Partial renewal of granular activated carbon biofilters for improved drinking water treatment
Received
11th October 2017
, Accepted 6th February 2018
First published on 8th February 2018
Abstract
There is a trend of increasing natural organic matter (NOM) in raw drinking waters of Nordic countries due to climate change. Seasonal deterioration in NOM quality imparts challenges for delivering a consistently high drinking water quality. In this study, a simple and cost effective operational strategy was investigated that improved short-term NOM removal in a full-scale treatment plant. Three granular activated carbon (GAC) media biofilters were modified by replacing a small fraction of saturated filter media with new media. Relative to replacing the entire biofilter media, this approach required lower capital cost and shorter downtime, and maintained conditions for biological filter functioning. NOM removal efficiencies were compared in modified versus unmodified (reference) filters using online UV absorbance, and offline fluorescence and dissolved organic carbon measurements. The modified biofilters showed improved organic matter removal lasting for at least four weeks. Partial replenishment of GAC in full-scale biofilters may be a useful and sustainable operational strategy for coping with temporarily high NOM loads in raw waters that might otherwise cause water quality problems.
Water impact
Biologically activated carbon (BAC) filters are an integral part of many drinking water facilities that improve the stability of distributed water quality. This study investigates a promising, cost-effective strategy to improve short-term natural organic matter (NOM) removal by full-scale BAC filters.
|
1 Introduction
Drinking waters around the world are often produced from surface waters that contain natural organic matter (NOM) and pathogens. NOM impacts drinking water treatment causing problems with treatment process control and optimization, and increases coagulant requirements and disinfection dose.1 NOM further affects drinking water quality by forming carcinogenic disinfection by-products (DBPs) in combination with chlorine, promoting bacterial regrowth within the distribution system, and causing undesirable colour, taste and odor.2 Seasonal periods of elevated NOM and organic pollutant concentrations in raw water due to e.g. heavy rainfall, snow melt runoff, or lake turnover and algae bloom, lead to rapid changes in raw water quality,3 affecting treatment efficiency and making it difficult to meet water-quality standards year-round.4,5
For economic and practical reasons, efforts to improve the removal of NOM in continuously-operating treatment plants are usually directed towards optimizing and improving existing treatment steps instead of implementing costly upgrades.6 Granular activated carbon (GAC) filtration is a common component of drinking water treatment plants (DWTPs) that can effectively remove NOM from drinking water as well as trace organic contaminants, including pesticides, taste- and odor-causing compounds (in particular geosmin and 2-MIB), and reduce DBP precursors.7,8 Owing to their high adsorption capacity, GAC filters remove small- to intermediate-sized humic-like NOM fractions.9,10 However, the adsorption capacity of new GAC diminishes rapidly, and GAC filters convert naturally to biologically activated carbon (BAC) filters over time.11 Due to the high specific surface area of GAC absorbers,12 biofilms form that adsorb organic molecules and shelter microbes that additionally perform biological degradation. This biofilm effectively consumes biodegradable organic matter (BDOC) and removes certain organics more effectively than GAC, promoting a stable water quality in the drinking water distribution network.13–16
One possible way to improve short-term NOM removal by BAC (or saturated GAC) filters is to replace a small fraction of the filter media with new media (new GAC). Furthermore, if effective, this may be an economical solution compared to replacing the entire filter media due to lower investment cost and a shorter interruption to normal operations. The retention of a large proportion of saturated filter media maintains biological filter functioning, while the new GAC improves the filter's adsorption capacity. Unlike powdered activated carbon, this technique produces minimal sludge and since the removed GAC can be regenerated, this is a more sustainable solution. Despite these possible advantages, the technique is not commonly used in DWTPs and to the best of our knowledge, has not been addressed by the scientific literature.
NOM removal by activated carbon filters is traditionally assessed by measuring specific absorbance (SUVA), colour, turbidity, total organic carbon (TOC) and/or dissolved organic carbon (DOC).9,17,18 UV254 is often used as a surrogate for TOC and is strongly correlated with regulated DBP formation potential (DBP-FP) in drinking waters and organic micro pollutant removal in wastewaters.19,20 However, DOC, UV254 and SUVA are bulk properties that do not distinguish between different NOM fractions, and do not track low-molecular-weight aliphatic compounds.21 Previous studies show that humic fractions of NOM are removed more effectively by adsorption,22 whereas protein-like fractions are removed more effectively by biodegradation.23 These fractions can be distinguished sensitively using fluorescence spectroscopy.23–25
In this study, a short-term strategy for enhancing NOM removal was investigated whereby a small fraction of saturated filter media was replaced with new GAC in a full-scale drinking water treatment plant. Using continuous UV254 monitoring we addressed the question: how does partial GAC replenishment influence NOM removal by saturated GAC filter media, and how long does this effect last? Using fluorescence spectroscopy, we further addressed the question: which NOM fractions are influenced the most by GAC replenishment and what are the possible mechanisms in play?
