Uptake and impacts of polyvinylpyrrolidone (PVP) capped metal oxide nanoparticles on Daphnia magna: role of core composition and acquired corona†
Received
15th January 2018
, Accepted 8th June 2018
First published on 11th June 2018
Abstract
The potential long-term environmental impact of manufactured nanoparticles (NPs) remains poorly understood, in part due to the complexity of NPs themselves and the range of physico-chemical parameters that may influence their biological impacts (such as size, shape and chemistry), as well as their dynamic interactions with their environment, leading to acquisition of an eco-corona and a range of other possible transformations. A key hypothesis in nanosafety assessment is that the NP core chemistry is a primary factor controlling toxicity. This work aims to compare the uptake and impacts of 5 nm zinc oxide (ZnO) NPs which are highly soluble and cerium dioxide (CeO2) NPs which are considered to be poorly soluble, where both particles were produced using an identical protocol and capped with polyvinylpyrrolidone (PVP), on Daphnia magna (D. magna). These well-characterised NPs were developed as part of a systematic library, and were intended to allow controlled variation of one property at a time, e.g. the core composition as evaluated here. Half-maximal effective concentrations (EC50) were determined in pure medium and medium conditioned with biomolecules secreted by D. magna, as the presence of biomolecules in the environment has the ability to alter NP stability and biological effects. NPs were characterised by size and zeta potential measurements under both conditions. NP uptake concentrations and removal over 24 hours post exposure (without feeding) were determined by inductively coupled plasma – optical emission spectroscopy (ICP-OES) of the exposure and receiving media, respectively. Results showed that PVP capped ZnO NPs were more toxic than PVP capped CeO2 NPs. The NP impact and behaviour was due to physical effects with CeO2 NPs which showed signs of agglomeration while a chemical effect was apparent in the case of ZnO NPs which underwent partial dissolution in the gut following uptake.
Environmental significance
Daphnia magna (D. magna) are an important environmental indicator species who may be especially sensitive to nanoparticles (NPs) as a result of being filter-feeders. Utilising an identical synthesis method for PVP capped zinc oxide and cerium dioxide NPs allowed us to assess the contribution of core chemistry to the mode of action of the NPs in D. magna. While both NPs were taken up by D. magna for the first 12 hours of exposure, behaviour diverged over the rest of the 24 hour exposure period. In the case of the PVP capped CeO2 NPs, further uptake of particles was not observed, likely as a result of the NPs becoming lodged within the bush border of the D. magna making the organisms feel full and thus inhibiting further uptake. By contrast, the PVP capped ZnO NPs continued to be taken up and subsequently dissolved once within the gut due to the low pH in this microenvironment, thus exhibiting a “Trojan Horse” effect. Dissolution was enhanced by the presence of the acquired corona. Thus, the proteins released by D. magna create an eco-corona which affects the NP stability, their attractiveness as a food source, and subsequently influences the mode of toxicity of the NPs.
|
1. Introduction
NP toxicity testing is becoming increasingly important due to the widespread use of NPs of diverse chemical compositions in a variety of industrial applications.1–3 Increasing production and usage volumes leads to an increased likelihood of NPs being deposited into the environment, either during production, product use or upon disposal. Once in the environment, NPs may undergo transformations such as dissolution (and potentially re-precipitation as different species and/or with different morphologies) altering them from their pristine state.4 Additionally, the presence of natural biomolecules in a dynamic environment may cause significant changes to NP properties and behaviour, and must be evaluated as part of their toxicity assessment. Biomolecules present in their surroundings coat NP surfaces as a means of reducing their surface energy, thereby creating a biological or ecological ‘corona’ which ultimately changes the identity of the NP as organisms no longer see the bare NP but rather the corona-coated particle.5–8 The acquired corona can cause changes to the morphology and stability of the NPs which may make the NP more likely to bind to biological receptors or more available as a food source.8
Typical chemical toxicity testing involves obtaining dose–response relationships between cells or organisms subjected to known doses of chemicals over a period of time. These results then allow for the determination of appropriate dosages for drugs and acceptable limits for exposure to pollutants.9 Similar studies are carried out for NPs and nano-specific protocols already exist for many end-points. However, it should be noted that nanotoxicology is complex, and effects are not necessarily linearly related to dose,10 and can depend on numerous factors and properties including size, composition, surface charge, concentration, coating and the fact that NPs have a tendency to agglomerate at higher concentrations which alters the bioavailable dose. For this reason the development of NP libraries is critical, in order to be able to systematically vary NP properties and hence test and isolate the toxicological effects of a single variable.11
Over and above NP properties, there are several environmental factors that need to be considered also.12 For instance, NP surfaces have a high adsorption capacity leading to binding of natural organic matter (NOM) or organism-secreted biomolecules, which changes the NP surface properties and interactions.8 Cho et al.13 showed that sedimentation of NPs can influence how many NPs are taken up by cells in an in vitro assay, and agglomeration and sedimentation can have striking differences when considering NP uptake by whole organisms. Due to these potential transformations, characterisation of the NPs in the test media is important for a better understanding of toxicity pathways.14–17
This study looks at the toxicity of two NPs prepared using an identical synthesis method, and capped with identical amounts of the same capping agent, namely 5 nm PVP-capped ZnO and CeO2 NPs from a NP library that was designed to test the hypothesis that core chemistry is the driving factor for toxicity. These NPs were chosen due to their extensive uses in industry. For example, ZnO is used in numerous sectors including rubber, ceramics, chemicals, paints, agriculture, electronics and pharmaceuticals among others. It is used for preparing solar cells, gas sensors, chemical absorbent varistors, electrical and optical devices, electrostatic dissipative coatings, as a catalyst for liquid phase hydrogenation, and a catalyst for photo-catalytic degradation instead of titania NPs.18 It is also an approved sunscreen ingredient in many countries around the world due to its UV-absorbing properties.19 Ceria was first commercially used in 1999 as a catalyst in diesel particulate filters20 and nowadays its uses are numerous. These include optical, micro-electronic, solid oxide fuel cells, solar cells, catalysis, bio-medical applications, oxidation resistance, UV absorbents and filters, anti-reflective coatings, abrasives for chemical mechanical planarization and metallurgical and glass/ceramic applications.21–30 Due to their widespread use, the likelihood of these NPs being deposited into environmental waters is increasing and their interaction with organisms such as the fresh water zooplankton D. magna, a filter feeding primary consumer, is inevitable.
The organisation of economic development and cooperation (OECD) have standardised tests which aim to assess chemical (including NPs) toxicity towards D. magna.31,32 These standardised protocols do not take into account the complexity of natural waters, which contain NOM arising from decaying plants and organisms, which has a high affinity to bind to NP surfaces. D. magna themselves release biomolecules into their surrounding medium including chitin, derived from their exoskeleton every time moulting, occurs as well as an abundance of proteins present in the moulting fluid.33 It has been shown that D. magna steadily secrete proteins into their surrounding medium for up to six hours reaching ∼435 μg mL−1 (based on 10 neonates, 1–3 days old, in 5 mL of a hard water, see Fig. 1 for schematic illustration of the conditioning process).8 Biomolecules can have destabilising or stabilising effects on NPs, which can cause them to agglomerate and become a more or less attractively sized food source for D. magna which in turn can drastically affect their toxicity.8 It was previously observed that the secreted proteins caused agglomeration of polystyrene NPs, which lead to increased retention, and thus our working hypothesis is that bigger particles (closer to the algae size) are more recognisable and potentially more attractive as a food source, though NP dissolution also needs to be taken into account for many NP types. The addition of natural biomolecules during NP toxicity studies should be considered in NP exposure protocols and are taken into consideration in this study. Furthermore, whole organisms such as D. magna are themselves dynamic systems and therefore potential changes to the NPs may occur once consumed in, for example, the gut, which can be considered as a sub-environment where NPs may be altered.
