DOI:
10.1039/C6QM00298F
(Review Article)
Mater. Chem. Front., 2017,
1, 1001-1027
Ordered mesoporous carbon and its applications for electrochemical energy storage and conversion
Received
7th November 2016
, Accepted 4th January 2017
First published on 5th January 2017
Abstract
Ordered mesoporous carbon (OMC) is a flexible material providing interconnected channels for the diffusion of electroactive species in electrochemical systems. This is a unique feature, which distinguishes OMC from other types of carbonaceous materials including mesoporous carbon (MC). The potential of OMC in electrochemical systems has been overshadowed by the vague terminology of this class of mesoporous materials. Despite the ordered structure of mesopores, the electrochemistry of OMC is not straightforward. This manuscript reviews the opportunities experimentally presented for employing OMC in various electrochemical power sources such as ultracapacitors, supercapacitors, battery systems, fuel cells, and electrochemical hydrogen storage systems. The aim is to highlight its potential and critical issues. For instance, the graphitization of OMC is of particular importance, as the ordered alignment of π electrons can be beneficial for charge transfer, while the mesopore edges can resemble the peculiar properties of graphene edges.

Ali Eftekhari
| Ali Eftekhari is a professor of chemistry temporarily in the University of Ulster and Queen's University Belfast. He has worked in different capacities in various universities across 4 continents and founded two internationally recognized academic schools from scratch, which were considered as exceptional success stories by major media such as the British newspaper, The Guardian. His research interest is focused on material design for electrochemical systems and he has worked on energy storage systems for 20 years. He is the principal author of over 100 papers published in leading scholarly journals and has supervised over 110 postdoctoral researchers and graduate students. He is the President of the American NanoSociety. |
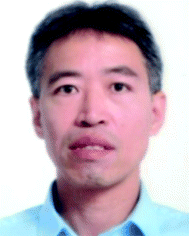
Zhaoyang Fan
| Dr Zhaoyang Fan obtained his bachelor and master degrees from the Tsinghua University of China and PhD from the Northwestern University of US. He is currently an associate professor in the Department of Electrical and Computer Engineering and NanoTech Center, Texas Tech University. His current research concerns synthesis and property studies of nanomaterials and semiconductor thin films, and their application development for photonic devices, photovoltaics, and electrochemical energy storage systems. |
1. Introduction
Owing to its unique flexibility in forming nanostructures, carbon has found a central role in the realm of nanotechnology, with fullerenes, carbon nanotubes, and graphene as the landmark discoveries.1 A new member of this family is ordered mesoporous carbon (OMC), which has recently attracted considerable attention.
On the other hand, porous carbon materials, although not so exciting, have a much longer practical application history. With their remarkable physicochemical properties, including a large specific surface area (SSA) and pore volume, high corrosion resistance, and good thermal and mechanical stabilities, and further considering their easy manufacturing and the abundance of their precursors on Earth, porous carbon materials have been employed in various applications. These include water and air filtration, as catalysts or catalyst supports, as gas storage hosts, and in electrochemical energy storage and conversion systems. They are still dominant characteristic materials for such applications.
According to the IUPAC classification of porosity, micropores are <2 nm, mesopores are in the range of 2–50 nm, and macropores are >50 nm. Traditional porous carbon materials, including activated carbons and carbon molecular sieves, are commonly synthesized using a pyrolysis process with appropriate carbon precursors such as coal, polymers, and carbides, with activation through potassium hydroxide2 or selective etching of metal ions in carbides by halogen gases.3 Associated with these micropore-dominated carbon materials are drawbacks that include slow mass transport in the micropores, low electrical conductivity resulting from abundant surface groups and defects, and porous structure collapse at high temperature, among others.
From the consideration of molecule diffusion and electrolyte ion transportation, mesoporous carbon (MC), which is dominated by 2–50 nm mesopores, is more interesting, since micropores might not be easily accessible by these species, while macropores are simply too large leading to a reduced SSA. Therefore, MC was subsequently developed through a variety of strategies.4–9 This MC material has a broad pore-size distribution without structural ordering (or symmetry). Indeed, MC is a general category according to the physical porosity and does not necessarily represent the same geometrical structure. The mesoporosity of carbon in the form of nanostructured texture obviously increases the specific surface area. Of course, the disordered pores are unpredictable in electrochemical performance, as the pores are not necessarily electrochemically accessible.
OMC certainly can be regarded as a natural evolution from MC when constraints of narrow pore size distribution with ordered pore positions are applied. Strictly speaking, if a perfect ordering is defined for a specific pore size on a macro-scale, such OMC will belong to the category of superlattices or meta-materials with unique properties due to its 2D or 3D periodical structure resulting in quantization of wave vectors (i.e. dramatically different from MC with a random pore size and a random pore position). However, from a practical point of view in electrochemistry, neither a perfect pore ordering nor the same pore size is necessary. OMC, with either a non-perfect ordering or a non-uniform pore size, due to the easier fabrication and control, is under active investigation for much better performance in many applications that traditionally employ MC or porous carbon. This is particularly true for electrochemical energy conversion and storage. The explosive increase in the number of papers published in the very recent few years illustrates the research interest in this field.
In this review, we will first summarize the synthesis methods and novel OMC properties, and then focus on its applications for electrochemical energy conversion and storage, including supercapacitors, lithium-ion batteries (LIBs), and other emerging rechargeable batteries.
2. Ordered mesoporous carbon: synthesis and properties
2.1. From mesoporous carbon to ordered mesoporous carbon
Mesoporous carbons could be synthesized by different methods, such as catalytic activation of carbon precursors using metal-containing species,9,10 carbonization of the blends of one thermosetting precursor and one thermally unstable polymer,7 and carbonization of organic aerogels.11 The produced mesopores from these methods generally have a broad size distribution. They also introduce a lot of micropores. Well-controlled pore size distribution through a template method, therefore, became more interesting.
The story was started by attempts at utilizing silica gel as the template for the preparation of carbonaceous materials. In the 1980s, synthesis of MC was investigated by impregnating a phenol–hexamine mixture as a carbon precursor into silica gel as a template.12 Owing to the spacious structure of silica gel, the carbon precursor can be easily inserted. Upon the completion of the carbonization process, the silica template can then be easily removed. Although the resulting MC has no structural ordering (symmetry), this effort provided the strategy of template-guided OMC synthesis, as long as an ordered template with suitable dimensions is available. Liquid crystals provide an ordered structure with the mobility of a liquid, which can be used as a mesoporous template. This feature was utilized in 1992 to fabricate mesoporous molecular sieves13,14 and led to the birth of an interesting family of ordered mesoporous materials with crystal-like symmetries. Carbon is usually a pioneer in forming new nanostructures, but cannot be synthesized chemically in a liquid through the liquid crystal template. Hence, OMC is synthesized by utilizing the mesoporous silica templates originally prepared using the liquid crystal template.15–17 For about two decades starting in the 1980s,12,18,19 various silica and zeolite templates were utilized for the preparation of MC. The birth of OMC did not happen until 1999 when cubic mesoporous aluminosilicate MCM-48 was employed as the hard template,15,20 thus creating a new nanocarbon structure with symmetry. Currently, OMC that has a very narrow pore-size distribution with periodical structural ordering is synthesized using a template. Depending on the template used, the OMC synthesis can be differentiated into two categories: hard-templating based (impregnation and etching back) and soft-templating based (direct synthesis). Their major differences are illustrated in Fig. 1.
 |
| Fig. 1 Schematics comparing (a) hard-template based and (b) soft-template based synthesis strategies. Reproduced with permission from ref. 19. Copyright 2006, Wiley-VCH. | |
2.2. OMC synthesis: hard-template based
In this strategy, an ordered mesoporous solid template is applied as the mold for the negative replication of OMCs. The pore size control and symmetric ordering are simply determined using the solid template and do not rely on the interaction between the carbon precursor and the template.
The development of hard-template based OMCs was comprehensively reviewed by Dai et al.4 In this hard-templating synthesis strategy,19 a preformed hard template, particularly a silica based ordered mesoporous structure, is used, and carbon precursors are impregnated into the pore volume. After carbonization, the hard template is etched away, leaving the negatively replicated OMC. That is, the interconnected pore space in the template is transferred into the continuous framework of the OMC, while the volume once occupied by the template material becomes mesopores in the OMCs. The 3D interconnected porous structure of the template is generally considered to be necessary for this process. The success of using an SBA-15 silica mesoporous molecular sieve as a template, which has a cylindrical pore structure, is due to the existence of interconnecting micropores in the sidewalls. It is worth mentioning that, in addition to the solution-based impregnation to fill the precursor into the pore volume of the template, chemical vapor deposition was also capable of filling the pore volume.
Template candidates.
Silica-based order mesoporous structures such as MCM, SBA, FDU, MSU-H, and HMS series have contributed to the synthesis of a variety of OMC structures such as cubic structures with several symmetries, face-centered cubic, body-centered cubic, and 2D hexagonal structures. Some efforts have also been focussed on the use of as-synthesized ordered structures of colloid nanoparticles (NPs) as the hard templates. The OMC pore size now is primarily determined by the NP size, and therefore, the pore size can be easily adjusted in a large range.21 Silica NPs, polymer beads such as polystyrene spheres and others can be used. Iron oxide NPs are interesting22 since iron can catalytically induce graphitization at lower temperatures (<1000 °C).23
The key advantage of silica-based templates is the highly ordered architecture of the mesopores, but a key disadvantage is the essential requirement of acid treatment for the template removal. The strong acid treatment does affect the carbon surface and its functionality. This is similar to the undesirable acid treatment required for the removal of the catalyst and catalyst support after chemical vapor deposition of carbon nanomaterials.24 Although the defects formed might be useful in the electrochemical system, the material ordered architecture is lost at the atomic scale. Other templates such as MgO have also been introduced,25–27 which can be removed using a light acid treatment, but the uniformity of the mesopores is not at the level of silica-based templates. However, another considerable advantage of such templates is a practical feasibility for scaling up the material production.
Carbon precursors.
During the high-temperature carbonization and graphitization process, the molecular structure of the precursor will determine the shrinkage of the structural frame and the possibility of micropore generation in the frame, and therefore, the morphology and the SSA of the OMC are formed. Commonly applied precursors can be categorized into those with loose molecular structures such as sugar, sucrose and furfuryl alcohol, and those with dense aromatic structures such as pitch, pyrene, polyacrylonitrile, and acenaphthene. Using the latter category of precursors, the OMC could replicate the reverse image of the hard templates with minimal framework shrinkage, high mechanical strength, and negligible microporosity. In contrast, if precursors with loose structures are applied, framework shrinkage will increase the mesopore size, and particularly micropores will be generated in the framework, leading to bimodal pore-size distribution and a larger SSA. When an SBA-15 silica template is used, micropores in the OMC can also be produced due to the micropores in the pore walls of the template.28,29
Although silica is an ideal template for the preparation of OMC, template removal is time-consuming and somehow destructive to achieve the complete removal of silica. Other mesoporous templates can have some advantages. For instance, electroactive materials are excellent templates if the target application of OMC is in electrochemical systems. For instance, mesoporous nickel oxide, which is an electroactive material for supercapacitors, has been successfully utilized to prepare OMC.30
With the sacrifice of the template used, this hard-template based strategy has its intrinsic limitation on cost. However, it does have several prominent advantages. The impregnation mechanism offers a relatively facile process to precisely replicate the negative image of the hard template, and the nature of the hard template ensures the pyrolysis process causing less damage to the structure regularity and ordering. Another prominent merit of this method is easier graphitization of the OMC formed within the hard template.
2.3. OMC synthesis: soft-template based
Instead of sacrificing the template by etching it away, it is possible to directly synthesize OMC by self-assembling a block copolymer surfactant and a carbon precursor.4,31–35 The advantage of this method is the absence of the template removal step, which is somewhat destructive. An OMC with mesopores in the range of 3–7 nm can be easily synthesized by this method. The interaction between the carbon precursor and the surfactant, which drives the self-assembly of the soft template, determines the pore structure. This strategy is rooted in the pioneering work on the M41S family silica materials.13 The association of surfactant micelles with the precursor through a weak interaction, such as hydrogen bonds, leads to the polycondensation of the precursor. The self-assembly of the composite micelles yields an ordered mesostructured carbon. The removal of the soft template and further carbonization of the remaining precursor polymer yields the OMC with a defined symmetry and pore size.