2 Materials and methods
2.1 Treatment plant description
This study was carried out during summer period (July–August, 2015) at Mariebergs DWTP in Uddevalla municipality, Sweden. The DWTP has an average treatment capacity of 11
300 m3 per day. The DWTP receives surface water from Lake Köperödsjö, which receives its water from a small stream running through farmland. This treatment plant was selected since it experiences odor episodes during summer months and has detected geosmin and 2-methylisoborneol suggesting an algal source leading to seasonal issues with undesirable taste and odor in the finished drinking water. In the years before this study was initiated, the DWTP in response, had converted several rapid sand filters into BAC filters, which is a common odor-reduction strategy in Sweden.26,27
Marieberg DWTP uses processes consisting of direct filtration by continuous-upflow rapid sand filters (DynaSand®) with polyaluminum chloride for coagulation. The Dynasand filters (n = 46), are located in two separate halls containing 24 filters (Hall A, Fig. 1) and 22 filters (Hall B, Fig. 1), respectively. The coagulation contact time differs between these two halls and results in a slightly better coagulation performance in Hall B, and a pH difference between Hall A and B (Table 1). Following direct filtration, water from both halls enter seven GAC filters (placed in parallel) in down-flow mode (Fig. 1). After GAC filtration, filtered water passes through two-step disinfection by UV irradiation and chlorine dioxide (the chlorine dioxide dose in finalized water, leaving the DWTP was 0.11 mg l−1 as the water leaves the DWTP).
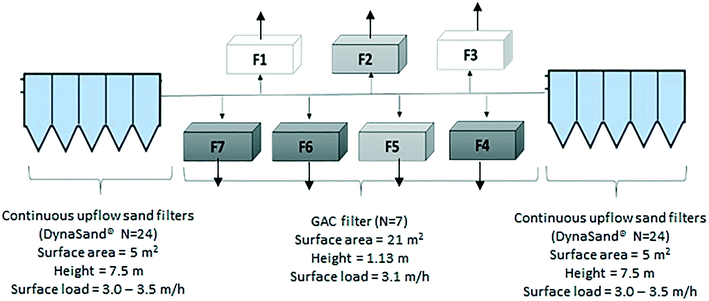 |
| Fig. 1 Schematic of continuous-upflow sand filters and parallel downstream GAC filters in this study. Dark grey boxes (F4, F6, and F7) are modified GAC filters, light grey boxes (Rf2 and Rf5) are reference filters. Additional filters exist (F1 and F3), but were not studied in this experiment. | |
Table 1 Incoming water quality, and properties of the existing and replenished GAC material
Incoming water quality and operational parameters of the BAC filters |
|
Units |
Hall A |
Hall B |
Influent DOC |
mg L−1 |
3.1–3.7 |
3.0–3.6 |
Influent pH |
— |
6.4–6.9 |
6.3–6.7 |
Influent UV254 |
cm−1 |
0.053–0.059 |
0.047–0.053 |
Temperature |
°C |
17.2–18.6 |
17.2–18.6 |
Turbidity |
NTU |
0.01–0.11 |
0.02–0.11 |
Surface load |
m h−1 |
3.1–3.5 |
3.1–3.5 |
Empty bed contact time |
min |
20–22 |
20–22 |
GAC properties |
|
Unit |
Existing GAC |
Added GAC |
Carbon type |
|
Aquasorb 1000 |
UniVar DGF 12 × 40–55 |
Carbon source |
|
Bituminous coal |
Bituminous coal |
Particle size |
mm |
0.80–1.2 |
0.42–1.7 |
Apparent density |
kg m−3 |
510 |
520 |
Mean particle diameter |
mm |
1.4 |
1 |
GAC depth |
m |
0.9 |
0.1 |
At the time of this study, the DWTP was operating without any significant variations in the operation of treatment processes, except for the modification of the three GAC filters. Incoming raw water parameters were stable during the experimental period with DOC in the range of 8.0–9.9 mg C L−1 and UV254 in the range of 0.35–0.38 cm−1. After DynaSand filtration, the DOC levels were reduced by 65 ± 4% and UV254 was decreased by 85 ± 4%.
2.2 GAC filters
Marieberg DWTP has seven BAC filters that receive the filtrate from the upstream DynaSand filters. Water from the two halls enters the seven BAC filters from two opposite directions (Fig. 1). The media in the GAC filters consist of three layers. Before any modification to the filters, the top layer had 1 m of GAC (Aquasorb 1000, 0.8–1.2 mm), the middle layer had 0.05 m of sand (2–4 mm), and the bottom layer had 0.08 m of coarser sand (4–8 mm).
Backwashing of the filters were performed every 96 hours using finished water from the DWTP, to wash out captured flocs and limit the biological growth on the filters. All filters contained aged GAC (>3 years) before any modifications were carried out. Therefore, it can be assumed that each filter was acting as a physical and biological filter with little to no adsorption.28
2.3 Experimental design
Five GAC filters were studied, of which three were modified (F4, F6 and F7) during the experiments and two were kept as unmodified controls (reference filters Rf2 and Rf5). Since there was no sampling point immediately prior to the GAC filters, an numerical model was built using EPANET29 to calculate the amount of water entering each filter from the separate DynaSand halls. This indicated that Hall A supplied water to filters F1, F6 and F7 while Hall B supplied water to filters F3, F4 and Rf5. Reference filter Rf2 mainly received water from Hall A (73% from Hall A). Therefore, the modified filters F6 and F7 were compared with reference filter Rf2 and the modified filter F4 was compared with reference filter Rf5 (Fig. 1).
During the experiments in this study, the GAC filters were modified as follows: in the modified GAC filters, 10% bed height of saturated GAC was replaced with new GAC (600 kg of GAC by weight) which had been soaked in clean water for 24 hours to remove any adsorbed air within the filter media.30 During the modification process, the modified filter was kept out of operation and flow to the other filters was increased. To avoid disrupting the overall provision of drinking water during the experiment, modifications were carried out sequentially for different filters. The sequence of GAC replenishment was as follows: F6 (June 26–27), F7 (July 1–2) and F4 (July 2–3). The process of replenishing a filters with new GAC took approximately 40 h and included the following steps:
1. Attaching a hydraulic pump and eductor to remove the top layer of GAC slurry from the filter by maneuvering a suction hose around the filter. Pumping time is proportional to the mass of GAC that needs to be removed. In this study, removing 10% bed height took approximately 3–4 h.