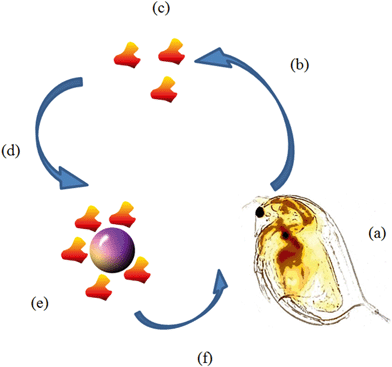 |
| Fig. 1 Schematic of EC50 conditioning/incubation experiment. (a) 10 D. magna juveniles are placed in 2 mL of HH Combo medium (b) D. magna condition medium and release proteins for 0, 12 or 24 hours. (c) At the end of the conditioning time D. magna neonates are removed leaving behind only the conditioned medium containing proteins (d) NPs are dispersed using the conditioned medium to reach the EC50 concentration. (e) NPs are incubated in the conditioned medium for 0, 12 or 24 hours allowing the proteins to bind to the NP creating an eco-corona. (f) 20 fresh D. magna juveniles are exposed to the corona coated NPs for 24 hours and then survival is counted. | |
The aim of this work is to assess the impact of identically sized (DLS size = c. 5 nm) well-characterised metal (cerium and zinc) oxide NPs having the same capping (PVP) and prepared via the same synthesis route, on juvenile D. magna survival. The approach taken was to correlate the survival with the amount of NPs internalised and retained, and to investigate the role of the secreted biomolecule corona, taking into account both the medium conditioning time (i.e. the length of time over which D. magna juveniles released biomolecules into the medium prior to dispersion of the NPs) as well as the length of time allowed for the biomolecules to interact with the NPs and form a corona prior to exposure to the D. magna juveniles.
2. Materials and methods
2.1
D. magna culturing
Genetically identical D. magna (Bham 2 strain) were cultured in high-hardness (HH) Combo media (pH 7.6–7.8) adapted from Baer et al.34 A complete list of the salt ingredients can be found in Table S.1 in the (ESI†). The medium was then aerated and pH adjusted, following which 4 mL of animate (Table S.2†) and 2 mL vitamins (Table S.3†) were added. 15 adults were contained in each culturing vessel containing 900 mL of media. The vessels were kept at 20 °C in a culturing chamber with maintained humidity. D. magna cultures were fed Chlorella vulgaris (C. vulgaris) algae daily (1 mL days 1–2, 1.5 mL day 3 onwards; note: 1 mL algae = 0.5 mg carbon at optical density (OD) = 0.8). The media was refreshed weekly in order to maintain healthy broods. Juveniles of age 1–7 days were collected before use.
During the NP exposure experiments (section 2.4), a higher number of D. magna per volume was utilised (20 D. magna per 2 mL) and several control steps were implemented to ensure that this did not introduce stress to the daphnids. There was a complete absence of any of the typical indicators of short term stress, with no observation of altered haemoglobin levels or erratic swimming behaviour, indicating no effect of the smaller exposure volume on juvenile daphnids' well-being. Additionally, the control D. magna were put back into regular culture conditions after the 24 hour experiment and grown to full adults which then went on to produce healthy (all female) neonates of their own. Control D. magna grew to a healthy size and maintained a regular life span indicating that the short time being put into a small volume did not induce any long term effects.
Given the difference in the size between daphnids of 1 day old compared to 7 days old, an even distribution of age ranges were incorporated into each sample. This ensured no size and thus no age effect with regards to uptake and depuration. Considering this work also deals with the release of proteins by D. magna, the proteins released by day 1 as well as day 7 were identified by mass spectrometry and can be found in ESI† Table S.4.
2.2 Media conditioning and NP incubation
Media was conditioned by D. magna by placing 10 juveniles (1–7 days old) in 2 mL of HH Combo media for a specified ‘conditioning (C) time’ (0, 12 or 24 h). Conditioning time was started when the daphnids were put into the HH Combo medium and allowed to secrete proteins. Daphnids were not fed in order to ensure the proteins accumulated in the medium were only proteins released by the daphnids. Following the conditioning step, the organisms were removed from the medium by carefully pipetting them out of the medium leaving only the conditioned medium. NPs were then incubated at the EC50 concentrations (refer to section 2.4 indicating how this was calculated) using the conditioned medium as a dispersion liquid and incubated for either 0, 12 or 24 hours allowing proteins to interact with the NPs. For 0 hour conditioning, incubation (I) was done with fresh HH Combo medium (no proteins).
2.3 NP synthesis and characterisation
Cerium nitrate hexahydrate, zinc nitrate hexahydrate, acetone, and 10k PVP were all used as received from suppliers. 10k PVP capped CeO2 and ZnO were synthesised according to Briffa et al.11 Details of the characterisation of the pristine NPs (dynamic light scattering (DLS), zeta potential, ultra-violet visible light spectroscopy (UV-VIS), X-ray photoelectron spectroscopy (XPS), Fourier transform infra-red (FT-IR) and transmission electron microscopy (TEM)) are reported in the same paper.11 Characterisation at time 0 and 24 hours has also been conducted in HH Combo medium (Table S.5 for CeO2 and Table S.6† for ZnO NPs). The fresh NP pellet resulting from the synthesis, following an acetone wash to remove excess PVP, having a concentration of 2.030 ± 0.480 mg L−1 for CeO2 and 0.960 ± 0.137 mg L−1 for ZnO as determined by ICP-OES, was retained. Any remaining acetone was allowed to evaporate and the pellet was then dispersed in medium (25 mL in the case of CeO2 and 12.5 mL in the case of ZnO) rather than DI water.11 The concentrations of the resulting NP stock solutions were 0.4 mg L−1 (PVP capped CeO2 or ZnO NPs).
2.4 Assessing NP impact on D. magna – EC50 determination
The NP stock solutions were diluted with HH Combo media to various concentrations ranging between 0–0.4 mg L−1 for CeO2 and 0–0.1 mg L−1 for ZnO, to ensure a span in terms of the percentage survival observed. Twenty D. magna juveniles (1–7 days old) were exposed for 24 hours to 2 mL of a range of concentrations of either PVP capped CeO2 or PVP capped ZnO NP dispersions in order to determine dose–response behaviour and the EC50. After 24 hours the % survival was determined by counting the living organisms observed visually as showing swimming behaviour within 15 seconds of gentle agitation. The EC50 values of the 5 nm PVP capped CeO2 and PVP capped ZnO NP were determined to be 0.193 mg L−1 and 0.055 mg L−1 respectively (n = 3). Ionic controls were run in parallel using the metal nitrate salts since these were the precursor materials for the synthesis. These were carried out using an ionic metal concentration equivalent to that of the metal concentration comprising the NPs (n = 3). Negative controls, where daphnids were placed in fresh medium, had 100% survival.