Since the early report of the soft-templated OMCs by a stepwise assembly approach,31 great progress has been made in synthesizing OMCs using this method, as has been comprehensively reviewed by Yuan's group.36 A variety of OMCs were synthesized in the forms of spheres,37 rhomb-dodecahedra,38 wires39 and ribbons,40 films,31 or monoliths.41 The strategy, through the supramolecular assembly of the soft surfactant templates, can be accomplished by an evaporation-induced self-assembly (EISA) or hydrothermal process. The EISA of a block copolymer and resols is a facile approach with flexibility since it can separate the cross-linking and thermo-polymerization process of the resols from the self-assembling process. The ordered mesostructure is formed on the solvent evaporating surface, and therefore, the OMC films could be produced. Different OMCs with 2D hexagonal, lamellar, 3D bicontinuous, body-centered cubic, and other structures have been prepared using this route.42 Using water to replace the organic solvents is an alternation,43 in which the surfactant-driven self-assembly and carbon precursor polymerization occur in a cooperative process. Such an aqueous route offers better reproducibility and is suitable for large scale production. The hydrothermal process was also reported to fabricate OMCs.37,41
Direct synthesis with surfactant recycling provides the opportunity for large-scale production. The challenge of this strategy is that currently available options of the soft templates are mainly limited to block copolymers and the precursor to phenolic resin. The block copolymer limits the minimum pore size to ca. 3 nm. Smaller amphiphilic molecules as templates should be explored for smaller pore sizes.
Upon comparing the OMCs synthesized from these two strategies, it is found that the soft-template OMCs have thick pore walls with a continuous framework, and thus, offer stability in harsh thermal and oxidation treatments for functionalization. However, the hard-template OMCs are much easier to be graphitized, while it is difficult for the soft-template OMCs.
The morphology of the OMCs (including specific surface area, pore size, and volume) can be altered by post-synthesis modification. KOH activation is a common method industrially employed for generating the micropores in activated carbon.2,44–46 Depending on the activation conditions (temperature, concentration, etc.), the micropores generated can merge into each other to form mesopores or create a microporosity texture. In the latter case, micropores can build shortcut bridges between the mesopores, which can be beneficial for electrochemical performance.47 This method of activation can double the specific surface area of OMC.
2.4. Graphitization of OMC
It is sometimes wrongfully suggested that OMC is not a graphitic material. Considering the fact that amorphous carbon materials are actually a form of disordered graphite, these types of carbonaceous materials are always graphitic with different degrees of graphitization, which is usually judged by the D and G bands in Raman spectra. From a practical perspective, the OMCs with a higher graphitization degree are better for various applications. This is particularly true for electrocatalyst and electrode applications in which higher electrical conductivity critically depends on the degree of graphitization.48 Thus, there is demand for increasing the degree of graphitization of OMCs.
Low-temperature carbonization offers a well-ordered structure, but with an amorphous or low degree of graphitization framework. Post-synthesis thermal treatment,49 particularly if conducted at a high temperature (>2000 °C), will induce significant graphitization, but result in a lower degree of ordering with broad pore-size distribution. Carbon precursors with rich fused aromatic structures such as polyaromatic hydrocarbons or aromatic molecules23,50–55 are better than those with loose molecular structures since the former can be graphitized at much lower temperatures, particularly when an iron-based catalyst is incorporated.28,56 CVD growth at a temperature higher than 900 °C is another option,57 leading to the formation of graphitic OMCs.57–60 In almost all methods, the reaction or treatment is conducted at a high temperature where carbon atoms have enough energy to arrange or re-arrange themselves into the graphitic hexagonal form.61 The temperature could be lowered in the presence of catalytic species, particularly iron-based nanomaterials.
A simple and effective approach is the carbonization of metal phthalocyanines within an SBA-15 template.60 However, this method is not practical because of the high cost of phthalocyanines. It has been reported that phthalocyanine can be replaced by natural fat as the carbon feedstock in the same template system to produce the OMCs with graphitic walls at relatively low temperature.62 A new approach is to utilize a triblock-copolymer template, which results in the formation of highly graphitic OMCs while the mesopore size is strongly tunable in the range of ca. 3–40 nm.31,32,63,64
Graphitization of OMC is of particular importance in electrochemical systems, as it increases the electrical conductivity, which is indeed a major requirement to reduce the charge transfer resistance. In the absence of appropriate graphitic conductivity, the electrical conductivity of OMC should be improved by some conductive agents such as graphene or metals to achieve the required conductivity for electrochemical performance.65–74
2.5. OMC with graphene walls
Graphene foam has been developed in recent studies of graphene-based electrode applications. Chemically-derived individual reduced graphene oxide sheets, with a sub-micrometer size, can be assembled into a foam structure through freeze-drying or similar methods.75–77 Such a graphene foam has irregular pore distribution, and particularly, it does not form an intimately connected network. Direct growth of multilayered graphene (MLG) inside of a 3D metal frame resulted in macro-pores of a few hundred micrometers in diameter by the metal foam template.78 Both are not the optimized electrode structures when compared to the graphitized OMC structure with graphene walls.
The OMC with graphene walls is a variation of graphitized OMC when the highly graphitized pore wall is thinned down to a few atomic layers. The soft-template based method will not provide a high degree of graphitization and is therefore unsuitable for producing graphitized OMC; while for the hard templates, in order to obtain pore walls of graphitized OMC down to several atomic layers, the void space in the hard templates should be thin enough. Furthermore, the high degree of pore ordering and graphitization demands a catalytic graphitization process at a moderate temperature.
Jang et al.79 reported the growth of mesoporous graphene balls (MGBs) using drop-cast polystyrene (PS) beads as a template. As illustrated in Fig. 2a, carboxylated PS was synthesized via emulsion polymerization and then sulfurized to obtain SPS-COOH, which can strongly absorb iron ions (Fe3+) when immersed in FeCl3 solution. Using the drop-cast beads as templates and precursors, the sample was annealed in an H2/Ar environment to catalytically promote graphene ball growth. Thus, the resulting graphene balls have a diameter similar to the functionalized PS beads, but etching away the Fe from the sphere walls will form MGBs, as shown by the transmission electron microscopy (TEM) images in Fig. 2b and c. Due to the irregular distribution of the PS beads in the template, the synthesized MGBs did not form an ordered 3D macrostructure.
 |
| Fig. 2 (a) Schematic of mesoporous graphene nanoball synthesis from drop-casting a functionalized bead/FeCl3 solution, to thermal annealing for graphene growth, and etching away the Fe domain. (b and c) TEM images of the mesoporous graphene nanoballs. Reproduced with permission from ref. 79. Copyright 2013, American Chemical Society. | |
Dong et al.22,80 took one step further to synthesize graphitized OMCs with superlattice symmetry. In their work, the Fe3O4 colloidal NPs formed a superlattice structure, which was employed as both the template and the catalyst. Using oleic acid (OA) capped Fe3O4 colloidal nanocrystals (NC), a 3D superlattice was self-assembled followed by the carbonization/graphitization of the OA ligands at 500 °C in the presence of an iron catalyst. The partially graphitized OMC after the NC removal is shown in Fig. 3a and b. Further heat treatment at 1000 °C results in the formation of multilayered graphene as a pore wall (Fig. 3c and d). The resulting graphitized OMC exhibits a long-range highly-ordered superlattice symmetry, replicated from the template.
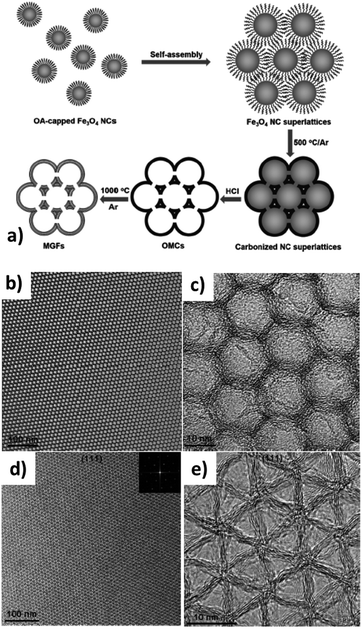 |
| Fig. 3 (a) Schematic of OMG synthesis from colloidal Fe3O4 NPs. (b and c) TEM images of the as-synthesized OMC superlattice after catalytic carbonization at 500 °C with fcc symmetry. (d and e) TEM images of OMG after being further graphitized at 1000 °C, imaged for the (111) plane. Reproduced with permission from ref. 22. Copyright 2015, Wiley-VCH. | |
Huang's group81 employed SBA-15 as a template and poly-furfuryl alcohol (PFA) as a precursor to synthesize a graphitized OMC. Fig. 4 demonstrates the synthesis procedure. First, an impregnated template is formed by depositing the Ni agent within the silica template, and then the N-doped ordered mesoporous with walls made of few-layer carbon are built within the template using a CV process. The final step is the removal of the template and the metallic catalyst.
 |
| Fig. 4 (a) Schematic of synthesizing nitrogen doped OMG. (b) Low-angle X-ray diffraction patterns of the synthesized sample with or without N-doping, showing the characteristic peaks of hexagonal packing. Reproduced with permission from ref. 81. Copyright 2015, American Association for the Advancement of Science. | |
2.6. Graphene edge-oriented pore walls
For these highly graphitized OMCs, the graphite basal plane is generally exposed as the pore wall surface. In electrochemical systems, the graphite basal plane is far less attractive than the edges. Ideal graphene is not an interesting material in terms of electrochemical reactions due to the high ratio of the basal plane to edges, and particularly the flatness of the hexagonal network of carbon atoms in which the sp2 hybrid is not very reactive.82 The latter is somehow shielded by the π electrons avoiding direct adsorption at carbon atoms. It is known that the edge plane of highly oriented pyrolytic graphite has an electrochemical reactivity several orders of magnitude higher than its basal plane due to a large amount of defects, such as kinks, steps, and vacancies, at the edge that produce a high density of defect states near the Fermi level.83 These atomic edges or steps are the potential adsorption sites of redox species in the electrolyte and sites of electron transfer. The edge planes can also provide an electrical double layer capacitance of 50 to 70 mF cm−2 in comparison with that of basal planes, which provide a capacitance of only ∼3 mF cm−2.84 The edge-oriented multilayered graphene flakes have been investigated for a variety of electrochemical applications.85–88 Therefore, it is highly desirable to synthesize OMCs with exposed graphene edges.
Using Al-incorporated silica templates and various aromatic compounds as precursors in an autoclave process, followed by further graphitization at high temperatures, Ryoo's group56 demonstrated a unique CMK-3 OMC with graphitic framework structures through in situ conversion of the aromatic compounds into mesophase pitch inside the silica templates. The degree of graphitization of OMC can be at the level of multi-walled carbon nanotubes or multiple-layer graphene. A striking feature is that the hexagonal arrays of carbon nanorods are formed by stacking of the discoid graphene sheets oriented perpendicular to the rod orientation, with the exposed edge-on graphitic structure, as shown in Fig. 5.
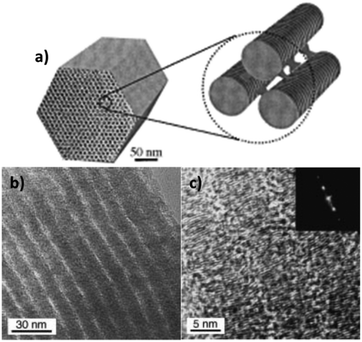 |
| Fig. 5 (a) Schematic of CMK-3G OMG consisting of hexagonal arrays of carbon nanorods, while the nanorods are formed by stacking of the discoid graphene sheets oriented perpendicular to the rod orientation. (b) TEM images of CMK-3G showing the rod array. (c) TEM images showing the stacked discoid graphene sheets. The inset shows the corresponding electron diffraction pattern. Reproduced with permission from ref. 84. Copyright 1971, The Electrochemical Society. | |
Utilizing nickel foam89 or cellulose paper90 as a template, Ren et al. deposited an edge-oriented multiple-layer graphene porous structure using a plasma CVD process and the template can be removed.91 Since the template used has large pores without ordering, the edge-oriented graphene porous structure thus produced does not belong to the category of graphitized OMC. However, if suitable templates are used, such a plasma CVD process may provide a viable approach for producing edge-oriented graphitized OMC.