2. Distributing the soaked new GAC on top of the old filter materials. Distributing time is proportional to the mass of GAC to be replaced; in this study, this step took approximately 3–4 hour.
3. Eliminating dark “carbon fines”31 from the new GAC by repeated backwashing of the filter. Due to high fine particulates, the first filtrate is normally discarded. Backwashing time is proportional to the mass of GAC replenished (in this study, 4 × 20 min).
4. Before returning the filter to service it is usual to check biological water quality30,31 (by e.g. analysis of E.coli). In this study, waiting for the result of the biological water quality added a 24 h delay.
Properties of replaced and existing GAC are presented in Table 1. The replenished GAC was produced from a coal-based source by steam activation (Table 1). The new GAC has microporous structure, thus is expected to favour the sorption of small organic compounds relative to larger ones.10 Since the existing GAC and added GAC are of similar density and size, they mix thoroughly during the backwashing process so that the new GAC becomes distributed throughout the BAC filter.30 It is important that added the density of added GAC matches the existing GAC and uniformly distributes throughout the filter bed. Otherwise, the filter media will not be cleaned thoroughly or GAC will be washed out during backwashing.
2.4 Sampling
Continuous monitoring of UV254 absorbance was used to detect real-time changes in filter performance due to GAC addition. A spectrophotometer probe (Spectro::lyser™ i::scan, Messtechnik GmbH) was installed at the outlet of the GAC filters. The probe was inserted in a flow-through chamber in which the filtrate water from the seven GAC filters passed through in sequence so that filtered water from each filter was measured for 10 minutes every 70 minutes. Additional grab samples for DOC and fluorescent dissolved organic matter (FDOM) measurement were obtained weekly from seven sampling locations, consisting of filtrates from DynaSand Hall A and Hall B and filtrates from three modified and two reference GAC filters (Fig. 1). Grab samples were collected and filtered using inline capsule filters (Opticap® XL4 Durapore® 0.22 μm), which were flushed with 4 L ultrapure water (Milli-Q) prior to each sampling occasion and with 1 L of sample before collection. Samples were collected in ashed 40 mL amber glass vials. FDOM and DOC samples were collected in separate vials. FDOM samples were collected in triplicate, stored at 4 °C and analysed within 7 days. DOC samples were taken in duplicate, acidified to pH 2 with 2 M HCl within 24 hours of collection and stored in a freezer at −20 °C until analysis.
2.5 Analytical methods
A fluorescence spectrophotometer (Aqualog, Horiba Inc.) was used to measure FDOM fluorescence in a 1 cm per cell at 20 °C. Fluorescence excitation-emission matrixes (EEMs, N = 138) were measured by scanning the excitation wavelengths from 220–500 nm while emission was detected from 250–600 nm. Processing of fluorescence data followed established methodologies.32 Briefly, this included spectral correction and blank subtraction to remove Raman and Rayleigh scatter as well as correction for primary and secondary inner filter effects. Fluorescence intensities were normalized to the area under the water Raman peak at 350 nm thereby converting them to Raman units (R.U.).
DOC was measured using a Shimadzu TOC-VCPH carbon analyser. DOC concentrations were calculated using a five-point calibration curve for potassium phthalate standard solutions (1.0–10.0 mg C L−1) followed by subtraction of Milli-Q blank.
2.6 Statistical methods and data analysis
Two methods were used for interpreting the fluorescence data. The first examined differential EEMs, obtained by subtracting the EEM of a modified GAC filter from its reference filter. This helps to visualize fluorescence removal over the complete range of excitation and emission wavelengths.33 Differential EEMs were calculated according to eqn (1): | Differential EEM = EEMReferencefilter − EEMNewGACaddedfilter | (1) |
Secondly, a more advanced statistical method for interpreting fluorescence EEMs called parallel factor analysis (PARAFAC) was used.34 Fluorescence EEMs consist of overlapping signals that can be separated by multiway analysis.35 PARAFAC modelling is one type of multiway analysis that extracts from EEMs the relative intensities (Fmax) of a limited number of independent components, each having unique excitation and emission spectra. PARAFAC modelling was performed according to standard methods using the drEEM Toolbox 0.2.0.35 The models were generated for 3 to 6 components and the appropriate number of PARAFAC components were identified using a range of techniques including random split-half analysis and by comparing the obtained spectra to published studies using the OpenFluor database,36 which contains 71 studies from a range of aquatic environments (e.g., marine, terrestrial, wastewater, drinking water).
The removal efficiencies in the GAC filters for DOC, UV254 and fluorescence components were calculated using eqn (2)–(4):
|  | (2) |
|  | (3) |
|  | (4) |
Here Fmax refers to the maximum intensity of a PARAFAC component in Raman units.
To determine how long the effect of GAC addition persisted, the average deviation in absorbance between the reference and modified filter (i.e. UV254,Reference − UV254,modified) was compared. The modified filter was considered to have returned to its original condition when the average deviation after modification was within 5% of the average deviation before modification.
3 Results and discussion
3.1 Comparison of UV254 absorbance of modified and reference biofilter filtrate samples
The positive effect of GAC replenishment was seen by comparing filtrates from modified filters with reference filters on the basis of continuous UV254 measurements (Fig. 2a and 3a). In Fig. 2, reference filter Rf5 can be compared with modified filter F4. Before replenishing GAC in F4, the average deviation was −0.03 m−1, indicating that filter F4 was performing marginally worse at removing UV absorbing NOM fractions. Four weeks after modification when monitoring was ceased, F4 was still performing better than the reference filter with an average deviation of +0.25 m−1. Thus the effect of replenishing 10% GAC bed height improved filter performance lasted for at least four weeks.