2.5 NP stability in HH Combo medium
NP stability was determined at the EC50 concentrations by measuring the zeta potential on a Malvern Zetasizer ZS. Results were obtained at 21 °C following 2 minutes equilibration. Measurements were taken at time 0 and after 24 hours. 10 consecutive measurements were collected and averaged to calculate the zeta potential at the pH of the medium (pH = 7.4–7.8). Zeta potential is not a definitive indication of stability though it is a useful indicator. Stability was also assessed at 0 and 24 hours by DLS and by TEM.
2.6 Assessment of NP dissolution
A 24 hour solubility assay was performed using the approach of Avramescu et al.35 The dissolution was performed at the respective EC10 concentrations of the NPs. From the values obtained for the EC50 determination graph (Fig. 2), the EC10 was calculated. The EC10 concentrations were found to be 0.116 mg L−1 for 5 nm PVP capped CeO2 and 0.01367 mg L−1 for 5 nm PVP capped ZnO. The NPs were dispersed in 24 hour conditioned or unconditioned medium at pH 4 and 7.4 to simulate the wide range of pH values found in the D. magna gut.36 Samples were placed in a covered shaker incubator (SI-900R; JEIO TECH, S. Korea) for 12 hours at 100 rpm and then transferred to a second incubator (SI-600R, Jeio Tech; S. Korea) and left to stand for 12 hours without agitation. In both cases the temperature was kept at 20 °C to simulate the growth conditions using during the D. magna uptake experiments. At the end of the 24 hours, samples were centrifuged in vivaspin tubes (3 kDa filter) for 1 hour at 5000 rpm. 2 mL of the filtered NP dispersion samples were diluted to 2% nitric acid. Three repeats for each time sample were analysed by ICP-OES to determine the metal content in the supernatant as explained in section 2.8.
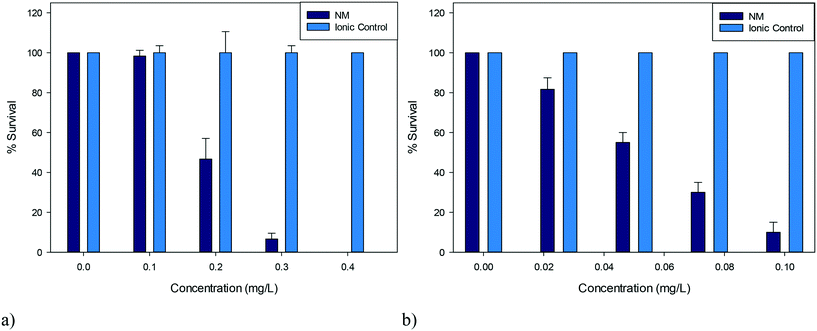 |
| Fig. 2 Percentage survival of D. magna juveniles exposed to a) 5 nm PVP capped CeO2 NPs b) 5 nm PVP capped ZnO NPs and their corresponding ionic controls (n = 3). | |
2.7 NP uptake and removal by D. magna
The EC10 concentrations were chosen to ensure a high percentage survival in order to assess NP uptake. Ten juveniles (1–7 days) were exposed to the EC10 concentrations for 0, 0.5, 1, 6, 12 and 24 hours, following which an aliquot of the NP containing media was removed for determination of the remaining NP concentration by ICP-OES (explained in section 2.8). A control experiment to assess potential for NP loss to the vessel walls was performed at the EC10 concentration in the exact same exposure vessels but without any D. magna. Concentrations of the test solutions were analysed at the exact same time points (0, 0.5, 1, 6, 12 and 24 hours).
2.8 Determination of concentration remaining in solution following NP uptake by D. magna and the amount of NP dissolution at pH 4 and 7
0.250 μL of the NP dispersion samples extracted from the exposure solutions at the time points noted above were first digested overnight in 20% nitric acid. Following this the samples were diluted to 2% nitric acid. Three repeats for each time point were analysed. ICP-OES analysis was carried out using an Optima 8000 spectrometer. Calibration standards (0.01, 0.1, 0.5 and 1 ppm) were prepared in 2% nitric acid using TraceCERT® zinc and cerium standards obtained from Sigma-Aldrich. The concentration of each of the samples was measured by comparison to the concentration of the standards, with the 0.5 ppm standard used as the calibration quality control (QC). The supernatants from the dissolution experiments were analysed in a similar manner, without the need for the overnight digestion, since in this case only the dissolved metals passed through the centrifuge tube filters.
2.9 Assessing the effect of D. magna conditioning and NP incubation time on the EC50
The same study described in section 2.4 was performed using the NPs incubated for 0, 12 or 24 hours in D. magna conditioned medium (medium exposed to 10 D. magna juveniles for 0, 12 or 24 hours followed by removal of the daphnids as described above) prior to presentation of the NPs to a new set of D. magna juveniles (1–7 days) (Fig. 1). This was carried out in order to assess the impact of eco-corona formation and rearrangement on NP toxicity, as well as how incubation time affected NP stability and bioavailability to the D. magna juveniles.
2.10 Assessment of NP size (in HH Combo medium and conditioned medium)
NP size was measured after each conditioning/incubation time point at 21 °C in HH Combo medium using a nano Zetasizer ZS (Malvern Instruments) with a red laser (633 nm). The autocorrelation function calculated the diffusion coefficient (D), which was converted to hydrodynamic radius (RH) using the Stokes–Einstein equation RH = kBT/(6πηD), where η is the dynamic viscosity of the medium and kBT is the thermal energy. 10 consecutive measurements were collected and averaged. TEM imaging was carried out using a JEOL 1200 and JEOL 1400 TEM at an accelerating voltage of 80 kV.
2.11 Hard-corona isolation and identification of proteins by mass spectrometry
PVP capped ZnO and CeO2 NPs were incubated for 0, 12 or 24 hours in 0, 12 or 24 hour conditioned medium. Following this, the hard-corona was isolated according to the method of Monopoli et al.5 Briefly, NPs were centrifuged at 15
300g for 15 minutes at 4 °C. The supernatant was removed and pellets were retained and suspended in 1 mL of phosphate buffered saline (PBS). This was repeated three times. Centrifugation removed firstly the free proteins then loose corona proteins. Pellet samples were digested by trypsin and separated by liquid chromatography (LC) prior to analysis for proteomics by mass spectrometry carried out by the Advanced Mass Spectrometry Facility at the University of Birmingham.
2.12 Statistics
Statistical significance was calculated using a standard Student's t-test. p values of 0.05, 0.01 and 0.001 were determined with *, ** and *** indicating representative significances in the data.
3. Results and discussion
3.1 Effect of CeO2 and ZnO NP on D. magna survival
D. magna juveniles were exposed to a range of concentrations of either 5 nm PVP capped ZnO or CeO2 NPs such that a range of percentage survival could be observed. It was determined that PVP capped ZnO NPs were more toxic compared to PVP capped CeO2 NPs as seen by their corresponding EC50 concentrations of 0.055 mg L−1 and 0.193 mg L−1 respectively (Fig. 2). To assess whether toxicity was due to particulate influence rather than being an ionic effect, D. magna were exposed to the corresponding Ce4+ and Zn2+ concentrations from cerium nitrate and zinc nitrate salts (which are the respective NP synthesis precursors) in parallel control experiments. Results showed that the toxicity was NP specific, since 100% survival was observed with the ionic controls at all concentrations tested (Fig. 2). Other groups have found EC50 values for ionic controls that differ from the results obtained here, however these were for hydroxide, carbonate and sulfate salts and therefore cannot directly be compared due to different dissociation values.37,38
Both types of NPs were coated in 10k PVP and it was also determined that the coating (or any non-associated PVP molecules) was not responsible for the observed toxicity, as a PVP positive control was also exposed to D. magna using the higher concentration exposures corresponding to CeO2 NPs, with any minor death being statistically insignificant (p > 0.05) (see Table S.7 in the ESI†).