In general, there are various methods to enhance the degree of graphitization of the OMCs to align the π electrons along the ordered architecture, and it is still an active area of research. However, our knowledge about the arrangement of graphene hexagons across the mesopores is very limited. In the graphene structure, edge atoms have anomalous electronic properties resulting in different electrochemical performance. However, the actual atomic structure of the edges of OMC mesopores is not fully understood.
3. OMC in electrochemical systems
3.1. Electrochemistry of OMC
A comparative study revealed that the electrochemical activity of the OMC is higher than that of graphene, which is a popular material in the realm of electrochemistry.92 In a sense, OMC is considered as an alternative to the classical microporous carbon materials which have been commercially available for decades. Therefore, the goal of OMC production is to achieve the highest possible specific surface area, but this is not the main requirement in the electrochemical systems. Microporous carbonaceous materials have an extremely high specific surface area, but micropores are too small for the diffusion of electroactive species. Since mesopores fit well with the size of diffusing species, OMCs have attracted considerable attention in the realm of electrochemistry. However, the common standpoint was to employ the OMC as a high surface area carbon. This is the reason that the majority of electrochemical studies of OMCs are focused on supercapacitors93–105 or sensors.106
The characteristic feature of the OMC in electrochemical systems is the possibility of fast diffusion through the solid electroactive material. Unfortunately, our understanding of the electrochemistry of OMCs is very limited, as fewer efforts have been made for this important issue. There is a similar situation for the electrochemistry of graphene because graphene is not as simple as the flat hexagonal network of carbon atoms imagined.107–109 Instead, graphene is full of chemical and physical irregularities which can substantially change the electrochemical process. This misleading comes from the straightforward structure of ideal graphene, but OMC chemical irregularities are too complicated even for an ideal structure.
The arrangement of carbon atoms at the mesopore edges is indeed a mystery, particularly as the graphitization level of OMC is not absolute or uniform. Similar to graphene, the electrochemistry at the mesopore edges is completely different from that on the internal walls of the mesopores. A key difference is that edges and dangling atoms in graphene are chemically preferred sites; but in OMC, the mesopore edges are even physically preferred to initiate the electrochemical reactions, as they are more accessible.
Since carbonaceous nanomaterials are subject to chemical treatment resulting in severe structural changes, their electrochemistry cannot be easily judged. For instance, it was claimed that the majority of the electrochemical activity of graphene samples is due to the defects formed during the post-treatment rather than the original graphene structure.110,111 This phenomenon is more complicated for OMC, as the template removal is at the atomic scale. In other words, not only the chemical treatment but also the carbon template interactions can result in peculiar defects.
Electrochemistry within the OMC matrix is probably different from that on other carbonaceous nanomaterials and far from fully understood. For instance, double layer capacitance is a straightforward phenomenon with least electrochemical complexity, but the specific capacitance of OMC within its silica template is similar to that after template removal.112
3.2. Micropores within macroporous structures
The internal accessibility of mesopores is not the only factor in electrochemical performance, as electroactive species should reach the mesopores regularly. Since the rate-determining step in most electrochemical systems is the diffusion process, it is vitally important to facilitate fast diffusion of electroactive species to keep them ready for insertion into the mesopores. Note that the charge transfer resistance for a conductive carbon with a high specific surface area is not normally the rate-determining step. This macrostructure of mesoporous carbon is of particular importance for electrochemical systems. Ordered hierarchical mesoporous/macroporous carbon displays good performance in electrochemical systems, as the ordered macropores facilitate fast diffusion through the electrolyte to mesopores.113Fig. 6 shows the ordered structure at two different scales of such carbonaceous materials.
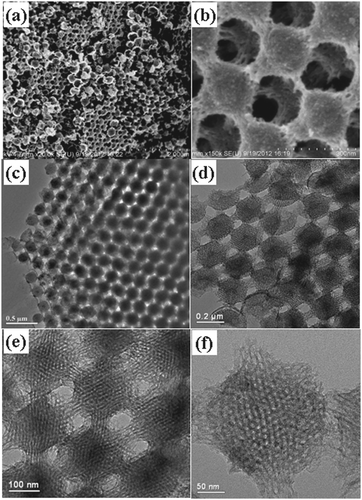 |
| Fig. 6 SEM and TEM images of an ordered hierarchical mesoporous/macroporous carbon at different scales. Reproduced with permission from ref. 113. Copyright 2013, Wiley-VCH. | |
Fig. 7 displays how a macroporous structure can be created using mesoporous building blocks. Such two-level porous carbon has been prepared using different methods.114 A simple idea is to use two different templates for the formation of macropores and mesopores simultaneously. For instance, polystyrene is known as a good template for the formation of macropores; introducing silica nanoparticles creates mesopores throughout the walls of the macroporous carbon synthesized.115
 |
| Fig. 7 An illustration for designing carbon-supported Pt electrocatalysts with macroporous/mesoporous architecture. Reproduced with permission from ref. 114. Copyright 2015, Wiley-VCH. | |
3.3. OMC nanocomposites
As described above, OMC is prepared to utilize mesoporous templates, which should be removed. Depending on the properties of the template, the final material can be OMC/silica, which is indeed a nanocomposite with potential applications. In a similar fashion, OMC can be used as the template for the synthesis of a mesoporous electroactive material; then, it is not necessary to remove the template, as the OMC template is indeed an important component of the targeted nanocomposite. This leads to the formation of a uniformly distributed nanocomposite, which is indeed a key goal for electroactive nanocomposites in which carbon should contribute to the electrical conductivity and fairly separating the electroactive materials to become more electrochemically accessible. OMC has been widely used as a nanocomposite component for various electroactive materials.116
Since the size of the mesopores is too small for conducting uniform reactions therein, it is a serious challenge to uniformly fill the mesopores with the second component. For instance, sonochemical synthesis can result in a more uniform distribution of reactants to form the electroactive material uniformly distributed over the OMC pores.116
A common issue for electroactive materials is the necessity of binders to form a solid electrode. Binders are usually electrochemically inactive and may block the diffusion of electroactive species; besides the fact that their mass reduces the specific energy and power of electrochemical power sources. In addition to the possible chemical interaction of OMC with electroactive materials, the interconnected architecture of OMC provides a rare opportunity for in situ preparation of nanocomposites in which the electroactive material is entangled through the OMC scaffold.117,118
While OMC mesopores can serve as local tubes for the formation of an electroactive material, micropores are too small and will be blocked by the resulting material for electrochemical performance.119 This blockage is not necessarily physical filling, as mesopores are also filled with the electroactive material, but its lattice network is still large enough to allow solid-state diffusion. This feature is not limited to OMC, and 3D MCs with larger mesopores are indeed good matrices for the formation of electroactive materials.120
4. OMC in electrochemical energy storage
4.1. OMC for ultracapacitors (double layer capacitors)
High surface area carbon has always been the first choice for double layer capacitors (aka ultracapacitors). It is not difficult to prepare a carbon with an extremely high surface area, but not all the surface area is electrochemically accessible. Thus, most carbon-based ultracapacitors have specific capacitance below their theoretical capacities. OMC can provide an ordered matrix for the interfacial interaction with charged ions within the electrolyte solution. However, the limitation is the size of the mesopores. If they are too small, ions cannot freely diffuse therein, and if too large, the specific surface area is low. In fact, the mesopores should be tuned for a specific ultracapacitor depending on the size, charge, and structure of the electroactive species forming the double layer.121 Gogotsi and Simon have elaborated the dependency of the specific capacitance of an OMC on the pore size.122 In general, an OMC with sufficiently large pores has a specific surface area around 1000 m2 g−1, resulting in specific capacitance comparable with other types of carbon materials.65,123,124
However, the capacitive behavior of OMC is not straightforward as the nature of the double layer within the mesopores is still ambiguous. Different values have been reported for the specific capacitance of similar OMCs. It has been explained that the synthesis conditions and post-treatment have huge impacts on the electrochemical performance of OMC.125
Highly ordered single crystals of mesoporous carbon show excellent electrochemical performance for ultracapacitors.126 The large crystalline particles dictate that the electrochemical process is conducted through the particle matrix rather than the external interface (Fig. 8). Although it is not entirely a solid-state system because electrolytes are soaked within the mesopores, the electrochemical performance relies on diffusion through interconnected pores. This clearly indicates that internal mesopores are responsible for the high specific capacitance achieved (281 F g−1 at 0.5 A g−1 in a 6 M KOH electrolyte) rather than having a high surface area because of smaller particles. The importance of this feature is because of the essential requirement of the electroactive packing within the electrode structure. It seems that larger particles, which are of practical interest, with mesoporosity, can provide a large electrochemically accessible area. However, it should be taken into account that the cyclic voltammetric behavior indicates that the system is not purely capacitive. In addition to the complicated pseudocapacitive contribution, the solid-state diffusion is a rate-determining step when the electrochemically accessible area is mostly spread through the internal mesoporosity.
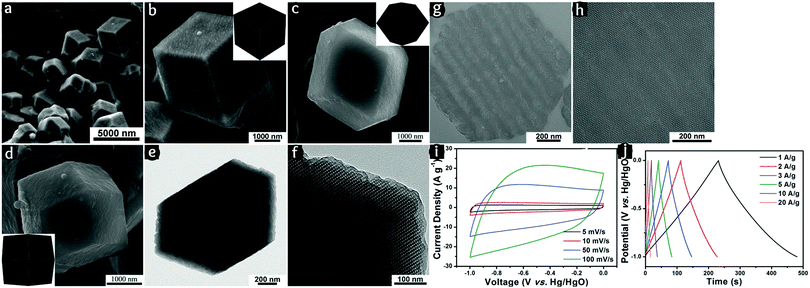 |
| Fig. 8 (a–h) SEM and TEM images of an N-doped OMC with Im3m symmetry and rhombic dodecahedral single crystal morphology. (i) Cyclic voltammograms and (j) charge/discharge profiles of the N-doped OMC as an ultracapacitor. Reproduced with permission from ref. 126. Copyright 2015, Royal Society of Chemistry. | |
The important feature is that the well-ordered particle morphology does not guarantee a smooth electrochemical performance. Two different factors are responsible for this deviation from an ideal behavior. (i) The diffusion of electroactive species within the mesopores, which is dependent on the uniform distribution of the electrolytes within the mesopores and how the mesopores are interconnected. (ii) The macro-structure of the electrode in which the individual particles are packed together. Although the inner structure of the OMC might be well-oriented, there is still no guarantee that the mesopores are electrochemically accessible. In the material characterization, the mesopores might seem open in TEM images, but when packing the particles, some mesopores are blocked by the neighboring particles. Therefore, the ordered structure whether at the mesoscale or larger scales cannot be the sole basis for the material performance, instead, the electrochemical accessibility should be inspected at different scales.
The best capacitive behavior of OMC is obtained when the degree of graphitization is maximum.127,128 Since the graphene basal plane is not electrochemically active,110,111 the formation of graphitic walls along the 001 direction assists charge transfer between the electrode/electrolyte interface and the current collector. Furthermore, the shape and the structure of the double layer over the basal plane of graphene are different from those on amorphous walls of mesopores.