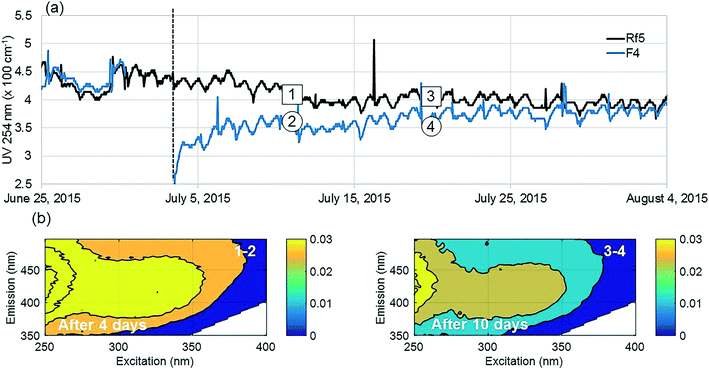 |
| Fig. 2 Water quality in filtrates from modified (F4: blue line) and reference (Rf5: black line) filters. Vertical dotted lines denote filter start-up after GAC replenishment. Numbers denote grab sampling of Rf5 (squares) and F4 (circles). (a) UV254 (m−1) measured continuously; (b) fluorescence EEMs (in Raman unit) from filter F4 minus EEMs from filter Rf5. Data for 30 days are presented here. | |
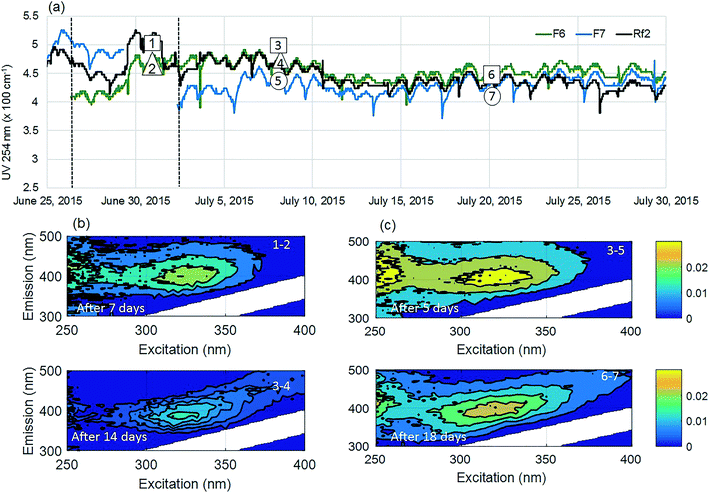 |
| Fig. 3 Water quality in filtrates from modified (F6: green line, F7: blue line) and reference (Rf2: black line) filters. Vertical dotted lines denote filter start-up after GAC replenishment. Numbers denote grab sampling of Rf2 (squares), F6 (triangles) and F7 (circles). (a) UV254 (m−1) measured continuously; (b) fluorescence EEMs from filter F6 minus EEMs from filter Rf2, (c) fluorescence EEMs from filter F7 minus EEMs from filter Rf2. Data for 30 days are presented here. | |
Similarly, before they were modified, filter F7 was performing worse than the reference filter Rf2, with an average deviation of −0.5 m−1 (Fig. 3). Four weeks after modification when the experiment ended, it was performing marginally better than the reference filter (−0.10 m−1) than it had performed before new GAC was added. The deviation could not be calculated for the modified filter F6 due to insufficient data prior to filter modification; however, a similar pattern was observed with a temporary improvement in UV254 removal after filter replenishment.
Dissolved molecules contributing to UV254 absorbance include humic-like aromatics with conjugated double bonds, which are the main precursors of regulated DBPs as well as organic micro pollutants18–20 and potentially, some unregulated DBPs.9,37 Relative reductions of UV254 by modified filters were highest (15–80%) for the first 10 days compared to reference filters (Fig. 2a and 3a). Filter F4 was modified last and showed improvements for longer than the other filters, possibly since it did not receive an increased surface load while other filters where out of operation.
3.2 Comparison of DOC measurements of modified and reference biofilter samples
DOC removal by the reference filters in this study was moderate initially (12–14%) and improved only marginally after GAC replenishment (18–23%). Previous work shows that new GAC filters typically remove around 40–90% of DOC through adsorption, depending on incoming water quality.38–41 After formation of biofilm, DOC removal by adsorption decreases gradually and both adsorption onto biofilm and biological degradation process contribute to DOC reduction at a lower removal efficiency (15–45%).23,42–44 In our study, the low DOC removal can be explained by the fact that the GAC in the filters was over three years old, although the relatively high DOC loading (Table 2) would have caused saturation within few months of regeneration.21
Table 2 DOC concentration in incoming water and filtrates from GAC filters
Sample location |
DOCa (mg C L−1) |
Removal efficiency (%) |
DOC removal measured from weekly sampling data averaged over one month after GAC replenishment.
|
Filtrate from Hall A |
3.5 ± 0.2 |
— |
Reference GAC (Rf2) |
3.0 ± 0.2 |
14 |
Modified GAC (F6) |
2.8 ± 0.1 |
20 |
Modified GAC (F7) |
2.7 ± 0.2 |
23 |
Filtrate from Hall B |
3.3 ± 0.2 |
— |
Reference GAC (Rf5) |
2.9 ± 0.4 |
12 |
Modified GAC (F4) |
2.7 ± 0.1 |
18 |
At the end of experiments, the relative DOC removal continued to be 15–45% higher by modified filters than the reference filters. Since biodegradation is the main removal mechanism within the reference filters, this additional removal by the modified filter is probably due to combination of biological degradation and adsorption.