3.2 Stability of NPs in HH Combo medium
The stability of the NPs in unconditioned HH Combo was studied by means of DLS. It should be noted that the DLS data are indicative of the size and are included to illustrate the trend in particle behaviour only, as the samples became far too polydisperse for reliable size identification using this technique. Size and zeta potential measurements, shown in Fig. 3 and Table 1 respectively, were taken at time 0 and after 24 hours exposure to the medium. Over this time CeO2 NPs resulted in larger sized NPs indicating slight aggregation of NPs (Fig. 3a). The HH Combo medium has a high salt content resulting in a high ionic strength medium. Since the PVP is a sterically stabilising polymer this high ionic strength medium only slightly affects NP stability. The ZnO NPs dispersed in the medium resulted in smaller sized NPs after 24 hours of exposure, possibly indicating partial dissolution of NPs (Fig. 3b). Dissolution is a well-known characteristic of ZnO NPs which will be further discussed in section 3.3. Tables S.5 and S.6 in the ESI† provide further details of the characterisation of the NPs in the HH Combo medium at 0 and 24 hours, including TEM analysis of size.
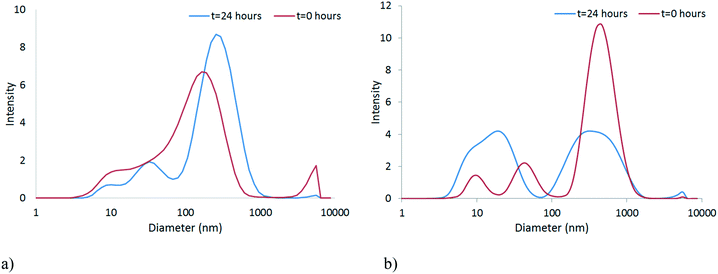 |
| Fig. 3 Size distribution graphs at time 0 and after 24 hours for a) CeO2 b) ZnO in the unconditioned HH Combo medium. | |
Table 1 Zeta potential stability of NPs in HH Combo media (pH 7.4–7.8)
|
Zeta potential at T = 0 (mV) |
Zeta potential at T = 24 (mV) |
10k PVP capped CeO2 |
−0.318 ± 0.120 |
−0.375 ± 0.096 |
10k PVP capped ZnO |
−3.85 ± 0.889 |
−2.60 ± 0.532 |
Zeta potential values at time 0 are seen to be close to 0 mV. Despite this being the isoelectric point the NPs are still stabilised through steric stabilisation due to the PVP polymer capping. After 24 hours exposed to the medium the NPs show very slight changes to the zeta potential values, but not sufficient to disturb the steric stabilisation.
3.3 Dissolution of NPs
The dissolution study aimed to analyse the dissolution behaviour of the NPs at EC10 concentrations (0.116 mg L−1 for CeO2 and 0.01366 mg L−1 for ZnO) in the conditioned and unconditioned media (pH 7.4) and mimicking the pH of the gut of the D. magna (pH 4).
In the case of CeO2 NPs at pH 7.4 there is negligible dissolution in both conditioned and unconditioned media (Fig. 4a). This is complementary to our ionic control results (Fig. 2) where no toxicity was observed. At pH 4, which simulates the pH within the gut of the D. magna, there is around 40% Ce dissolution in both conditioned and unconditioned media. Though the dissolution is similar, the toxicity of these scenarios varies, as seen in Fig. 6 below (explained in section 3.5.1) with the CeO2 NPs in the conditioned medium (24C 24I) having a lower toxicity than those in the unconditioned medium (0C 24I).
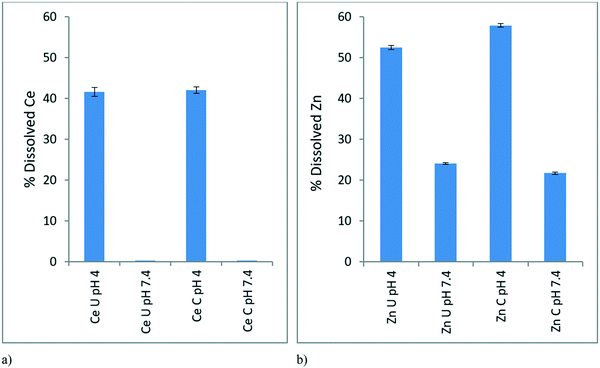 |
| Fig. 4 Average (n = 3) percentage dissolved metal released from EC10 concentrations of a) CeO2 NPs, b) ZnO NPs in conditioned (C) and unconditioned (U) medium at pH 4 and pH 7.4, as determined by ICP-OES following centrifugal separation of the ionic fraction. The dissolution of CeO2 NPs at pH 7.4 was minimal (<0.05%). | |
In the case of the ZnO NPs, dissolution occurred to a greater extent compared to CeO2 NPs as expected given that it is well-known that ZnO NPs have a high dissolution potential.39 The dissolution of ZnO NPs within the simulated gut environment (pH 4) was greater (50–60%) than in both conditioned and unconditioned medium at pH 7.4 (Fig. 4b) where dissolution was ∼25%. This indicates a “Trojan Horse” effect whereby the ZnO NPs taken by the D. magna juveniles undergo dissolution within the D. magna gut leading to the observed toxicity of the NPs. The NPs in the medium have a lower dissolution than within the gut due to the difference in pH, leading to a lower observed toxicity towards the D. magna, as ions released externally are not taken up actively (as per the lack of toxicity observed with the ionic controls) whereas ions released in the gut are in direct contact with gut tissue. Once the NPs are consumed the lower pH of the gut would drastically increase dissolution and toxicity hence the “Trojan Horse” effect. The rate of dissolution at pH 4 was slightly greater for the conditioned (58%) compared to the unconditioned media (52%) as the proteins may be facilitating ion release.
3.4 Uptake of NPs by D. magna juveniles
The uptake of the CeO2 and ZnO NPs by D. magna juveniles from the unconditioned HH Combo medium was assessed at 0, 0.5, 1, 6, 12 and 24 hours by analysing the change in metal concentration remaining in the unconditioned exposure medium (i.e. by subtraction of the amount at time, t, from the initial EC10 concentration). This was done to investigate the uptake (and potential excretion) of NPs without any additional influencing factors. The EC10 concentration was used to ensure a high survival percentage so that uptake could be monitored. These results can be seen in Fig. 5a and b for CeO2 and ZnO NPs respectively.