The unique feature of OMC is the translational symmetry of its unit cell, but the internal structure of the unit cell of mesopores can have a rough texture. Although micropores are too small for long diffusion of electroactive species, the presence of microporous texture on the walls of mesopores can be beneficial for ultracapacitor performance as far as they are accessible.129,130
Highly ordered nanofibers of OMC provide an architecture for more effective diffusion of the electroactive species across the electrode matrix. OMC nanofibers display a well-defined capacitive behavior.131 On the other hand, as the nanofibers can be prepared using an AAO hard template, it is possible to directly grow them on the current collector, which is in favor of the overall electrical conductivity and suitable for high-power micro-supercapacitors.132 The hollow structure can provide a better accessibility of the mesopores from both sides, and the specific capacitance of a fibrous-structured hollow OMC has been reported to be very high (359 F g−1), with an extremely large power density (10 kW kg−1) in a 6 M KOH electrolyte.133
In a similar fashion, OMC can be directly synthesized on a polymer substrate to form a flexible film.134 It is a general demand to make the electrochemical cells flexible for wearable electronics, which is realistic by the development of gel electrolytes; however, electrode materials are usually fragile, and the main obstacle still exists in this direction.
The superiority of OMC for supercapacitors is not limited to its high surface area. It is well known that a purely double layer capacitor based on the carbonaceous material is not realistic, as there are always some faradaic redox reactions. This is primarily because of the functional groups formed or adsorbed on the active surface of the carbon. This behavior is more studied for the functional groups on graphene, particularly chemically-derived reduced graphene oxide,135–137 but similar mechanisms are also applied to surface-modified OMC. The presence of various functional groups can significantly alter the electrochemical performance of OMC.138 Chemical treatment or activation of OMC results in the formation of such functional groups, which are responsible for part of the superior ultracapacitor performance.139
Similarly, graphene edges play particular roles in electrochemical systems, as the charge transfer mechanism and barrier can be utterly changed through the dangling bonds at kinks, steps, and vacancies at the edge that produce a high density of defect states near the Fermi level.140 Therefore, the graphite edge plane offers a capacitance 20 times larger than the basal plane. This feature can also be the case for OMC pores having sharp edges. Nevertheless, the structural arrangement of carbon atoms in OMC has not been fully understood yet. The peculiar charge transfer can be controlled by the morphological structure or degree of graphitization of OMC.
Similar to the role of functional groups, doping alters the uniformity of charge distribution across carbon atoms, and chemical reactivity to have more contributions of pseudocapacitive nature. Nitrogen is the most common element for the doping of various types of carbon materials, which also enhances their electrical conductivity. It has also been widely used for the doping of OMC.141–150 Owing to the pseudocapacitive contribution, the specific capacitance of N-doped OMC is over 300 F g−1 in a 1 M H2SO4 electrolyte.151
Another important feature of doping is to enhance the wettability of OMC, which is a serious requirement for ultracapacitors, and also other electrochemical applications. Pristine OMCs normally have a hydrophobic nature, and thus, the electrode/electrolyte interface is poorly formed. N-doping can scientifically contribute to the surface polarity of OMC, but it is not easy to dope a high content of nitrogen. The presence of a Pluronic surfactant having a high content of oxygen in the OMC synthesis interacts with an N-rich carbon precursor to decompose it. This results in a deformed mesostructure, and thus, N-doping sacrifices the uniformity and order of OMC.152–154 On the other hand, simply increasing the amount of nitrogen dopant is not beneficial for the ultracapacitor performance. Although nitrogen doping generally increases the electrical conductivity of carbon because of a higher charge/electron carrier mobility,155,156 too much N can increase the interfacial resistance too.157 Moreover, the pseudocapacitive process contributed by the presence of N can block the original double layer capacitance (because the electrode surface does not have a uniform charge distribution for the formation of the classic Helmholtz layer), resulting in a smaller overall specific capacitance. A comparative study showed that the optimum amount of N doping for an OMC ultracapacitor is about 6%.157
In any case, N-doping can significantly improve the specific capacitance of OMC-based ultracapacitors.155,158–164 The surface of the mesoporous silica template plays a substantial role in the chemical structure of OMC, and can result in the formation of an N-rich surface having a significant redox system. This significantly improves the electrochemical performance.158 The presence of N adatoms and surface groups paves the way for new faradaic reactions, which can contribute to the pseudocapacitive performance, e.g. by facilitating H insertion.165 The specific capacitance of an OMC-based ultracapacitor can be directly increased by increasing the presence of nitrogen while the presence of oxygen functional groups has a slight influence.166
Although the role of the N dopant is to cause chemical inhomogeneity, its influence depends on the OMC architecture too. This effect is much higher for a 3D architecture of OMC (e.g. FDU-16), as the specific capacitance is increased 7 times by N-doping.167 While the original FDU-16 OMC is not considered as a promising material for ultracapacitors, N-doped FDU-16 OMC is among the best candidates. The cyclability is impressive, as the specific capacitance actually increases during the first 10
000 cycles due to the structural rearrangement opening new channels for diffusion.167
Another common dopant of carbon is boron.168 Doping of OMC with boron also increases the specific capacitance.169 When substituting carbon, boron atoms are natively bonded with oxygen atoms. The change in charge distribution across the carbon network and the oxygen functional groups directly bonded with the OMC lattice causes a significant contribution of pseudocapacitance in addition to the original double layer charging.170 Nevertheless, this chemical reactivity results in poor cyclability due to structural changes in the course of faradaic reactions.170
While neighbor elements (B and N) just alter the uniformity of charge distribution across the carbon network, doping with larger elements such as P can alter the morphology too resulting in substantial changes in the surface area and pore volume.171
While hydrophilicity can improve the electrode wettability in electrochemical systems, hydrophobicity can reduce corrosion. Doping of OMC with P increases the hydrophobicity, and thus, protects against corrosion.172 Sulfur functional groups can also contribute to the pseudocapacitive behavior of OMC.173 This also emphasizes that the influence of doping is not only due to the presence of the doping element in the carbon network (changing the charge distribution) but also the functional groups formed on the dopant.
In fact, while the uniform architecture of OMC is beneficial for electrochemical performance, chemical uniformity is not desirable, because chemical inhomogeneities of atoms at the electrolyte/electrode interface facilitate charge transfer and electrochemical reaction. While gaining the ordered structure of OMC, doping can create chemical inhomogeneity. Increasing this inhomogeneity with multiple dopants can significantly increase the specific capacitance, e.g. N/P,171,174,175 B/P,176 and N/S.177–180
Although the large surface area provides a significant double layer capacitance, the faradaic pseudocapacitance (or more precisely a pseudocapacitive mechanism) of N-doped OMC is becoming dominant at high dopant concentration.181 Therefore, the impedance spectroscopic spectra of N-doped OMC represent the characteristics of faradaic systems with a charge transfer semi-circle followed by Warburg impedance.
An important issue, which is usually neglected, is the electrolyte solution. It is evident that the capacitive behavior is affected by the electroactive species due to their charge, but the influence of size is of critical importance for OMC, as the sizes of hydrated/solvated ions are comparable with those of mesopores. This means that some ions are larger or smaller than OMC mesopores, leading to different capacitive behaviors.182,183
It is worth emphasizing that a nitrogen-doped nano-tubular ordered mesoporous few-layer carbon (OMFLC) structure81 has a large surface area (1580 m2 g−1), a large total pore volume (2.20 cm3 g−1), and an average pore width (2.25 nm). After composition optimization, a specific capacitance as high as 855 F g−1 at a current density of 1 A g−1 was obtained in an aqueous solution of H2SO4 (Fig. 9a and b). The Ragone plot of energy vs. power densities of symmetric cells based on this material in two different electrolytes (H2SO4 and Li2SO4) shows extraordinary performance compared to other conventional batteries and supercapacitors (Fig. 9c and d).
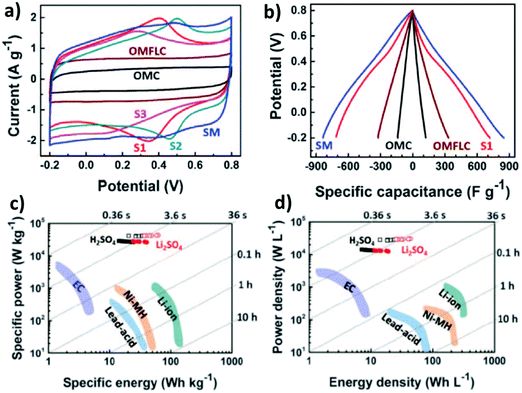 |
| Fig. 9 (a) Cyclic voltammetry test and galvanostatic test of the cells using different components. Ragone plot of specific energy vs. specific power in (c) and energy density vs. power density in (d) of the cell performance, in comparison with other conventional batteries and supercapacitors. Reproduced with permission from ref. 81. Copyright 2015, American Association for the Advancement of Science. | |
4.2. OMC for pseudocapacitors
In pseudocapacitors, the so-called pseudocapacitance is the result of facile faradaic redox reactions at the surface or sub-surface of the electroactive material. Better rate capability and pseudocapacitive behavior are obtained when the ratio of surface reaction is significant in comparison with that of the solid-state reaction. This dominance can be formed by increasing the specific surface area; thus, a significant fraction of the faradaic reaction occurs at the surface with no need for slow solid-state diffusion to reach the reactive redox sites, with the latter being characteristic of charge storage in a battery.
Transition metal oxides are the common candidates for pseudocapacitors. Due to their electronic insulating nature, carbon nanostructures are commonly used as a conductive framework for these electroactive oxides. Increasing the specific surface area is indeed a common strategy in developing electroactive materials for supercapacitors. High surface area nanocomposites with carbon are an appropriate design, as nanostructured carbon can assist in distributing the electroactive material while contributing to the electrical conductivity. In addition to common advantages of carbonaceous nanomaterials, OMC can serve as an ideal template for the formation of a nanostructured electroactive material. Because of inter-connections of OMC pores, the electroactive material is widely exposed to the electrolyte to shorten the diffusion paths by minimizing solid-state diffusion.184
OMC can chemically interact with the precursors for in situ synthesis of the electroactive nanocomposites.185 This is obviously accompanied by higher mechanical stability and electrically conductivity. Owing to the mechanical stability of electroactive materials within the OMC architecture, supercapacitors based on OMC nanocomposites show practical cyclability over thousands of cycles.186
Conductive polymers are good candidates for supercapacitors, as their redox systems are normally spread over wide ranges of potentials delivering pseudocapacitive behavior. Although diffusion through the polymer matrix is not very slow, it is still necessary to reduce the solid-state diffusion. Interestingly, monomers can be injected within the OMC mesopores to initiate the polymerization from within the pores.187 Electrochemical polymerization of aniline has also been conducted within the graphite interlayers.140 The specific capacitance of OMC/polyaniline can be over 900 F g−1 with an excellent cyclability over thousands of cycles in a 1 M H2SO4 electrolyte.187 OMC/polyaniline shows a good mechanical stability because of molecular interactions within the nanocomposite matrix.188 It has been shown that the rate capability of an OMC/polypyrrole supercapacitor is about 50% better than pure polypyrrole.189 The OMC/PEDOT supercapacitor showed only 14% capacity loss after 10
000 charge/discharge cycles.190
The conductive polymer formed within the 3D meso-architecture of OMC can be the matrix for additional electroactive materials too. The interesting feature is that all the reactants can chemically interact for designing an in situ synthesis. For instance, polyaniline and manganese oxide directly synthesized within OMC showed a good supercapacitor performance.191–193 An extremely high specific capacitance of 1668 F g−1 has been reported for ruthenium oxide/iron oxide embedded within OMC in a 0.5 M H2SO4 electrolyte.194 In general metal oxides embedded in OMC display high specific capacitance.195
It should be taken into account that the size of mesopores is somewhat comparable to the lattice size of the electroactive material. Thus, the presence of an appropriate amount of reactants within the mesopores is highly effective. For the case of metal oxide, the size, shape, and charge of the reactant anion play a substantial role in the structure and pseudocapacitive behavior of the metal oxide formed within the OMC.119
Comparison of 1D, 2D, and 3D OMCs made from different templates revealed that the ion mobility significantly depends on the diffusion direction in an OMC/Fe2O3 nanocomposite. 1D architectures showed the best rate capability in electrochemical performance, probably because of fewer interactions between the diffusing species.196
The presence of nitrogen heteroatoms in the structure of OMC can significantly improve the interaction with electroactive materials resulting in enhanced specific capacitance.197
4.3. OMC for lithium ion batteries
The important roles of carbonaceous materials whether as active materials or conductive agents in both anodes and cathodes of lithium ion batteries are evident. On the other hand, knowing the fact that the main challenge of lithium batteries has always been related to the slow solid-state diffusion, the ordered interconnected channels of OMC can provide a new opportunity.