3.3 Comparison of FEEM measurements of modified and reference biofilter filtrate samples
Fluorescence monitoring extended the results from UV254 and DOC monitoring by revealing changes in NOM composition. Differential EEM (EEMReferencefilter − EEMModifiedfilters) represents the portion of fluorescence removed after GAC replenishment by the modified filter. Differential EEM showed that during the first few weeks following GAC replenishment, modified filters were more efficient in removing all types of fluorescent organic matter (i.e. the complete range of excitation and emission wavelengths) compared to reference filter (Fig. 2b and 3b and c). With filter running time, as the active sites of new GAC became exhausted, the modified filters gradually returned to their original performance which is visible from the changes in intensity of the differential EEMs (Fig. 2b and 3b and c). The differential EEM results shows that modified filters were most efficient at removing FDOM with excitation/emission wavelengths Ex/Em of <250, 340/410–450 nm and <250, 290/340–380 nm region. These fluorescence peaks with emission <380 nm are generally attributed to protein-like fluorescence while peaks with emission >380 nm are attributed to humic-like FDOM.45–48
PARAFAC analysis revealed three independently-varying fluorescent components were present in the samples (Fig. 4). Cross-referencing the components C1–C3 with the OpenFluor database36 identified statistically similar signals in other studies. The first identified component, C1, with Ex/Em of <250, 330 nm/480 nm appears in many studies where it is believed to originate from terrestrial humic material.46,49,50 The emission at longer wavelengths suggests that C1 comprises of conjugated, aromatic, larger molecules with several functional groups.51,52 The second identified component, C2, with Ex/Em of <250, 330 nm/410 nm has fluorescence characteristic of productive freshwaters impacted by agriculture and of terrestrial humic-like NOM modified by microbial reprocessing.36,53 The third identified component, C3, with Ex/Em of <250, 290 nm/360 nm has a tryptophan protein-like character and may be associated with algal or microbial-derived organic matter and anthropogenic inputs.36,54,55
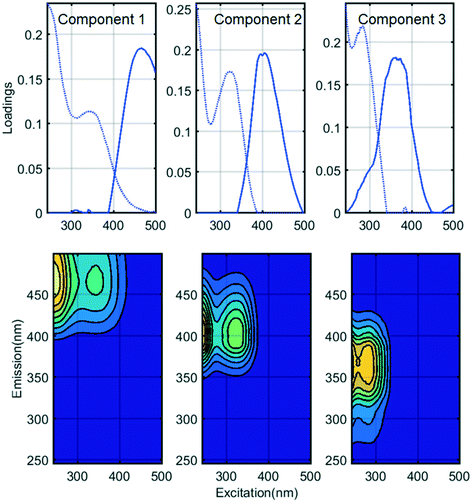 |
| Fig. 4 Spectral properties of the three independent fluorescence components identified by PARAFAC in the DWTP dataset. Top: Excitation (dotted line) and emission (solid line) spectra for each component. Bottom: Fluorescence fingerprints; colors denote relative intensity. | |
Previously all of these identified PARAFAC components have been found to correlate with regulated and unregulated DBPs precursors.56–59 Thus their presence could indicate a risk for producing DBPs in chlorinated finished water.
3.4 Removal efficiency of NOM components in modified filters
Overall removal efficiencies of all optically-active (absorbing/fluorescing) NOM and DOC by the GAC filters are illustrated in Fig. 5. Error bars on this figure represent standard error calculated by the error propagation method60 and are smallest for fluorescence measurements. Fig. 5 shows that the biofilters in this study preferentially removed FDOM fractions compared to both the wider DOM pool represented by DOC, and the UV-absorbing fractions that did not fluoresce. The relatively low removal of DOC indicates that a large fraction of the DOM pool consists of non-fluorescent fractions with relatively poor removal. However, removal of all three fractions including DOC improved following GAC replenishment, indicating a positive overall effect on NOM removal. Since there would be no biological removal associated with the new GAC, the increased removal is most-likely attributable to adsorption onto the new GAC filter material.
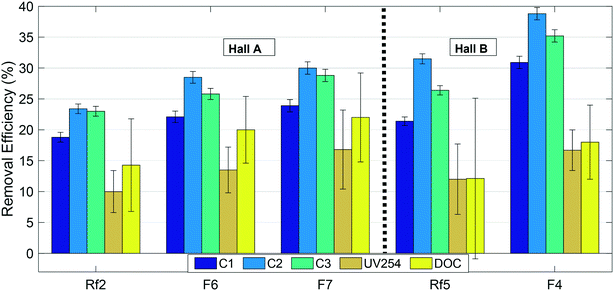 |
| Fig. 5 Removal efficiencies of NOM fractions in modified (F6, F7 and F4) and reference (Rf2 and Rf5) filters. Error bars indicate standard errors calculated by error propagation (N = 4). | |
For fluorescent NOM, removal efficiencies in all modified and reference filters followed the trend C2 > C3 > C1, i.e. were highest for the microbial or processed humic-like component C2, lowest for the “terrestrial” humic-like component C1, and intermediate for the protein-like fraction (Fig. 5). This trend has previously been observed in BAC filter studies53,57,61 where it was concluded that C3 and C2 were more bioavailable and hence better removed by biodegradation than terrestrial humic-like component (C1). At the same time, other trends have been observed, for example, Peleato et al. observed highest removal of C3, while Fu et al. observed lowest removal of C3.18,62 These differences may be a function of different types of GAC supporting different biological communities, or may reflect differences in NOM composition in the incoming water. In this study, all the filters receiving water from Hall B showed better removal of fluorescent fractions and poorer removal of non-fluorescent fractions than all the filters receiving water from Hall A.