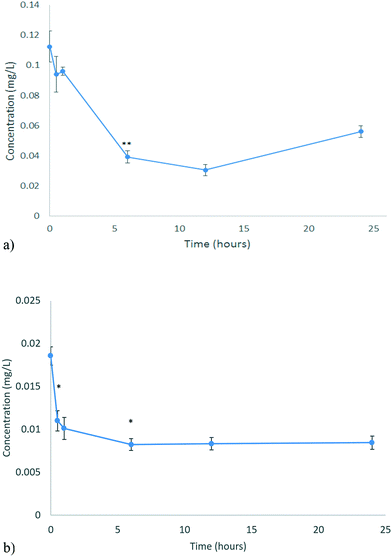 |
| Fig. 5 Uptake of NPs by D. magna for a) CeO2 b) ZnO as determined by ICP-OES analysis of the metal content remaining in the medium. Note that at longer times, some of the NPs taken up initially (or the metal from NPs that dissolved in the gut) may be released and contribute to the concentrations detected in the medium. No significant amounts of ZnO were found to absorb to the vessel walls, while c. 30% of the CeO2 was found to have absorbed at 12 and 24 hours, which is corrected for in (a). Statistical analysis for both types of NMs over time in their vessels is shown in Tables S.8 and S.9.† | |
D. magna juveniles were exposed to 0.116 mg L−1 CeO2 NPs (EC10 concentration). D. magna juveniles were found to steadily take up CeO2 NPs for the first 12 hours as shown by the decrease in the Ce concentration of the medium (corrected for the minor loss of CeO2 NPs to the vessel at longer exposure times) (Fig. 5a). After this, the concentration of Ce in the medium begins to increase again, indicating that the rate of NP excretion is faster than the rate of NP uptake. It is a well-established fact that D. magna decrease their grazing if they are already fed.40 It has been found that NPs may become lodged within the gut, in proximity to the bush border, which appears to be causing the D. magna to feel full, resulting in reduced uptake over the subsequent period.8,41 The presence of CeO2 NPs within their gut appears to decrease subsequent uptake of the CeO2 NPs after 12 hours. It is well-known that D. magna require a food source to push out previously ingested material so that the amount remaining in the gut may be cleared in real environmental scenarios where food is present. At the same time, NPs can bind to a food source, incidentally increasing their uptake such that a food source acts as a modulator of both uptake and excretion.42
D. magna juveniles were exposed to 0.01366 mg L−1 ZnO NPs (equivalent to the EC10 concentration). Living D. magna juveniles steadily take up Zn for the first 6 hours (Fig. 5b) whereby then they have reached a full body burden (steady-state) and the rate of uptake is equal to the rate of excretion. D. magna juveniles appear to still be taking up Zn as well as excreting keeping a steady state concentration of Zn within them. This is different to what was seen for CeO2 NPs as D. magna were only excreting after 12 hours. Once ZnO NPs were consumed they dissolved within the gut of the organism (section 3.3). At pH 7.4, which is the pH of the medium, the majority (75–80%) of the material taken up exists as NPs as seen from the dissolution results (Fig. 4b). The juveniles continue to take up NPs as they are not feeling full. This can be shown by the fact that there are no statistically significant changes to the Zn concentration in the medium beyond 6 hours of exposure. During these 6 hours the concentration of Zn in the medium decreased by 0.010334 mg L−1 (c. 76%).
3.5 Effect of medium conditioning by D. magna and incubation time of NPs in the conditioned medium on D. magna survival
The OECD standard protocols for assessing chemical (including NP) toxicity towards D. magna investigates toxicity in medium containing no organic components. While this works for chemicals as there are less interactions with biomolecules, for NPs this is unsuitable, given the high surface area and surface energy of NPs, which drives them to interact strongly with available biomolecules.5,43 We have previously shown that the presence of a secreted corona increased the toxicity of polystyrene NPs, by inducing some agglomeration and thus making the NPs a more attractive food source for the daphnids,8 both as a result of being closer in size to the micron sized algae that daphnids graze on normally44 as well as having an organic texture from the adsorbed biomolecules. However, the role and effect of biomolecules secreted by organisms such as D. magna on NP stability, bioavailability and toxicity is still insufficiently understood, and the present work assesses whether the effects observed with polystyrene NPs are also observed with metal oxide NPs, and whether the adsorbed biomolecule corona enhances or delays the dissolution potential of the NPs.
The effect of conditioning time (C) (the time where D. magna juveniles were allowed to secrete proteins into the surrounding medium) and incubation time (I) (time allowed for NPs to interact with the proteins present in the medium before then being exposed to new juvenile daphnids) on the toxicity of the NPs towards D. magna was investigated. This was done by comparing the percentage of daphnid survival from each scenario to the original EC50 concentration for the respective NP (as determined previously from Fig. 2) with no conditioning or incubation steps (indicated by the red bars in Fig. 6 and 7 below).
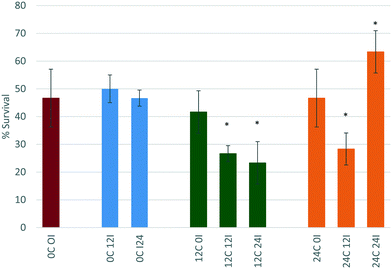 |
| Fig. 6 Effect of conditioning time (C) (0, 12, or 24 hours) and incubation time (I) (0, 12 or 24 hours) on the percentage survival of D. magna juveniles exposed to the EC50 concentration of CeO2 NPs as determined previously in unconditioned HH Combo medium. | |
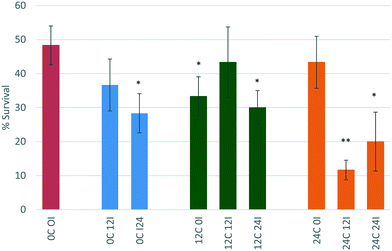 |
| Fig. 7 Effect of conditioning time (C) (0, 12, or 24 hours) and incubation time (I) (0, 12 or 24 hours) on the percentage survival of D. magna juveniles exposed to the EC50 concentration of ZnO NPs as determined previously in unconditioned HH Combo medium. | |
3.5.1 Effect of secreted corona on toxicity of CeO2 NPs to D. magna.
CeO2 NPs incubated for either 12 or 24 hours in fresh medium (0 hours conditioning, so medium that is free of proteins) show no statistical changes in terms of toxicity compared to the control (no conditioning and immediate exposure) (Fig. 6 (comparing red bar to blue bars)). This is complementary to the size results (Table 2). Table 2 indicates that for 0 hours conditioning and either 12 or 24 hours incubation (0C 12I and 0C 24I) there is no change in the primary peak size (c. 30 nm) when compared to 0 hours conditioning and 0 hours incubation (0C 0I). This indicates that in the case of the CeO2 NPs, no change in size corresponds to no change in toxicity, as expected. Therefore the length of time CeO2 NPs remain dispersed in HH Combo medium before being exposed to the daphnids does not induce any changes to the NPs in terms of their toxicity to D. magna (p > 0.05).
Table 2 Effect of conditioning (C) and incubation (I) time on NP primary peak size (hydrodynamic diameter, as determined by DLS) for the 5 nm 10k PVP capped CeO2 NPs (n ≥ 5)
Scenario |
Primary peak size (nm) |
0C 0I |
29.83 ± 5.15 |
0C 12I |
31.45 ± 4.03 |
0C 24I |
30.56 ± 4.53 |
12C 0I |
27.00 ± 9.63 |
12C 12I |
38.50 ± 22.95 |
12C 24I |
447.8 ± 419.4 |
24C 0I |
25.77 ± 5.89 |
24C 12I |
1645 ± 868.3 |
24C 24I |
11.21 ± 3.60 |
Incubation of the CeO2 NPs for 0 hours (immediate exposure after dispersion) in either 12 or 24 hour conditioned medium (12C 0I and 24C 0I), also does not change the toxicity of the NPs towards D. magna. Again, there is no change in size of the NPs, which remains at c. 30 nm suggesting that this may be too short a time period for proteins to bind to the NPs before the daphnids start filtering them, or that the proteins that bind to the NPs immediately have a stabilising effect on the NPs.