Most of the electrode materials of lithium ion batteries are poorly conductive. Carbon is a good conductive agent, but it also blocks the Li diffusion. For instance, LiFePO4 is a promising cathode material, but it needs a conductive agent to reach an acceptable conductivity for charge transfer from the redox sites to the current collector. LiFePO4/OMC has been synthesized by a one-pot route.198 In this direction, OMC paves the way for employing novel candidates, which are not practical standalone systems.199 The 3D structure of OMC is an excellent matrix for the inclusion of electrode materials for battery performance.200
Synthesizing hollow OMC does not result in a high specific surface area (usually in the order of 500 m2 g−1), but as the outer structure has a better electrochemical accessibility, the material displays a high rate capability for lithium ion batteries by reducing the struggles of the Li cation in solid-state diffusion.201,202
The OMC scaffold provides an excellent matrix for the preparation of electroactive nanocomposites as both anode203–207 and cathode198,199 materials. The majority of these nanocomposites are prepared by in situ pore-filling approaches. In this case, the material density and mechanical stability are high enough for practical applications.
In this direction, the OMC architecture is of key importance, as the diffusion of Li-ions is the rate-determining step. The lithium battery performance of Sn nanoparticles embedded within an OMC with larger pores and thinner pore walls has been reported to be better.160
Most of the OMCs are prepared based on silica templates, and the template removal can be a harmful process. Quite interestingly, silica is a promising candidate as an anode material in lithium ion batteries; and preparation of silica/carbon nanocomposites is an effective approach to overcome the low electrical conductivity of the silica component. This suggests that the OMC and its template can be active anode materials for lithium ion batteries.208 In other words, this is indeed an in situ synthesis of a highly ordered nanocomposite.
Pure OMC can be a candidate for anode materials similar to other graphitic carbon nanomaterials. However, the electrochemical performance is significantly poor in comparison with conventional graphite in which Li can be intercalated.209 In fact, although diffusion through mesopores is facilitated, intercalation within the carbon lattice is not favorable in the case of OMC.
In a similar fashion, OMC can be used as an electrode material for other metal ion batteries. For instance, N-doped OMC has been utilized as an anode material in sodium ion batteries.210
4.4. OMC for metal–sulfur batteries
Metal–sulfur batteries have recently attracted considerable attention due to their high specific capacity resulting in an incredibly high energy density. The main challenge of these types of batteries is the sulfur cathode. Since sulfur is non-conductive, the cathode should be prepared using a conductive agent. Like similar battery systems, carbon is the first choice as the conductive agent, and various forms of carbon have been employed for the fabrication of Li–S cathodes.211–214 Octasulfur (cyclo-S8) is the common allotrope of sulfur, and its reduction to the final product of Li2S during the discharge process is a stepwise process, producing Li2Sn (n = 4–8) polysulfide intermediates which are dissoluble in the electrolyte. The dissolved polysulfides shuttle between and react on the sulfur cathode and the lithium anode, resulting in a “chemical shortcut” inside the cell.215 This shuttle phenomenon causes irreversible loss of active materials, capacity fade, self-discharge, and anode etching, among many other detrimental effects. This is the most formidable challenge for the sulfur cathode. Nazar and her coworkers prepared OMC/S as a promising cathode material for Li–S batteries for the first time in 2009.216 The interesting feature of this idea is that sulfur can be entrapped within the OMC structure if the pores are of the right size. This work started a new era in developing Li–S batteries by physically trapping and chemically binding polysulfides in the cathode matrix.
The point is that OMC provides a good permeability for the electroactive species; thus, sulfur can be incorporated within the OMC structure.217–219 This protects sulfur from dissolution while keeping it electrochemically accessible. It is evident that the key challenge for improving the battery performance of OMC/S cathodes is to control the morphology to increase the amount of sulfur entrapment. In general, OMC particles of 200–500 nm with mesopores of 3–10 nm have shown good battery performance with specific capacities over 1000 mA h g−1.
The OMC scaffold can retain a high amount of sulfur, ca. over 50% therein.220 Owing to the mechanical stability of entanglement within the interconnected mesopores, the OMC cathode displays an excellent cyclability. Since sulfur is not a concrete electrode material with a rigid structure like metal oxides, it is subject to severe dissolution. The size of OMC mesopores well fits with the S8 structure formed therein, and thus, the OMC/S cathode shows an excellent cyclability in comparison with other carbonaceous materials.221 However, the performance is not straightforward in practice, as the irreversible inclusion of sulfur within the microporosity of the mesopores results in severe capacity fading. As a matter of fact, OMC can be a good candidate as an S electrode if its architecture is thoroughly designed.
In addition to physically entrapping polysulfides to retard their diffusion out of the cathode, to further prevent the release of sulfur, binding these polar species chemically onto the carbon matrix is another strategy. Since carbon itself is intrinsically non-polar with a lower binding capability to the polar polysulfides, surface functionalization of OMC, including heteroatom doping, conductive polymers or transition metal oxide and sulfide decoration can dramatically enhance the binding capability of OMC to polysulfides.222 With a surface of S-embedded OMC covered by a conductive polymer, a high specific capacity over 1000 mA h g−1 is achieved with an acceptable capacity retention for over 1000 cycles.223 Using PEDOT decorated ordered mesoporous carbon nanocubes to load sulfur, Wang et al.224 demonstrated a sulfur cathode with large capacity, long cycling stability and high rate capability (Fig. 10).
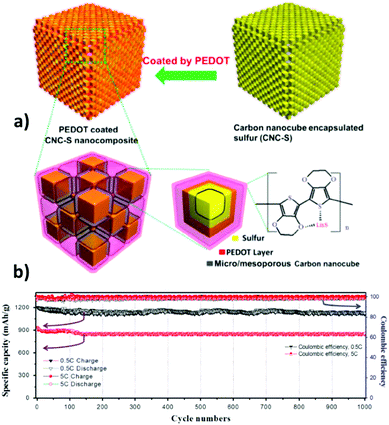 |
| Fig. 10 (a) Schematic of PEDOT decorated mesoporous carbon nanocubes for a sulfur cathode. (b) Cycling performance at 0.5C and 5C, and the corresponding Coulombic efficiencies. Reproduced with permission from ref. 224. Copyright 2015, Elsevier. | |
4.5. OMC for Li–air batteries
In metal–air batteries, such as Li–O2, the key challenge is the porous catalytic cathode material in which Li2O2 is formed and decomposed. It forms the bottleneck since the sluggish ORR/OER kinetics increase the overpotential and limit the recharge capacity and rate performance. The insoluble discharge product (Li2O2) will gradually block the flow of the electrolyte and O2. It has been demonstrated that the OMC skeleton is suitable for the formation of Li2O2.225 The ordered matrix of OMC provides an opportunity for uniform distribution of catalyst nanoparticles. This is of particular importance, as metallic nanoparticles should not block the Li diffusion, which is the main charge carrier. Using an ordered hierarchical mesoporous/macroporous carbon, a high-performance cathode was demonstrated.113
The cathode of metal–air batteries resembles fuel cells and needs ideal electrocatalysts for both the oxygen evolution reaction (OER) and oxygen reduction reaction (ORR) during the charge and discharge processes, respectively. Whether employing the classical Pt catalyst or a new alternative such as metal oxides,226 a key requirement is a uniform distribution of the catalyst over the catalyst support to make the catalyst more accessible electrochemically. Using OMC as an electrocatalyst will be summarized in Section 4.6. Wang et al. fabricated a three-dimensional ordered mesoporous carbon by growing a thin layer of FeOx using atomic layer deposition; when decorated with Pd nanoparticle catalysts, the new cathode exhibits a capacity greater than 6000 mA h g−1 and a cyclability of more than 68 cycles.225Fig. 11 shows the morphological structure and the performance of the OMC/FeOx/Pt electrode.
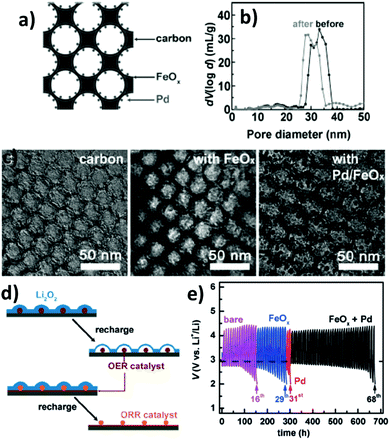 |
| Fig. 11 (a) Schematic of the catalyst loaded OMC structure. (b) Pore size distribution change after FeOx coating. (c) TEM images of pristine OMC, after FeOx coating, and after Pd NP decoration. (d) Schematic illustrating the influence of Li2O2 deposition on the ORR activity of carbon and the subsequent effects of the FeOx coating and Pd NP decoration. (e) Cycling curves for the bare, FeOx coated, and Pd-decorated OMC electrodes. Reproduced with permission from ref. 225. Copyright 2015, Wiley-VCH. | |
Although the current focus is on Li–O2 batteries among metal–air batteries, the possibility of employing OMC is not limited to Li–O2 only. For instance, OMC has been successfully employed for other metal–air cells such as Zn–air cells.227
4.6. OMC as an electrocatalyst support
Electrocatalysts participate in electrochemical reactions by lowering the reaction activation energy and by lowering the excess energy consumed by the activation barrier. They play critical roles in electrochemical fuel (H2, CO, CH4, etc.) production and energy generation (e.g. in fuel cells). A wide range of electrochemical systems have utilized Pt or similar precious metals or transition metal oxides as electrocatalysts. The role of the catalyst support is critically important in these systems to fairly disperse the catalysts to improve the system efficiency and reduce the amount of expensive catalyst required. Carbon is an ideal catalyst support because of its electrical conductivity and high specific surface area, and the mesoporous structure is the best choice for two reasons: simultaneous synthesis of the catalyst can result in the formation of uniform nanoparticles over the mesopores as a template, and catalyst nanoparticles can be uniformly distributed over the mesoporous structure. However, preparation of carbon/catalyst nanocomposites is not as easy as it seems; a significant part of the catalyst is actually covered by carbon and becomes electrochemically inaccessible.228,229
A preliminary study showed that the capability of OMC for dispersing Pt nanoparticles is higher than those of similar carbon nanomaterials.230 This named OMC as a promising catalyst support for various applications, particularly fuel cells. The morphology of micropores is basically responsible for the desirable arrangement of metallic catalysts to be well dispersed while electrochemically accessible.231 In addition to the physical entrapment of metallic nanoparticles within the OMC scaffold, chemical interactions can also improve the catalytic activity of OMC supported Pt.232 Functionalizing OMC with oxygen groups can assist in anchoring the Pt catalyst while preserving the originally ordered structure.233 For the electrochemical oxidation of CO, this reduces the overpotential by about 100–150 mV. Although the fundamental mechanism for this improvement has not been elaborated yet, similar values for various OMCs have been reported by different authors.233,234
Quite interestingly, the catalytic activity of OMC-supported Pt depends on the OMC architecture rather than the mesopore size.235Fig. 12 compares the structure and electrocatalytic activities of two OMCs having an inverse structure; while one is an excellent catalyst support, the other is quite poor.