Previous work indicates that new GAC is most efficient at removing small to intermediate sized NOM fractions, because the GAC surface does not admit large-sized and condensed DOM.63–66 Longer-wavelength emitting components like C1 are generally believed to reflect a larger size fraction than shorter-wavelength emitting components like C2,67 which is consistent with the lower removal efficiency of C1 compared to C2. On the other hand, this does not explain the relatively poor removal of DOC, much of which consists of substances with lower molecular weights than humic acids.68 If biological processing on the GAC filters converts fluorescent organic matter to non-fluorescent (and UV absorbing) soluble microbial products, this could account for the relatively high removal of FDOM despite low removal of DOC.
GAC replenishment caused a short-term improvement in the filters' capacity to remove NOM. Our results indicate differences in the treatment efficacy of various NOM fractions and differences in their removal mechanisms. Because only part of the GAC was replaced, the biological activity of the majority of the BAC was retained. Whether the modifications affected the biological activity of the remaining BAC in any way cannot be reliably determined from this study; in future, it would be worthwhile to study the effect of replacing different amounts of GAC to determine how much GAC can be replaced before the biodegradation function is unduly hampered.
3.5 Economic aspects of GAC replenishment
From economic perspective, adding a layer of GAC is much more economical than regenerating or replacing a complete GAC filter. While there are some fixed costs associated with modifying a GAC filter (e.g. administration and set-up time, acquisition and installation of pumps, biological tests), the majority of costs are proportional to the amount of GAC that will be replaced (e.g. new GAC purchase, extraction and distribution pump time, backwashing time, transportation and disposal costs). The operation cost for BAC systems is far less than for GAC systems with estimated savings around 2–3 fold including a 4–5 fold extension of service life.69 Since the strategy maintained 90% of the filter media within the existing BAC filters, biodegradation continued to contribute to NOM removal at the same time that adsorption was boosted by new GAC. For many DWTPs, this simple strategy may be preferable to alternative options that would deliver similar improvements due to its relatively low cost and ease of implementation. Data obtained from Marieberg DWTP as well as a second DWTP in Sweden that recently implemented a similar strategy estimated costs in the range of 1750–2300 USD per m3 of GAC. Costs for DWTPs in other locations would be expected to vary depending on the total amount of GAC to be replaced and local labour, transport and waste management costs.
4 Conclusion
• Experiments performed on GAC filters at full-scale DWTPs indicated that replenishing a small fraction (10% bed height) of saturated filter media with new filter media temporarily improved the removal of various organic matter fractions. Some variations in performance could be attributed to variations in incoming water quality and flow rates.
• Online monitoring of UV254 absorbance for four weeks following GAC replenishment indicated enhanced removal of the UV-absorbing NOM fraction lasting at least four weeks. The UV-absorbing fraction is known to include precursors of regulated DBPs.
• In all filters, removal efficiencies for fluorescent organic matter fractions followed the trend C2 > C3 > C1, i.e. highest for the microbial or processed humic-like component C2, lowest for the terrestrial humic-like component C1, and intermediate for the protein-like fraction.
• In all filters, DOC was removed much less efficiently than fluorescent fractions suggesting that some fluorescent organic matter was converted to non-fluorescent organic matter in the filter media.
• Partial GAC replacement is a relatively simple and cost-efficient strategy that could deliver short-term improvements in NOM removal to cope with temporarily strained operating conditions.
Abbreviations
BDOC | Biodegradable organic matter |
BAC | Biological activated carbon |
DBP | Disinfection-by-product |
DBP-FP | DBP formation potential |
DOC | Dissolved organic carbon |
DWTPs | Drinking water treatment plants |
EEMs | Fluorescence excitation-emission matrixes |
FDOM | Fluorescent dissolved organic matter |
GAC | Granular activated carbon |
NOM | Natural organic matter |
PARAFAC | Parallel factor analysis |
R.U. | Raman units |
SUVA | Specific absorbance |
TOC | Total organic carbon |
UV254 | UV absorption at 254 nm |
Conflicts of interest
The authors declare no competing financial interest.
Acknowledgements
This research was funded by the National drinking water research center DRICKS at Chalmers University of Technology, Göteborg Stad (Kretslopp och Vatten), Swedish Water and Wastewater Association (SVU), Västvatten AB, VIVAB, Trollhättan Energi, Sydvatten and Norrvatten. The authors would like to thank the Marieberg DWTP (Västvatten AB) staff for their valuable and fruitful collaboration and especially Elisabet Edlund (water plant operator during the experimental period) for her cooperation in the collection of the water samples.
References
- A. Matilainen, E. T. Gjessing, T. Lahtinen, L. Hed, A. Bhatnagar and M. Sillanpaa, Chemosphere, 2011, 83, 1431–1442 CrossRef CAS PubMed.
- P. C. Singer, Water Sci. Technol., 1999, 40, 25–30 CAS.
- E. Hood, D. M. McKnight and M. W. Williams, Water Resour. Res., 2003, 39, 1188 CrossRef.