For CeO2 NPs incubated for 12 hours in 12 or 24 hour conditioned medium (12C 12I and 24C 12I, respectively), the toxicity of the NPs increases (both at p < 0.05 *) with % survival decreasing to 26.6% and 28.3% respectively. The NP size in the sample 12C 12I shows a large standard deviation (38.50 ± 22.95 nm) indicating a broad size distribution and that the proteins present in the medium had a longer time to interact with the NPs causing changes to their size distribution and toxicity. Furthermore, a maximum polydispersity index (PDI) of 1.00 ± 0.00 is seen indicating that the sample is significantly affected by the proteins present in the medium. A more polydisperse sample along with the shift to larger mean sizes implies the presence of agglomerates, which as proposed previously makes the NPs a more attractively sized food source for the D. magna. The 24C 12I scenario CeO2 NPs show a similar trend to those of the 12C 12I scenario, wherein the NPs have a long time to interact with proteins. Once again, an increase in size with a large standard deviation and a high PDI value (0.918 ± 0.128) are apparent. A longer incubation time in conditioned medium leads to an even larger particle size, reaching almost micron size.
NPs that are incubated for a duration of 24 hours in 12 hour conditioned medium (12C 24I) also have an increase in their toxicity (p < 0.05 *) when compared to the original scenario (0C 0I). This is due to the increase in size observed (447.8 ± 419.4 nm) and the higher polydispersity (PDI = 0.67 ± 0.20). However the toxicity does not seem to change when compared to the 12C 12I scenario (still p < 0.05 *) indicating the length of incubation time is not influencing the toxicity once sufficient time has passed to allow corona formation and evolution to a stable configuration.45,46
Interestingly, CeO2 NPs that are incubated for 24 hours, which is the longest incubation time, in the 24 hour conditioned medium (24C 24I) show decreased toxicity when compared to the original control (0C 0I). The size results show a significant decrease in size to a final diameter of 11.21 ± 3.60 nm. This is most likely due to the Vroman effect whereby the highest mobility and abundance proteins bind to the NP first (within the first 12 hours of incubation), these are later (between 12–24 hours) replaced by less motile proteins that have a higher affinity for the surface.12,47 These new proteins have a more stabilising effect on the NPs indicated by the smaller particle size. Based on our previous hypothesis, these smaller NPs represent a less attractive sized food source and thus are taken up less, leading to the significantly lower toxicity observed (p < 0.05 *) so that the survival is now 63% of the total exposed population. The highly dispersed CeO2 NPs (24C 24I) are still taken up, but most likely in a passive fashion as part of the water filtration, and are probably passing effortlessly through the gut, compared to the agglomerated CeO2 NPs (e.g. 12C 24I and 24C 12I) which require the peristaltic motion of the gut lumen microvilli to push them through the gut.
3.5.2 Effect of secreted corona on toxicity of ZnO NPs to D. magna.
The 5 nm PVP capped ZnO NPs that had been incubated for 12 hours (0C 12I) showed no change in toxicity compared to the control (red bar in Fig. 7). However 24 hour incubation in unconditioned medium (0C 24I) resulted in a significant decrease in survival to 28% (p < 0.05 *). This shows that without proteins present, there is no change in ZnO NP toxicity up to 12 hours, though at longer wait times of 24 hours (before exposure) the ZnO NPs undergo a slight decrease in size due to dissolution which increases their toxicity slightly as seen in Fig. 4b. As incubation time of NPs in unconditioned medium increases to 24 hours the size of the NPs decreases. This affects toxicity (Table 3) and is most likely due to dissolution. The Zn ionic control (Fig. 2) showed no influence on toxicity up to the maximum concentration tested (0.1 mg L−1). However the ZnO NPs are probably a more attractive food source and are actively consumed compared to the ions (which are passively taken up). Following this a “Trojan Horse” effect is observed, whereby following uptake of the NPs by the D. magna juveniles, the NPs dissolve within the very acidic gut as seen in Fig. 4b, and hence influence toxicity.
Table 3 Effect of conditioning (C) and incubation (I) time on NP primary peak size (hydrodynamic diameter, as determined by DLS) for the 5 nm 10k PVP capped ZnO NPs (n ≥ 5)
Scenario |
Primary peak size (nm) |
0C 0I |
20.63 ± 4.14 |
0C 12I |
16.14 ± 3.68 |
0C 24I |
14.89 ± 0.85 |
12C 0I |
202.5 ± 39.90 |
12C 12I |
27.94 ± 3.73 |
12C 24I |
26.28 ± 1.196 |
24C 0I |
31.70 ± 11.78 |
24C 12I |
277.1 ± 322.9 |
24C 24I |
41.82 ± 8.24 |
D. magna juveniles that were exposed to ZnO NPs that had been incubated for 0 hours (immediate exposure) in 12 hour conditioned medium (12C 0I) showed an increase in toxicity whereby the survival decreases to 33%. This is contrary to what was observed for the CeO2 NPs in the same scenario and is indicative that this is a core effect highlighting the different behaviour and interactions of ZnO and CeO2 NMs. The process of physical adsorption of proteins to NPs can happen immediately and only takes a few minutes48 or it could take substantially longer depending on the chemistry of the NPs. The immediate incubation of ZnO in 12 hour conditioned medium (12C 0I) causes some agglomeration of the NPs, and increases their toxicity towards D. magna (p < 0.05 *) most probably as a result of enhanced uptake of larger sized entities. The increase in NP size (to 202.5 ± 39.90 nm as per Table 3) is due to the presence of the most abundant proteins binding to the NPs causing destabilisation. This most likely causes D. magna to take up the ZnO NPs to a higher degree and once within the gut the “Trojan horse” effect is observed as a result of the low pH-driven dissolution.
Interestingly, there seems to be a rearrangement of proteins on the NP surface after 12 hours of incubation (12C 12I) as the toxicity of the NPs decreases to 43% survival compared to the 12C 0I scenario. This is a typical phenomenon governed by the previously noted Vroman effect, whereby proteins that are higher in abundance bind to the NP initially, and are later displaced by proteins which have a higher affinity for the NP. The size of the NPs is now measured to be 27.94 ± 3.73 nm (see Table 3). This may be a combined effect of NP dissolution as well as the rearrangement of different proteins on the surface. After 24 hours of incubation (12C 24I), the toxicity increases again (p < 0.05 *) indicating a possible reorganisation of proteins at the NP surface.
NPs that had been incubated for 0 hours (immediate exposure after dispersion) in 24 hour conditioned medium (24C 0I) showed no change to toxicity relative to the NPs in the unconditioned medium. The larger amount of proteins present in the medium compared to those secreted only after 12 hours of conditioning (12C 0I), appear to have had stabilising effects on the NPs, causing no changes to its toxicity. Previous studies indicate that neonates (1–3 days) release 435 μg mL−1 in 6 hours8 and this increases to 470 μg mL−1 by 24 hours.
After 12 hours of incubation in 24 hour conditioned medium (24C 12I), the toxicity of the NPs drastically increased and the percentage survival dropped to 11.6% (p, 0.01 **). NPs have had a longer duration of time to interact with the higher concentration of proteins present in the medium. This has been a sufficient time to cause changes to the NPs invoking an increase in their toxicity. The size of the NPs is very polydisperse and the PDI value is high (0.704 ± 0.214). This large size range is indicative of agglomeration which increases the NPs uptake into D. magna. Once consumed the NPs are prone to dissolution in the gut (as discussed in section 3.3).40 A 24 hour incubation in 24 hour conditioned medium (24C 24I) also shows an increase in toxicity (p < 0.05 *).