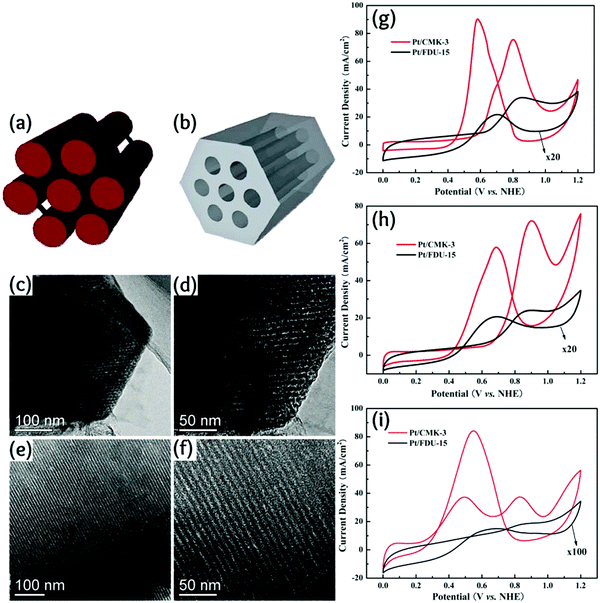 |
| Fig. 12 (a and b) Schematic model, (c–f) TEM images and (g–i) electrocatalytic activities of two OMC samples synthesized using CMK-3 and FDU-15 templates. Reproduced with permission from ref. 235. Copyright 2011, Royal Society of Chemistry. | |
Pt/OMC nanocomposites show excellent electrocatalytic behavior, e.g. for fuel cells.236–238 The electrocatalytic activity of the Pt catalyst supported by OMC for oxygen reduction is two times higher than that supported by commercial conductive carbon.239 A similar behavior has been reported for the electrocatalytic activity of Pd in alkaline fuel cells.240
It has been reported that in the preparation of OMC-supported Pt/Ni bimetallic catalysts, the amount of Ni controls the surface hydroxyl groups, which causes the hydrophilicity of the OMC catalyst support.241 This significantly enhances the electrocatalytic activity for the oxidation of methanol by facilitating the formation of Pt–OH. Another important phenomenon is that increasing the Ni content reduces the size of bimetallic nanoparticles, which can become smaller than the size of mesopores. This is accompanied by a better distribution of Pt and higher accessibility. This enhanced electrocatalytic activity of the bimetallic catalyst is not limited to Ni. PtFex, for example, displays exactly the same behavior.242 A vital necessity for optimum electrocatalytic activity is the integrity of bimetallic nanoparticles over the OMC matrix. The shape and size of the nanoparticles should match those of the mesopores.
OMC is a good compositing agent for metal oxides, which are promising candidates for ORR electrocatalysis, due to the interaction of oxygen groups.243,244
Nitrogen doping improves the properties of OMC as a catalyst support due to the presence of nitrogen coordinated metal centers.141 This can facilitate platinum-free electrocatalysts.245 As explained for the case of supercapacitors, bi-dopants can further contribute to the chemical inhomogeneity of OMC resulting in better charge transfer in electrocatalytic reactions.246 The electrocatalytic activity of nitrogen and sulfur co-doped OMC is comparable to that of Pt/C for the ORR.247 Doping of OMC with N and a metal can lead to superior electrocatalysts. N and Fe co-doped OMC shows a superior electrocatalytic activity for the ORR, even stronger than that of Pt.248
However, the influence of doping is not straightforward as several factors are changed simultaneously. Fig. 13 shows the effect of N-doping on Pt/OMC and Pt–Co/OMC electrocatalysts. In contrast to the enhanced capacitive behavior of N-doped OMC described before, N doping of Pt/OMC reduces both capacitance and electrocatalytic activity. However, N-doping strongly improves both the capacitive and electrocatalytic activity of Pt–Co/OMC. This can be ascribed to several factors. The presence of Co can enhance the graphitization of OMC. As discussed for the bimetallic catalysts before, the presence of Co results in smaller nanoparticles, which are comparable to the mesopore sizes.69 Therefore, the systems are significantly different. This difference is more visible for direct electrochemical oxidation at the electrocatalysts (Fig. 13b). N doping strongly improves the electrocatalytic activity of Pt–Co/OMC but at the price of higher overpotentials, due to the electrochemical redox systems of N-rich functional groups.
 |
| Fig. 13 CV curves of Pt/OMC, Pt/N-OMC, Pt/Co-OMC, and Pt/Co-N-OMC samples in 0.5 M H2SO4 (a) and 2.0 M CH3OH/1.0 M H2SO4 (b) with a scan rate of 20 mV s−1. Reproduced with permission from ref. 69. Copyright 2013, American Chemical Society. | |
The electrocatalytic capability of OMC is not limited to the catalyst support, as it can be directly used as a metal-free catalyst.249 For this purpose, it is necessary to alter the lattice and the surface structure of OMC by doping and surface functionalization. Doping of OMC with various elements such as boron,250 sulfur,251etc. showed an acceptable electrocatalytic activity for the ORR. Similar to the case of supercapacitors, co-doping can further improve the electrocatalytic activity of OMC.252
Although OMC is chemically stable in various media including the alkaline electrolyte, there is a gradual decrease in catalytic activity. In the case of pure OMC, the capacitive behavior is weakened while pseudocapacitive behavior is strengthened, because of the formation of reactive defects and functional groups. For doped OMCs in which there are active redox sites, aging causes a decrease in chemical reactivity.253
The ordered mesoporous structure of OMC provides an excellent opportunity for the formation of 1D catalysts within the OMC structure. This feature has been utilized for in situ synthesis of various catalysts supported on OMC.254,255
Since the pores are usually long along the OMC structure, diffusion of electroactive species is normally unidirectional. Modifying OMC by carbon nanotubes can create shortcuts for charge transfer perpendicular to the pores, and thus, significantly improve the electrocatalytic activity.120,182 Since the electrocatalytic activity is not controlled by the amount of carbon nanotubes, carbon nanotubes are not real channels for diffusion, but shortcuts opening gates in other directions (for charge transfer rather than ion diffusion).256 The same behavior has been reported for the OMC/CNT architecture as an ultracapacitor.257 This is similar to the phenomenon reported for electrodeposition in the presence of carbon nanotubes in which the presence of carbon nanotubes within the diffusion layer just changes the electrodeposition pathway without physical entrapment in the depositing film.258,259 Although the silica/carbon nanocomposite was originally an intermediary template, it can be prepared as a free-standing film with excellent casting capability for practical applications.260
4.7. Electrochemical hydrogen storage
Hydrogen is ideally the greenest fuel, but its applications have been limited by difficulties in storage. In the past two decades, carbon was a promising candidate for storing hydrogen in lieu of metal hydrides storing hydrogen in the bulk. Thus, the surface structure of carbon is of particular importance for achieving an acceptable hydrogen storage capacity. A key problem in hydrogen storage on the carbon surface is that adsorbed H atoms can interact with each other to form H2 and leave the material in gaseous form. OMC seems to be a promising candidate, as adsorbed atoms are somehow entrapped within the mesopores. In addition to the morphological structure, chemical functionality also plays a substantial role in the hydrogen storage capacity of OMC. It has been reported that while oxidized nitrogen functional groups may prevent the adsorption of hydrogen, N-doped OMC showed 1.5 times enhancement in electrochemical hydrogen storage.261,262
While OMC stores hydrogen by surface interaction, metals can store hydrogen in the form of metal hydride. Mixing metal nanoparticles with OMC can assist catalytic hydrogen to dissociate for absorption on the carbon surface while contributing to the hydrogen storage in the form of metal hydride. The Ni/OMC nanocomposite showed a good capacity for electrochemical hydrogen storage.263 As mentioned above, Ni can improve the hydrophilicity of OMC due to the hydrogen functional groups. The capability of OMC-supported Pt/Ni for electrochemical hydrogen storage is increased three times when the Ni content is increased from 2 to 15%.241 A similar behavior has also been observed for OMC-supported NiFe too.264
There is great flexibility for doping of OMC targeting electrochemical applications. Cu and N were utilized as dopants to improve the capacity of OMC for electrochemical hydrogen storage.265
5. Summary and outlook
OMC has attracted considerable attention during the past 5 years for potential applications in various electrochemical energy storage and conversion systems. However, OMC is somewhat considered as a standard material with well-defined architecture, which may be misleading. In practice, at least in electrochemical systems, OMCs are very complicated materials which represent some kind of order at the mesoscale. Both the chemical structures of the pore walls and edges are critically important in electrochemical systems, but less attention is usually paid to comparing similar OMCs in a specific application. On the other hand, not only is the interconnection of the mesopores of essential importance, but also the packing of the material particles can define the overall accessibility of the mesopores. In other words, in a compact electrode, the OMC architecture is not as ordered as seen in the TEM images.
Along with the increasing interest in OMC, there have appeared reports in the literature claiming the unsuitability of OMC for many electrochemical systems. Here, we attempted to highlight two aspects of this potential material: (i) it is a fact that OMC is not as charming and ideal as it is seen in TEM images, and (ii) possible weak performance in electrochemical systems is not necessarily the incapability of OMC for conducting the electrochemical reaction within its ordered architecture. In general, OMC should be specifically tuned for various applications including those of electrochemical energy storage and conversion systems. Some of the reports available in the literature clearly show the potential of OMC for various electrochemical systems, but the “one size fits all” strategy does not work here.
The main obstacle for the practical application of OMC in electrochemical energy systems is the lack of an appropriate synthesis route for the mass production of OMC while designing its architecture for the electrochemical cell. While the conventional silica template method is ideal for the preparation of highly ordered mesopores at a lab scale, there is still no practical approach for scaling up this synthesis route. On the other hand, soft-template methods or other hard templates are more practical for mass production, but at the cost of losing the highly ordered architecture of OMC to some extent. Combining these methods might be a subtle approach for the practical production of OMC. Owing to the essential importance of the pore directions in the OMC architecture, not only the synthesis but also the arrangement of the OMC particles in the electrode composite is of practical importance. Therefore, the OMC should be prepared as a film or directly grown on the current collector to guarantee the original electrochemical properties. In conclusion, we need more fundamental studies to understand how the OMC atomic structure controls the electrochemical processes, instead of typical examinations of OMCs in different systems.
Acknowledgements
Z. F. acknowledges the support from the National Science Foundation (1611060).
References
- Y. Gogotsi, Not Just Graphene: the Wonderful World of Carbon and Related Nanomaterials, MRS Bull., 2015, 40, 1110–1121, DOI:10.1557/mrs.2015.272
.
- M. Lillo-Ródenas, J. Juan-Juan, D. Cazorla-Amorós and A. Linares-Solano, About Reactions Occurring During Chemical Activation with Hydroxides, Carbon, 2004, 42, 1371–1375, DOI:10.1016/j.carbon.2004.01.008
.
- J. Chmiola, Anomalous Increase in Carbon Capacitance at Pore Sizes Less Than 1 Nanometer, Science, 2006, 313, 1760–1763, DOI:10.1126/science.1132195
.
- C. Liang, Z. Li and S. Dai, Mesoporous Carbon Materials: Synthesis and Modification, Angew. Chem., 2008, 47, 3696–3717, DOI:10.1002/anie.200702046
.
- Z. Hu, M. P. Srinivasan and Y. Ni, Preparation of Mesoporous High-Surface-Area Activated Carbon, Adv. Mater., 2000, 12, 62–65, DOI:10.1002/(SICI)1521-4095(200001)12:1<62::AID-ADMA62>3.0.CO;2-B
.
- M. M. Titirici, A. Thomas, S. Yu, J. Müller and M. Antonietti, A Direct Synthesis of Mesoporous Carbons with Bicontinuous Pore Morphology from Crude Plant Material by Hydrothermal Carbonization, Chem. Mater., 2007, 19, 4205–4212, DOI:10.1021/cm0707408
.
- T. Kowalewski, N. V. Tsarevsky and K. Matyjaszewski, Nanostructured Carbon Arrays from Block Copolymers of Polyacrylonitrile, J. Am. Chem. Soc., 2002, 124, 10632–10633, DOI:10.1021/ja0178970
.
- H. Tamai, T. Kakii, Y. Hirota, T. Kumamoto and H. Yasuda, Synthesis of Extremely Large Mesoporous Activated Carbon and Its Unique Adsorption for Giant Molecules, Chem. Mater., 1996, 8, 454–462, DOI:10.1021/cm950381t
.
- H. Yasuda, H. Tamai, M. Ikeuchi and S. Kojima, Extremely Large Mesoporous Carbon Fibers Synthesized by the Addition of Rare Earth Metal Complexes and Their Unique Adsorption Behaviors, Adv. Mater., 1997, 9, 55–58, DOI:10.1002/adma.19970090112
.
- T. Kyotani, Control of Pore Structure in Carbon, Carbon, 2000, 38, 269–286, DOI:10.1016/S0008-6223(99)00142-6
.