- S. A. Parsons, B. Jefferson, E. H. Goslan, P. R. Jarvis and D. A. Fearing, Water Sci. Technol.: Water Supply, 2005, 4, 43–48 Search PubMed.
-
R. Vogt, Increase in colour and amount of organic matter in surface waters, In NORDTEST Position paper, 2003 Search PubMed.
- M. R. Collins, G. L. Amy and P. H. King, J. Environ. Eng., 1985, 111, 850–864 CrossRef CAS.
- G. Newcombe, J. Morrison and C. Hepplewhite, Carbon, 2002, 40, 2135–2146 CrossRef CAS.
- C. Kim, S. I. Lee, S. Hwang, M. Cho, H.-S. Kim and S. H. Noh, Journal of Water Process Engineering, 2014, 4, 91–98 CrossRef.
- J. L. Weishaar, G. R. Aiken, B. A. Bergamaschi, M. S. Fram, R. Fujii and K. Mopper, Environ. Sci. Technol., 2003, 37, 4702–4708 CrossRef CAS PubMed.
- T. Karanfil and J. E. Kilduff, Environ. Sci. Technol., 1999, 33, 3217–3224 CrossRef CAS.
- P. Servais, G. Billen and P. Bouillot, J. Environ. Eng., 1994, 120, 888–899 CrossRef CAS.
- R. M. Hozalski, S. Goel and E. J. Bouwer, J. - Am. Water Works Assoc., 1995, 87, 40–54 CrossRef CAS.
- W. H. Joe, I. C. Choi, Y. A. Baek, Y. J. Choi, G. S. Park and M. J. Yu, Water Sci. Technol., 2007, 55, 111–116 CrossRef CAS PubMed.
- S. Velten, M. Boller, O. Köster, J. Helbing, H.-U. Weilenmann and F. Hammes, Water Res., 2011, 45, 6347–6354 CrossRef CAS PubMed.
-
S. Velten, Adsorption capacity and biological activity of biological activated carbon filters in drinking water treatment, ETH, 2008 Search PubMed.
-
P. M. Huck, Optimizing filtration in biological filters, American Water Works Association, 2000 Search PubMed.
-
AWWA, Operational Control of Coagulation and Filtration Processes, American Water Works Association, 3rd edn (M37), 2011 Search PubMed.
- J. Fu, W.-N. Lee, C. Coleman, M. Meyer, J. Carter, K. Nowack and C.-H. Huang, Chemosphere, 2017, 166, 311–322 CrossRef CAS PubMed.
- P. C. Singer and S. D. Chang, J. - Am. Water Works Assoc., 1989, 81, 61–65 CrossRef CAS.
- J. Altmann, A. S. Ruhl, F. Zietzschmann and M. Jekel, Water Res., 2014, 55, 185–193 CrossRef CAS PubMed.
- A. Matilainen, N. Vieno and T. Tuhkanen, Environ. Int., 2006, 32, 324–331 CrossRef CAS PubMed.
- S. Ciputra, A. Antony, R. Phillips, D. Richardson and G. Leslie, Chemosphere, 2010, 81, 86–91 CrossRef CAS PubMed.
- N. M. Peleato, M. McKie, L. Taylor-Edmonds, S. A. Andrews, R. L. Legge and R. C. Andrews, Chemosphere, 2016, 153, 155–161 CrossRef CAS PubMed.
- K. R. Murphy, A. Hambly, S. Singh, R. K. Henderson, A. Baker, R. Stuetz and S. J. Khan, Environ.
Sci. Technol., 2011, 45, 2909–2916 CrossRef CAS PubMed.
- R. K. Henderson, A. Baker, K. R. Murphy, A. Hambly, R. M. Stuetz and S. J. Khan, Water Res., 2009, 43, 863–881 CrossRef CAS PubMed.
-
B. Johansson, Dricksvattenteknik 3 (Publikation U8) Ytvatten, Svenskt Vatten, 2011 Search PubMed.
-
F. Persson, G. Heinicke, T. Hedberg, O. Bergstedt, C. Wångsell, H. Rydberg, I. Kjellberg and S.-E. Kristenson, Mikrobiologiska barriärer i vattenrening, Stockholm, 2005 Search PubMed.
- J. Kim and B. Kang, Water Res., 2008, 42, 145–152 CrossRef CAS PubMed.
-
L. A. Rossman, EPANET: Model for Water Distribution Piping Systems, US Environmental Protection Agency, 2008 Search PubMed.
-
Z. K. Chowdhury, R. S. Summers, G. P. Westerhoff, B. J. Leto, K. Nowack and C. J. Corwin, Activated carbon: solutions for improving water quality, American Water Works Association, 2013 Search PubMed.
-
A. F. Hess, M. J. Chipps and A. J. Rachwal, Filter maintenance and operations guidance manual, American Water Works Association, 2002 Search PubMed.
- K. R. Murphy, K. D. Butler, R. G. M. Spencer, C. A. Stedmon, J. R. Boehme and G. R. Aiken, Environ. Sci. Technol., 2010, 44, 9405–9412 CrossRef CAS PubMed.
- E. E. Lavonen, M. Gonsior, L. J. Tranvik, P. Schmitt-Kopplin and S. J. Köhler, Environ. Sci. Technol., 2013, 47, 2264–2271 CrossRef CAS PubMed.
- R. Bro, Chemom. Intell. Lab. Syst., 1997, 38, 149–171 CrossRef CAS.
- K. R. Murphy, C. A. Stedmon, D. Graeber and R. Bro, Anal. Methods, 2013, 5, 6557–6566 RSC.