4. Conclusion
On comparing CeO2 and ZnO NPs of the same size (5 nm) and stabilised by the same capping agent (10k PVP), it was found that the PVP capped ZnO NPs were more toxic than the PVP capped CeO2 NPs with EC50 concentrations of 0.055 mg L−1 and 0.193 mg L−1 respectively, thus proving the original hypothesis that core chemistry is a driving factor of toxicity. Results showed that this toxicity was not a direct ionic effect since the ionic controls were not toxic, but was rather a particulate effect in both cases, although the specifics differ for the two particle types. Furthermore the PVP capping had no influence on toxicity.
The effect of medium conditioning time and incubation time of NPs in this medium was also assessed in terms of their effect on the NPs' toxicity. Both conditioning and/or incubation time heavily impacted NP toxicity although the trends varied for ZnO and CeO2 NPs, once again implying core chemistry as a leading factor in toxicity. Currently OCED protocols, which were originally designed for bulk materials, do not consider the presence of biomolecules and how this impacts NP stability and toxicity. NPs have a high absorption capacity where biomolecules bind to their surface creating an eco-corona. This eco-corona adds changes to the toxicity of the NPs, by changing the NP agglomeration behaviour, its dissolution behaviour, or other effects, and must be assessed as a standard aspect of NP toxicity testing.
It was seen that both NPs were taken up by D. magna for the first 12 hours. Behaviour differed based on the chemistry of the NPs. Either NPs became lodged within the bush border of the D. magna gut inhibiting further uptake, as in the case of CeO2 NPs, or NPs were taken up and subsequently dissolved once within the gut due to the low pH in this microenvironment, thus exhibiting a “Trojan Horse” effect, as in the case of the ZnO NPs.
Conflicts of interest
There are no conflicts to declare.
Acknowledgements
This work was co-financed via funding from the European Commission via FP7 grants NanoMILE (Grant no. NMP4-LA-2013-310451) and FutureNanoNeeds (Grant no. NMP-2013-604602), Horizon2020 grant ACEnano (Grant no. H2020-NMBP-2016-720952) and the Endeavour Scholarships Scheme (Group B). The authors acknowledge use of the University of Birmingham College of Life and Environmental Sciences' Daphnia research facility, supported in part by the Environmental Genomics Initiative of the University of Birmingham (UoB), and funded in part through a major UoB grant to John Colbourne, where part of the research was undertaken.
References
- Y.-C. Chuang, J.-C. Li, S.-H. Chen, T.-Y. Liu, C.-H. Kuo and W.-T. Huang,
et al., An optical biosensing platform for proteinase activity using gold nanoparticles, Biomaterials, 2010, 31(23), 6087–6095 CrossRef PubMed.
- J. Pardeike, A. Hommoss and R. H. Müller, Lipid nanoparticles (SLN, NLC) in cosmetic and pharmaceutical dermal products, Int. J. Pharm., 2009, 366(1–2), 170–184 CrossRef PubMed.
- W. Stark, P. Stoessel, W. Wohlleben and A. Hafner, Industrial applications of nanoparticles, Chem. Soc. Rev., 2015, 44(16), 5793–5805 RSC.
- G. V. Lowry, K. B. Gregory, S. C. Apte and J. R. Lead, Transformations of Nanomaterials in the Environment, Environ. Sci. Technol., 2012, 46(13), 6893–6899 CrossRef PubMed.
-
M. P. Monopoli, A. S. Pitek, I. Lynch and K. A. Dawson, Formation and characterization of the nanoparticle–protein corona, Nanomaterial Interfaces in Biology: Methods and Protocols, 2013, pp. 137–155 Search PubMed.
- M. Lundqvist, J. Stigler, G. Elia, I. Lynch, T. Cedervall and K. A. Dawson, Nanoparticle size and surface properties determine the protein corona with possible implications for biological impacts, Proc. Natl. Acad. Sci. U. S. A., 2008, 105(38), 14265–14270 CrossRef PubMed.
- I. Lynch and K. A. Dawson, Protein-nanoparticle interactions, Nano Today, 2008, 3(1), 40–47 CrossRef.
- F. Nasser and I. Lynch, Secreted protein eco-corona mediates uptake and impacts of polystyrene nanoparticles on Daphnia magna, J. Proteomics, 2016, 137, 45–51 CrossRef PubMed.
- A. L. Chen, The dose makes the poison, Nat. Nanotechnol., 2011, 6(6), 329 CrossRef PubMed.
- M. Auffan, J. Rose, J.-Y. Bottero, G. V. Lowry, J.-P. Jolivet and M. R. Wiesner, Towards a definition of inorganic nanoparticles from an environmental, health and safety perspective, Nat. Nanotechnol., 2009, 4(10), 634–641 CrossRef PubMed.
- S. M. Briffa, I. Lynch, V. Trouillet, M. Bruns, D. Hapiuk and J. Liu,
et al., Development of scalable and versatile nanomaterial libraries for nanosafety studies: polyvinylpyrrolidone (PVP) capped metal oxide nanoparticles, RSC Adv., 2017, 7(7), 3894–3906 RSC.
-
I. Lynch, K. Dawson, J. Lead and E. Valsami-Jones, Macromolecular Coronas and their importance in Nanotoxicology and Nanoecotoxicology, Frontiers of Nanoscience, Elsevier, Amsterdam, 2014 Search PubMed.
- E. C. Cho, Q. Zhang and Y. Xia, The effect of sedimentation and diffusion on cellular uptake of gold nanoparticles, Nat. Nanotechnol., 2011, 6(6), 385–391 CrossRef PubMed.
- A. Albanese, C. D. Walkey, J. B. Olsen, H. Guo, A. Emili and W. C. Chan, Secreted biomolecules alter the biological identity and cellular interactions of nanoparticles, ACS Nano, 2014, 8(6), 5515–5526 CrossRef PubMed.
- A. Albanese, P. S. Tang and W. C. Chan, The effect of nanoparticle size, shape, and surface chemistry on biological systems, Annu. Rev. Biomed. Eng., 2012, 14, 1–16 CrossRef PubMed.
- A. Albanese and W. C. Chan, Effect of gold nanoparticle aggregation on cell uptake and toxicity, ACS Nano, 2011, 5(7), 5478–5489 CrossRef PubMed.
- K. A. Dawson, S. Anguissola and I. Lynch, The need for in situ characterisation in nanosafety assessment: funded transnational access via the QNano research infrastructure, Nanotoxicology, 2012, 7(3), 346–349 CrossRef PubMed.
- R. Y. Hong, J. H. Li, L. L. Chen, D. Q. Liu, H. Z. Li and Y. Zheng,
et al., Synthesis, surface modification and photocatalytic property of ZnO nanoparticles, Powder Technol., 2009, 189(3), 426–432 CrossRef.
- C. Cayrol, J. Sarraute, R. Tarroux, D. Redoules, M. Charveron and Y. Gall, A mineral sunscreen affords genomic protection against ultraviolet (UV) B and UVA radiation: in vitro and in situ assays, Br. J. Dermatol., 1999, 141(2), 250–258 CrossRef PubMed.
- L. De Marzi, A. Monaco, J. De Lapuente, D. Ramos, M. Borras and M. Di Gioacchino,
et al. Cytotoxicity and Genotoxicity of Ceria Nanoparticles on Different Cell Lines in Vitro, Int. J. Mol. Sci., 2013, 14(2), 3065–3077 CrossRef PubMed.
