr, B. J. Cardinale, R. Miller and Z. Ji, Stability and Aggregation of Metal Oxide Nanoparticles in Natural Aqueous Matrices, Environ. Sci. Technol., 2010, 44, 1962–1967 CrossRef CAS PubMed.
- J. T. K. Quik, I. Velzeboer, M. Wouterse, A. A. Koelmans and D. van de Meent, Heteroaggregation and sedimentation rates for nanomaterials in natural waters, Water Res., 2014, 48, 269–279 CrossRef CAS PubMed.
- E. M. Hotze, T. Phenrat and G. V. Lowry, Nanoparticle aggregation: challenges to understanding transport and reactivity in the environment, J. Environ. Qual., 2010, 39, 1909–1924 CrossRef CAS PubMed.
- D. Lin, X. Tian, F. Wu and B. Xing, Fate and Transport of Engineered Nanomaterials in the Environment, J. Environ. Qual., 2010, 39(6), 1896–1908 CrossRef PubMed.
- G. K. Darbha, C. Fischer, J. Luetzenkirchen and T. Schäfer, Site-Specific Retention of Colloids at Rough Rock Surfaces, Environ. Sci. Technol., 2012, 46, 9378–9387 CrossRef CAS PubMed.
- G. Krishna Darbha, C. Fischer, A. Michler, J. Luetzenkirchen, T. Schäfer, F. Heberling and D. Schild, Deposition of Latex Colloids at Rough Mineral Surfaces: An Analogue Study Using Nanopatterned Surfaces, Langmuir, 2012, 28, 6606–6617 CrossRef CAS PubMed.
- K. M. Spark and R. S. Swift, Effect of soil composition and dissolved organic matter on pesticide sorption, Sci. Total Environ., 2002, 298, 147–161 CrossRef CAS PubMed.
- W. J. Rawls, Y. A. Pachepsky, J. C. Ritchie, T. M. Sobecki and H. Bloodworth, Effect of soil organic carbon on soil water retention, Geoderma, 2003, 116, 61–76 CrossRef CAS.
- M. Uddin, A review on the adsorption of heavy metals by clay minerals, with special focus on the past decade, Chem. Eng. J., 2016, 308, 438–462 CrossRef.
- E. J. Smith, W. Davison and J. Hamilton-Taylor, Methods for preparing synthetic freshwaters, Water Res., 2002, 36, 1286–1296 CrossRef CAS PubMed.
-
J. J. Cole and Y. T. Prairie, in Reference Module in Earth Systems and Environmental Sciences, Elsevier, 2014, DOI:10.1016/b978-0-12-409548-9.09399-4.
-
E. M. Thurman, in Organic Geochemistry of Natural Waters, Springer Netherlands, Dordrecht, 1985, pp. 7–65, DOI:10.1007/978-94-009-5095-5_2.
- E. Tombácz and M. Szekeres, Colloidal behavior of aqueous montmorillonite suspensions: the specific role of pH in the presence of indifferent electrolytes, Appl. Clay Sci., 2004, 27, 75–94 CrossRef.
- F. Abdolahpur Monikh, A. Praetorius, A. Schmid, P. Kozin, B. Meisterjahn, E. Makarova, T. Hofmann and F. von der Kammer, Scientific rationale for the development of an OECD test guideline on engineered nanomaterial stability, NanoImpact, 2018, 11, 42–50 CrossRef.
-
J. H. Adair, E. Suvaci and J. Sindel, in Encyclopedia of Materials: Science and Technology, ed. K. H. J. Buschow, R. W. Cahn, M. C. Flemings, B. Ilschner, E. J. Kramer, S. Mahajan and P. Veyssière, Elsevier, Oxford, 2001, pp. 1–10, DOI:10.1016/b0-08-043152-6/01622-3.
- R. A. French, A. R. Jacobson, B. Kim, S. L. Isley, R. L. Penn and P. C. Baveye, Influence of Ionic Strength, pH, and Cation Valence on Aggregation Kinetics of Titanium Dioxide Nanoparticles, Environ. Sci. Technol., 2009, 43, 1354–1359 CrossRef CAS PubMed.
-
E. Tombácz, T. Nyilas, Z. Libor and C. Csanaki, Progress in Colloid and Polymer Science, Springer, Berlin, Heidelberg, 2004, vol. 125 Search PubMed.
- L. Delavernhe, A. Steudel, G. K. Darbha, T. Schäfer, R. Schuhmann, C. Wöll, H. Geckeis and K. Emmerich, Influence of mineralogical and morphological properties on the cation exchange behavior of dioctahedral smectites, Colloids Surf., A, 2015, 481, 591–599 CrossRef CAS.
- B. Joo Reginald Thio, D. Zhou and A. Keller, Influence of natural organic matter on the aggregation and deposition of titanium dioxide nanoparticles, J. Hazard. Mater., 2011, 189, 556–563 CrossRef PubMed.
- J. Vidmar, T. Zuliani, P. Novak, A. Drinčić, J. Ščančar and R. Milačič, Elements in water, suspended particulate matter and sediments of the Sava River, J. Soils Sediments, 2017, 17, 1917–1927 CrossRef CAS.
- N. Singh, E. Tiwari, N. Khandelwal and G. K. Darbha, Understanding the stability of nanoplastics in aqueous environments: effect of ionic strength, temperature, dissolved organic matter, clay, and heavy metals, Environ. Sci.: Nano, 2019, 6, 2968–2976 RSC.
- S. García-García, S. Wold and M. Jonsson, Effects of temperature on the stability of colloidal montmorillonite particles at different pH and ionic strength, Appl. Clay Sci., 2009, 43, 21–26 CrossRef.
Footnote |
† Electronic supplementary information (ESI) available. See DOI: 10.1039/c9em00428a |
|
This journal is © The Royal Society of Chemistry 2020 |
Click here to see how this site uses Cookies. View our privacy policy here.