- H. Tamon, H. Ishizaka, T. Araki and M. Okazaki, Control of Mesoporous Structure of Organic and Carbon Aerogels, Carbon, 1998, 36, 1257–1262, DOI:10.1016/S0008-6223(97)00202-9
.
- J. H. Knox, K. K. Unger and H. Mueller, Prospects for Carbon As Packing Material in High-Performance Liquid Chromatography, J. Liq. Chromatogr., 2006, 6, 1–36, DOI:10.1080/01483918308067647
.
- C. T. Kresge, M. E. Leonowicz, W. J. Roth, J. C. Vartuli and J. S. Beck, Ordered Mesoporous Molecular Sieves Synthesized by a Liquid-crystal Template Mechanism, Nature, 1992, 359, 710–712, DOI:10.1038/359710a0
.
- J. S. Beck, J. C. Vartuli, W. J. Roth, M. E. Leonowicz, C. T. Kresge, K. D. Schmitt, C. T. W. Chu, D. H. Olson, E. W. Sheppard, S. B. McCullen, J. B. Higgins and J. L. Schlenker, A New Family of Mesoporous Molecular Sieves Prepared with Liquid Crystal Templates, J. Am. Chem. Soc., 1992, 114, 10834–10843, DOI:10.1021/ja00053a020
.
- R. Ryoo, S. H. Joo and S. Jun, Synthesis of Highly Ordered Carbon Molecular Sieves Via Template-Mediated Structural Transformation, J. Phys. Chem. B, 1999, 103, 7743–7746, DOI:10.1021/jp991673a
.
- R. Ryoo, S. H. Joo, M. Kruk and M. Jaroniec, Ordered Mesoporous Carbons, Adv. Mater., 2001, 13, 677–681, DOI:10.1002/1521-4095(200105)13:9<677::aid-adma677>3.0.co;2-c
.
- J. Lee, J. Kim and T. Hyeon, Recent Progress in the Synthesis of Porous Carbon Materials, Adv. Mater., 2006, 18, 2073–2094, DOI:10.1002/adma.200501576
.
- K. Knox, B. Kaur and G. Millward, Structure and Performance of Porous Graphitic Carbon in Liquid Chromatography, J. Chromatogr. A, 1986, 352, 3–25, DOI:10.1016/s0021-9673(01)83368-9
.
- A. Lu and F. Schüth, Nanocasting: a Versatile Strategy for Creating Nanostructured Porous Materials, Adv. Mater., 2006, 18, 1793–1805, DOI:10.1002/adma.200600148
.
- J. Lee, S. Yoon, T. Hyeon, S. M. Oh and K. B. Kim, Synthesis of a New Mesoporous Carbon and Its Application to Electrochemical Double-layer Capacitors, Chem. Commun., 1999, 2177–2178, 10.1039/a906872d
.
- G. S. Chai, S. B. Yoon, J. Yu, J. Choi and Y. Sung, Ordered Porous Carbons with Tunable Pore Sizes As Catalyst Supports in Direct Methanol Fuel Cell, J. Phys. Chem. B, 2004, 108, 7074–7079, DOI:10.1021/jp0370472
.
- Y. Jiao, D. Han, L. Liu, L. Ji, G. Guo, J. Hu, D. Yang and A. Dong, Highly Ordered Mesoporous Few-Layer Graphene Frameworks Enabled by Fe O Nanocrystal Superlattices, Angew. Chem., 2015, 127, 5819–5823, DOI:10.1002/ange.201501398
.
- C. H. Kim, D. Lee and T. J. Pinnavaia, Graphitic Mesostructured Carbon Prepared from Aromatic Precursors, Langmuir, 2004, 20, 5157–5159, DOI:10.1021/la049602c
.
- A. Eftekhari, P. Jafarkhani and F. Moztarzadeh, High-yield Synthesis of Carbon Nanotubes Using a Water-soluble Catalyst Support in Catalytic Chemical Vapor Deposition, Carbon, 2006, 44, 1343–1345, DOI:10.1016/j.carbon.2005.12.006
.
- T. Morishita, T. Tsumura, M. Toyoda, J. Przepiórski, A. Morawski, H. Konno and M. Inagaki, A Review of the Control of Pore Structure in MgO-templated Nanoporous Carbons, Carbon, 2010, 48, 2690–2707, DOI:10.1016/j.carbon.2010.03.064
.
- Y. Yan, Q. Cheng, V. Pavlinek, P. Saha and C. Li, Controlled Synthesis of Mesoporous Carbon Nanosheets and Their Enhanced Supercapacitive Performance, J. Solid State Electrochem., 2013, 17, 1677–1684, DOI:10.1007/s10008-013-2025-3
.
- X. He, R. Li, J. Qiu, K. Xie, P. Ling, M. Yu, X. Zhang and M. Zheng, Synthesis of Mesoporous Carbons for Supercapacitors from Coal Tar Pitch by Coupling Microwave-assisted KOH Activation with a MgO Template, Carbon, 2012, 50, 4911–4921, DOI:10.1016/j.carbon.2012.06.020
.
- M. Kruk, M. Jaroniec, T. Kim and R. Ryoo, Synthesis and Characterization of Hexagonally Ordered Carbon Nanopipes, Chem. Mater., 2003, 15, 2815–2823, DOI:10.1021/cm034087+
.
- A. Lu, W. Schmidt, B. Spliethoff and F. Schüth, Synthesis of Ordered Mesoporous Carbon with Bimodal Pore System and High Pore Volume, Adv. Mater., 2003, 15, 1602–1606, DOI:10.1002/adma.200305176
.
- D. Yuan, F. Zeng, J. Yan, X. Yuan, X. Huang and W. Zou, A Novel Route for Preparing Graphitic Ordered Mesoporous Carbon As Electrochemical Energy Storage Material, RSC Adv., 2013, 3, 5570–5576, 10.1039/c3ra40677f
.
- Y. Wan, Y. Shi and D. Zhao, Supramolecular Aggregates As Templates: Ordered Mesoporous Polymers and Carbons, Chem. Mater., 2008, 20, 932–945, DOI:10.1021/cm7024125
.
- C. Liang, K. Hong, G. A. Guiochon, J. W. Mays and S. Dai, Synthesis of a Large-Scale Highly Ordered Porous Carbon Film by Self-Assembly of Block Copolymers, Angew. Chem., 2004, 43, 5785–5789, DOI:10.1002/anie.200461051
.
- Y. Meng, D. Gu, F. Zhang, Y. Shi, H. Yang, Z. Li, C. Yu, B. Tu and D. Zhao, Ordered Mesoporous Polymers and Homologous Carbon Frameworks: Amphiphilic Surfactant Templating and Direct Transformation, Angew. Chem., 2005, 44, 7053–7059, DOI:10.1002/anie.200501561
.
- Y. Huang, H. Cai, D. Feng, D. Gu, Y. Deng, B. Tu, H. Wang, P. A. Webley and D. Zhao, One-step Hydrothermal Synthesis of Ordered Mesostructured Carbonaceous Monoliths with Hierarchical Porosities, Chem. Commun., 2008, 2641–2643, 10.1039/b804716b
.
- Y. Deng, C. Liu, T. Yu, F. Liu, F. Zhang, Y. Wan, L. Zhang, C. Wang, B. Tu, P. A. Webley, H. Wang and D. Zhao, Facile Synthesis of Hierarchically Porous Carbons from Dual Colloidal Crystal/Block Copolymer Template Approach, Chem. Mater., 2007, 19, 3271–3277, DOI:10.1021/cm070600y
.
- T. Ma, L. Liu and Z. Yuan, Direct Synthesis of Ordered Mesoporous Carbons, Chem. Soc. Rev., 2013, 42, 3977–4003, 10.1039/c2cs35301f
.
- Y. Fang, D. Gu, Y. Zou, Z. Wu, F. Li, R. Che, Y. Deng, B. Tu and D. Zhao, A Low-Concentration Hydrothermal Synthesis of Biocompatible Ordered Mesoporous Carbon Nanospheres with Tunable and Uniform Size, Angew. Chem., 2010, 49, 7987–7991, DOI:10.1002/anie.201002849
.
- F. Zhang, D. Gu, T. Yu, F. Zhang, S. Xie, L. Zhang, Y. Deng, Y. Wan, B. Tu and D. Zhao, Mesoporous Carbon Single-Crystals from Organic–Organic Self-Assembly, J. Am. Chem. Soc., 2007, 129, 7746–7747, DOI:10.1021/ja072316d
.
- M. Steinhart, C. Liang, G. W. Lynn, U. Gösele and S. Dai, Direct Synthesis of Mesoporous Carbon Microwires and Nanowires, Chem. Mater., 2007, 19, 2383–2385, DOI:10.1021/cm070455o
.
- K. Wang, P. Birjukovs, D. Erts, R. Phelan, M. A. Morris, H. Zhou and J. D. Holmes, Synthesis and Characterisation of Ordered Arrays of Mesoporous Carbon Nanofibres, J. Mater. Chem., 2009, 19, 1331–1338, 10.1039/b817156d
.
- L. Liu, F. Wang, G. Shao and Z. Yuan, A Low-temperature Autoclaving Route to Synthesize Monolithic Carbon Materials with an Ordered Mesostructure, Carbon, 2010, 48, 2089–2099, DOI:10.1016/j.carbon.2010.02.022
.
- Y. Meng, D. Gu, F. Zhang, Y. Shi, L. Cheng, D. Feng, Z. Wu, Z. Chen, Y. Wan, A. Stein and D. Zhao, A Family of Highly Ordered Mesoporous Polymer Resin and Carbon Structures from Organic–Organic Self-Assembly, Chem. Mater., 2006, 18, 4447–4464, DOI:10.1021/cm060921u
.
- F. Zhang, Y. Meng, D. Gu, Y. Yan, C. Yu, B. Tu and D. Zhao, A Facile Aqueous Route to Synthesize Highly Ordered Mesoporous Polymers and Carbon Frameworks with
Bicontinuous Cubic Structure, J. Am. Chem. Soc., 2005, 127, 13508–13509, DOI:10.1021/ja0545721
.
- A. Stein, Z. Wang and M. A. Fierke, Functionalization of Porous Carbon Materials with Designed Pore Architecture, Adv. Mater., 2009, 21, 265–293, DOI:10.1002/adma.200801492
.
- T. Otowa, Y. Nojima and T. Miyazaki, Development of KOH Activated High Surface Area Carbon and Its Application to Drinking Water Purification, Carbon, 1997, 35, 1315–1319, DOI:10.1016/S0008-6223(97)00076-6
.
- S. Roldán, I. Villar, V. Ruíz, C. Blanco, M. Granda, R. Menendez and R. Santamaría, Comparison Between Electrochemical Capacitors Based on NaOH- and KOH-Activated Carbons, Energy Fuels, 2010, 24, 3422–3428, DOI:10.1021/ef901538m
.
- Y. Lv, F. Zhang, Y. Dou, Y. Zhai, J. Wang, H. Liu, Y. Xia, B. Tu and D. Zhao, A Comprehensive Study on
KOH Activation of Ordered Mesoporous Carbons and Their Supercapacitor Application, J. Mater. Chem., 2012, 22, 93–99, 10.1039/c1jm12742j
.
- P. F. Fulvio, R. T. Mayes, X. Wang, S. M. Mahurin, J. C. Bauer, V. Presser, J. McDonough, Y. Gogotsi and S. Dai, “Brick-and-Mortar” Self-Assembly Approach to Graphitic Mesoporous Carbon Nanocomposites, Adv. Funct. Mater., 2011, 21, 2208–2215, DOI:10.1002/adfm.201002641
.
- A. B. Fuertes and S. Álvarez, Graphitic Mesoporous Carbons Synthesised Through Mesostructured Silica Templates, Carbon, 2004, 42, 3049–3055, DOI:10.1016/j.carbon.2004.06.020
.
- H. Yang, Y. Yan, Y. Liu, F. Zhang, R. Zhang, Y. Yan, M. Li, S. Xie, B. Tu and D. Zhao, A Simple Melt Impregnation Method to Synthesize Ordered Mesoporous Carbon and Carbon Nanofiber Bundles with Graphitized Structure from Pitches, J. Phys. Chem. B, 2004, 108, 17320–17328, DOI:10.1021/jp046948n
.