-
D. R. Baer, P. Munusamy, A. Karakoti, S. Kuchibhatla, S. Seal and S. Thevuthasan, Ceria Nanoparticles: Planned and Unplanned preparation and Environmental Impacts on Particle Properties, 2012 Search PubMed.
- B. Djuričić and S. Pickering, Nanostructured cerium oxide: preparation and properties of weakly-agglomerated powders, J. Eur. Ceram. Soc., 1999, 19(11), 1925–1934 CrossRef.
- W. Lin, Y.-W. Huang, X.-D. Zhou and Y. Ma, Toxicity of cerium oxide nanoparticles in human lung cancer cells, Int. J. Toxicol., 2006, 25(6), 451–457 CrossRef PubMed.
- E. Matijević and W. P. Hsu, Preparation and properties of monodispersed colloidal particles of lanthanide compounds: I. Gadolinium, europium, terbium, samarium, and cerium (III), J. Colloid Interface Sci., 1987, 118(2), 506–523 CrossRef.
- S. Logothetidis, P. Patsalas and C. Charitidis, Enhanced catalytic activity of nanostructured cerium oxide films, Mater. Sci. Eng., C, 2003, 23(6–8), 803–806 CrossRef.
- L. Yin, Y. Wang, G. Pang, Y. Koltypin and A. Gedanken, Sonochemical Synthesis of Cerium Oxide Nanoparticles—Effect of Additives and Quantum Size Effect, J. Colloid Interface Sci., 2002, 246(1), 78–84 CrossRef PubMed.
-
Influence of aging on the properties of cerium oxide nanoparticles - implications to quantum confinement effect, ed. S. V. N. T. Kuchibhatla, A. S. Karakoti, S. Thevuthasan, S. Seal and D. R. Baer, Nanotechnology (IEEE-NANO), 2011 11th IEEE Conference on, 2011 15–18 Aug. 2011 Search PubMed.
- H.-I. Chen and H.-Y. Chang, Synthesis of nanocrystalline cerium oxide particles by the precipitation method, Ceram. Int., 2005, 31(6), 795–802 CrossRef.
- G. R. Bamwenda and H. Arakawa, Cerium dioxide as a photocatalyst for water decomposition to O2 in the presence of Ceaq 4+ and Feaq 3+ species, J. Mol. Catal. A: Chem., 2000, 161(1–2), 105–113 CrossRef.
- V. Morris, P. G. Fleming, J. D. Holmes and M. A. Morris, Comparison of the preparation of cerium oxide nanocrystallites by forward (base to acid) and reverse (acid to base) precipitation, Chem. Eng. Sci., 2013, 91(0), 102–110 CrossRef.
-
OECD, Test No. 202: Daphnia sp. Acute Immobilisation Test, OECD Publishing, 2004.
-
OECD, Test No. 211: Daphnia magna Reproduction Test, OECD Publishing, 2012.
- S. S. Oosterhuis, M. A. Baars and W. C. K. Breteler, Release of the enzyme chitobiase by the copepod Temora longicornis: characteristics and potential tool for estimating crustacean biomass production in the sea, Mar. Ecol.: Prog. Ser., 2000, 196, 195–206 CrossRef.
- K. N. Baer and C. E. Goulden, Evaluation of a high-hardness COMBO medium and frozen algae for Daphnia magna, Ecotoxicol. Environ. Saf., 1998, 39(3), 201–206 CrossRef PubMed.
- M.-L. Avramescu, P. E. Rasmussen, M. Chénier and H. D. Gardner, Influence of pH, particle size and crystal form on dissolution behaviour of engineered nanomaterials, Environ. Sci. Pollut. Res., 2017, 24(2), 1553–1564 CrossRef PubMed.
-
D. Ebert, Ecology, epidemiology, and evolution of parasitism in Daphnia, 2005 Search PubMed.
- R. C. Santore, R. Mathew, P. R. Paquin and D. DiToro, Application of the biotic ligand model to predicting zinc toxicity to rainbow trout, fathead minnow, and Daphnia magna, Comp. Biochem. Physiol., Part C: Toxicol. Pharmacol., 2002, 133(1), 271–285 Search PubMed.
- M. Heinlaan, A. Ivask, I. Blinova, H.-C. Dubourguier and A. Kahru, Toxicity of nanosized and bulk ZnO, CuO and TiO2 to bacteria Vibrio fischeri and crustaceans Daphnia magna and Thamnocephalus platyurus, Chemosphere, 2008, 71(7), 1308–1316 CrossRef PubMed.
- N. M. Franklin, N. J. Rogers, S. C. Apte, G. E. Batley, G. E. Gadd and P. S. Casey, Comparative Toxicity of Nanoparticulate ZnO, Bulk ZnO, and ZnCl2 to a Freshwater Microalga (Pseudokirchneriella subcapitata): The Importance of Particle Solubility, Environ. Sci. Technol., 2007, 41(24), 8484–8490 CrossRef PubMed.
- J. Mac Mahon and F. Rigler, Feeding rate of Daphnia magna Straus in different foods labeled with radioactive phosphorous, Limnol. Oceanogr., 1965, 10, 105–113 CrossRef.
- A. T. Wray and S. J. Klaine, Modeling the influence of physicochemical properties on gold nanoparticle uptake and elimination by Daphnia magna, Environ. Toxicol. Chem., 2015, 34(4), 860–872 CrossRef PubMed.
- J. Kalman, K. B. Paul, F. R. Khan, V. Stone and T. F. Fernandes, Characterisation of bioaccumulation dynamics of three differently coated silver nanoparticles and aqueous silver in a simple freshwater food chain, Environ. Chem., 2015, 12(6), 662–672 CrossRef.
-
I. Lynch, K. A. Dawson, J. R. Lead and E. Valsami-Jones, in: Chapter 4-Macromolecular Coronas and Their Importance in Nanotoxicology and Nanoecotoxicology, Frontiers of Nanoscience, ed. R. L. Jamie and V.-J. Eugenia, Elsevier, 2014, vol. 7, pp. 127–156 Search PubMed.
-
C. Hoek, D. Mann and H. M. Jahns, Algae: an introduction to phycology, Cambridge University Press, 1995 Search PubMed.
- E. Casals, T. Pfaller, A. Duschl, G. J. Oostingh and V. Puntes, Time evolution of the nanoparticle protein corona, ACS Nano, 2010, 4(7), 3623–3632 CrossRef PubMed.
- D. Dell'Orco, M. Lundqvist, C. Oslakovic, T. Cedervall and S. Linse, Modeling the time evolution of the nanoparticle-protein corona in a body fluid, PLoS One, 2010, 5(6), e10949 CrossRef PubMed.
-
M. Rahman, S. Laurent, N. Tawil, L. Yahia and M. Mahmoudi, Protein-nanoparticle interactions, Springer, 2013 Search PubMed.
- A. Albanese, C. D. Walkey, J. B. Olsen, H. Guo, A. Emili and W. C. Chan, Secreted biomolecules alter the biological identity and cellular interactions of nanoparticles, ACS Nano, 2014, 8(6), 5515–5526 CrossRef PubMed.
Footnotes |
† Electronic supplementary information (ESI) available. See DOI: 10.1039/c8en00063h |
‡ These authors contributed equally to this work. |
|
This journal is © The Royal Society of Chemistry 2018 |
Click here to see how this site uses Cookies. View our privacy policy here.