- K. R. Murphy, C. A. Stedmon, P. Wenig and R. Bro, Anal. Methods, 2014, 6, 658–661 RSC.
- Y. Tan, T. Lin, F. Jiang, J. Dong, W. Chen and D. Zhou, Chemosphere, 2017, 181, 569–578 CrossRef CAS PubMed.
- D. S. Chaudhary, S. Vigneswaran, H.-H. Ngo, W. G. Shim and H. Moon, Korean J. Chem. Eng., 2003, 20, 1054–1065 CrossRef CAS.
- B. W. Dussert and G. R. Van Stone, Water Eng. Manage., 1994, 141, 22–24 Search PubMed.
-
J. Lohwacharin, Y. Yang, N. Watanabe, A. Phetrak, H. Sakai, M. Murakami, K. Oguma and S. Takizawa, presented in part at the WA Specialty Conference on Natural Organic Matter, Costa Mesa, CA, USA, 2011 Search PubMed.
- E. E. Lavonen, D. N. Kothawala, L. J. Tranvik, M. Gonsior, P. Schmitt-Kopplin and S. J. Kohler, Water Res., 2015, 85, 286 CrossRef CAS PubMed.
- B. W. Dussert and G. R. Van Stone, Water Eng. Manage., 1994, 141, 22–24 Search PubMed.
- W. H. Kim, W. Nishijima, E. Shoto and M. Okada, Water Sci. Technol., 1997, 35, 147–153 CAS.
- M. B. Emelko, P. M. Huck, B. M. Coffey and E. F. Smith, J. - Am. Water Works Assoc., 2006, 98, 61–73 CrossRef CAS.
- P. G. Coble, C. E. Del Castillo and B. Avril, Deep Sea Res., Part II, 1998, 45, 2195–2223 CrossRef CAS.
- P. G. Coble, C. E. Del Castillo and B. Avril, Deep Sea Res., Part II, 1998, 45, 2195–2223 CrossRef CAS.
- M. Li, X. Sun, F. Qian, H. Xu and Y. Liu, Asian J. Chem., 2013, 25, 10095–10101 CAS.
- A. Li, X. Zhao, R. Mao, H. Liu and J. Qu, J. Hazard. Mater., 2014, 271, 228 CrossRef CAS PubMed.
- P. G. Coble, Mar. Chem., 1996, 51, 325–346 CrossRef CAS.
- Y. Yamashita and R. Jaffé, Environ. Sci. Technol., 2008, 42, 7374–7379 CrossRef CAS PubMed.
- J. Hur and G. Kim, Chemosphere, 2009, 75, 483–490 CrossRef CAS PubMed.
- B. M. Lee, Y. S. Seo and J. Hur, Water Res., 2015, 73, 242–251 CrossRef CAS PubMed.
- S. A. Baghoth, S. K. Sharma and G. L. Amy, Water Res., 2011, 45, 797–809 CrossRef CAS PubMed.
- D. M. Reynolds and S. R. Ahmad, Water Res., 1997, 31, 2012–2018 CrossRef CAS.
- N. Hudson, A. Baker and D. Reynolds, River Res. Appl., 2007, 23, 631–649 CrossRef.
- K. M. H. Beggs, R. S. Summers and D. M. McKnight, J. Geophys. Res.: Biogeosci., 2009, 114, 10 Search PubMed.
- N. M. Peleato and R. C. Andrews, Water Sci. Technol.: Water Supply, 2015, 15, 589–598 CrossRef CAS.
- D. W. Johnstone, N. P. Sanchez and C. M. Miller, Environ. Eng. Sci., 2009, 26, 1551–1559 CrossRef CAS.
- B. Hua, K. Veum, J. Yang, J. Jones and B. Deng, Environ. Monit. Assess., 2010, 161, 71–81 CrossRef CAS PubMed.
-
J. C. Pezzullo, Propagation and Compounding of Errors, http://statpages.info/erpropgt.html, (accessed January 18, 2018).
- S. Peldszus, C. Hallé, R. H. Peiris, M. Hamouda, X. Jin, R. L. Legge, H. Budman, C. Moresoli and P. M. Huck, Water Res., 2011, 45, 5161–5170 CrossRef CAS PubMed.
- N. M. Peleato, B. S. Sidhu, R. L. Legge and R. C. Andrews, Chemosphere, 2017, 172, 225–233 CrossRef CAS PubMed.
- E. Vuorio, R. Vahala, J. Rintala and R. Laukkanen, Environ. Int., 1998, 24, 617–623 CrossRef CAS.
- A. Matilainen, N. Vieno and T. Tuhkanen, Environ. Int., 2006, 32, 324–331 CrossRef CAS PubMed.
- C. J. Corwin and R. S. Summers, Environ. Sci. Technol., 2010, 44, 5403–5408 CrossRef CAS PubMed.
- J. E. Kilduff, T. Karanfil, Y.-P. Chin and W. J. Weber, Environ. Sci. Technol., 1996, 30, 1336–1343 CrossRef CAS.
- S. K. L. Ishii and T. H. Boyer, Environ. Sci. Technol., 2012, 46, 2006–2017 CrossRef CAS PubMed.
- F. Zietzschmann, C. Stützer and M. Jekel, Water Res., 2016, 92, 180–187 CrossRef CAS PubMed.
- C.-K. Lin, T.-Y. Tsai, J.-C. Liu and M.-C. Chen, Water Res., 2001, 35, 699–704 CrossRef CAS PubMed.
|
This journal is © The Royal Society of Chemistry 2018 |
Click here to see how this site uses Cookies. View our privacy policy here.