- A. Vinu, S. Anandan, C. Anand, P. Srinivasu, K. Ariga and T. Mori, Fabrication of Partially Graphitic Three-dimensional Nitrogen-doped Mesoporous Carbon Using Polyaniline Nanocomposite Through Nanotemplating Method, Microporous Mesoporous Mater., 2008, 109, 398–404, DOI:10.1016/j.micromeso.2007.05.037
.
- Z. Li and M. Jaroniec, Mesoporous Carbons Synthesized by Imprinting Ordered and Disordered Porous Structures of Silica Particles in Mesophase Pitch, J. Phys. Chem. B, 2004, 108, 824–826, DOI:10.1021/jp0368233
.
- M. Kruk, K. M. Kohlhaas, B. Dufour, E. B. Celer, M. Jaroniec, K. Matyjaszewski, R. S. Ruoff and T. Kowalewski, Partially Graphitic, High-surface-area Mesoporous Carbons from Polyacrylonitrile Templated by Ordered and Disordered Mesoporous Silicas, Microporous Mesoporous Mater., 2007, 102, 178–187, DOI:10.1016/j.micromeso.2006.12.027
.
- M. Kruk, B. Dufour, E. B. Celer, T. Kowalewski, M. Jaroniec and K. Matyjaszewski, Synthesis of Mesoporous Carbons Using Ordered and Disordered Mesoporous Silica Templates and Polyacrylonitrile As Carbon Precursor, J. Phys. Chem. B, 2005, 109, 9216–9225, DOI:10.1021/jp045594x
.
- A. Lu, A. Kiefer, W. Schmidt and F. Schüth, Synthesis of Polyacrylonitrile-Based Ordered Mesoporous Carbon with Tunable Pore Structures, Chem. Mater., 2004, 16, 100–103, DOI:10.1021/cm031095h
.
- T. Kim, I. Park and R. Ryoo, A Synthetic Route to Ordered Mesoporous Carbon Materials with Graphitic Pore Walls, Angew. Chem., 2003, 115, 4511–4515, DOI:10.1002/ange.200352224
.
- Y. Xia and R. Mokaya, Generalized and Facile Synthesis Approach to N-Doped Highly Graphitic Mesoporous Carbon Materials, Chem. Mater., 2005, 17, 1553–1560, DOI:10.1021/cm048057y
.
- Y. Xia and R. Mokaya, Synthesis of Ordered Mesoporous Carbon and Nitrogen-Doped Carbon Materials with Graphitic Pore Walls Via a Simple Chemical Vapor Deposition Method, Adv. Mater., 2004, 16, 1553–1558, DOI:10.1002/adma.200400391
.
- Y. Xia, Z. Yang and R. Mokaya, Simultaneous Control of Morphology and Porosity in Nanoporous Carbon: Graphitic Mesoporous Carbon Nanorods and Nanotubules with Tunable Pore Size, Chem. Mater., 2006, 18, 140–148, DOI:10.1021/cm0522634
.
- K. Lee, X. Ji, M. Rault and L. Nazar, Simple Synthesis of Graphitic Ordered Mesoporous Carbon Materials by a Solid-State Method Using Metal Phthalocyanines, Angew. Chem., 2009, 48, 5661–5665, DOI:10.1002/anie.200806208
.
- K. P. Gierszal, M. Jaroniec, T. Kim, J. Kim and R. Ryoo, High Temperature Treatment of Ordered Mesoporous Carbons Prepared by Using Various Carbon Precursors and Ordered Mesoporous Silica Templates, New J. Chem., 2008, 32, 981–993, 10.1039/b716735k
.
- Y. Wang, C. Zhang, S. Kang, B. Li, Y. Wang, L. Wang and X. Li, Simple Synthesis of Graphitic Ordered Mesoporous Carbon Supports Using Natural Seed Fat, J.
Mater. Chem., 2011, 21, 14420–14423, 10.1039/c1jm13054d
.
- W. Gao, Y. Wan, Y. Dou and D. Zhao, Synthesis of Partially Graphitic Ordered Mesoporous Carbons with High Surface Areas, Adv. Energy Mater., 2011, 1, 115–123, DOI:10.1002/aenm.201000009
.
- S. Tanaka, N. Nishiyama, Y. Egashira and K. Ueyama, Synthesis of Ordered Mesoporous Carbons with Channel Structure from an Organic–organic Nanocomposite, Chem. Commun., 2005, 2125–2127, 10.1039/b501259g
.
- L. Wang, L. Sun, C. Tian, T. Tan, G. Mu, H. Zhang and H. Fu, A Novel Soft Template Strategy to Fabricate Mesoporous Carbon/graphene Composites As High-performance Supercapacitor Electrodes, RSC Adv., 2012, 2, 8359–8367, 10.1039/c2ra20845h
.
- J. Zhi, W. Zhao, X. Liu, A. Chen, Z. Liu and F. Huang, Highly Conductive Ordered Mesoporous Carbon Based Electrodes Decorated by 3D Graphene and 1D Silver Nanowire for Flexible Supercapacitor, Adv. Funct. Mater., 2014, 24, 2013–2019, DOI:10.1002/adfm.201303082
.
- M. Li, J. Ding and J. Xue, Mesoporous Carbon Decorated Graphene As an Efficient Electrode Material for Supercapacitors, J. Mater. Chem. A, 2013, 1, 7469–7476, 10.1039/c3ta10890b
.
- M. Dai, L. Song, J. T. LaBelle and B. D. Vogt, Ordered Mesoporous Carbon Composite Films Containing Cobalt Oxide and Vanadia for Electrochemical Applications, Chem. Mater., 2011, 23, 2869–2878, DOI:10.1021/cm2002429
.
- J. Tang, T. Wang, X. Pan, X. Sun, X. Fan, Y. Guo, H. Xue and J. He, Synthesis and Electrochemical Characterization of N-Doped Partially Graphitized Ordered Mesoporous Carbon–Co Composite, J. Phys. Chem. C, 2013, 117, 16896–16906, DOI:10.1021/jp405775x
.
- C. Lee, S. Yoon, H. Kim, H. Youn, J. Han, K. C. Roh and K. Kim, A Two-dimensional Highly Ordered Mesoporous Carbon/graphene Nanocomposite for Electrochemical Double Layer Capacitors: Effects of Electrical and Ionic Conduction Pathways, J. Mater. Chem. A, 2015, 3, 2314–2322, 10.1039/c4ta05863a
.
- R. Liu, L. Pan, X. Liu and D. Wu, An Evaporation-induced Tri-constituent Assembly Approach to Fabricate an Ordered Mesoporous Carbon/graphene Aerogel for High-performance Supercapacitors, RSC Adv., 2015, 5, 16765–16768, 10.1039/c4ra13720e
.
- Y. Song, J. Yang, K. Wang, S. Haller, Y. Wang, C. Wang and Y. Xia, In-situ Synthesis of Graphene/nitrogen-doped Ordered Mesoporous Carbon Nanosheet for Supercapacitor Application, Carbon, 2016, 96, 955–964, DOI:10.1016/j.carbon.2015.10.060
.
- Y. Lv, Y. Feng, L. Gan, M. Liu, L. Xu, C. Liu, H. Zheng and J. Li, Synthesis of Co-containing Mesoporous Carbon Foams Using a New Cobalt-oxo Cluster As a Precursor, J. Solid State Chem., 2012, 185, 198–205, DOI:10.1016/j.jssc.2011.11.010
.
- X. Xu, Y. Liu, M. Wang, C. Zhu, T. Lu, R. Zhao and L. Pan, Hierarchical Hybrids with Microporous Carbon Spheres Decorated Three-dimensional Graphene Frameworks for Capacitive Applications in Supercapacitor and Deionization, Electrochim. Acta, 2016, 193, 88–95, DOI:10.1016/j.electacta.2016.02.049
.
- J. L. Vickery, A. J. Patil and S. Mann, Fabrication of Graphene-Polymer Nanocomposites with Higher-Order Three-Dimensional Architectures, Adv. Mater., 2009, 21, 2180–2184, DOI:10.1002/adma.200803606
.
- J. Wang and M. Ellsworth, Graphene Aerogels, ECS Trans., 2009, 19, 241–247, DOI:10.1149/1.3119548
.
- Z. Xu, Y. Zhang, P. Li and C. Gao, Strong, Conductive, Lightweight, Neat Graphene Aerogel Fibers with Aligned Pores, ACS Nano, 2012, 6, 7103–7113, DOI:10.1021/nn3021772
.
- Z. Chen, W. Ren, L. Gao, B. Liu, S. Pei and H. Cheng, Three-dimensional Flexible and Conductive Interconnected Graphene Networks Grown by Chemical Vapour Deposition, Nat. Mater., 2011, 10, 424–428, DOI:10.1038/nmat3001
.
- J. Lee, S. Kim, J. Yoon and J. Jang, Chemical Vapor Deposition of Mesoporous Graphene Nanoballs for Supercapacitor, ACS Nano, 2013, 7, 6047–6055, DOI:10.1021/nn401850z
.
- H. Yu, G. Guo, L. Ji, H. Li, D. Yang, J. Hu and A. Dong, Designed Synthesis of Ordered Mesoporous Graphene Spheres from Colloidal Nanocrystals and Their Application As a Platform for High-performance Lithium-ion Battery Composite Electrodes, Nano Res., 2016, 9, 1–15, DOI:10.1007/s12274-016-1246-z
.
- T. Lin, I. Chen, F. Liu, C. Yang, H. Bi, F. Xu and F. Huang, Nitrogen-doped Mesoporous Carbon of Extraordinary Capacitance for Electrochemical Energy Storage, Science, 2015, 350, 1508–1513, DOI:10.1126/science.aab3798
.
- A. Eftekhari and P. Jafarkhani, Curly Graphene with Specious Interlayers Displaying Superior Capacity for Hydrogen Storage, J. Phys. Chem. C, 2013, 117, 25845–25851, DOI:10.1021/jp410044v
.
- R. L. McCreery, Advanced Carbon Electrode Materials for Molecular Electrochemistry, Chem. Rev., 2008, 108, 2646–2687, DOI:10.1021/cr068076m
.
- J. Randin and E. Yeager, Differential Capacitance Study of Stress-Annealed Pyrolytic Graphite Electrodes, J. Electrochem. Soc., 1971, 118, 711–714, DOI:10.1149/1.2408151
.
- N. G. Shang, P. Papakonstantinou, M. McMullan, M. Chu, A. Stamboulis, A. Potenza, S. S. Dhesi and H. Marchetto, Catalyst-Free Efficient Growth, Orientation and Biosensing Properties of Multilayer Graphene Nanoflake Films with Sharp Edge Planes, Adv. Funct. Mater., 2008, 18, 3506–3514, DOI:10.1002/adfm.200800951
.
- J. R. Miller, R. A. Outlaw and B. C. Holloway, Graphene Double-Layer Capacitor with Ac Line-Filtering Performance, Science, 2010, 329, 1637–1639, DOI:10.1126/science.1194372
.
- G. Ren, M. N. F. Hoque, X. Pan, J. Warzywoda and Z. Fan, Vertically Aligned VO (B) Nanobelt Forest and Its Three-dimensional Structure on Oriented Graphene for Energy Storage, J. Mater. Chem. A, 2015, 3, 10787–10794, 10.1039/c5ta01900a
.
- X. Pan, K. Zhu, G. Ren, N. Islam, J. Warzywoda and Z. Fan, Electrocatalytic Properties of a Vertically Oriented Graphene Film and Its Application As a Catalytic Counter Electrode for Dye-sensitized Solar Cells, J. Mater. Chem. A, 2014, 2, 12746–12753, 10.1039/c4ta02028f
.
- G. Ren, X. Pan, S. Bayne and Z. Fan, Kilohertz Ultrafast Electrochemical Supercapacitors Based on Perpendicularly-oriented Graphene Grown Inside of Nickel Foam, Carbon, 2014, 71, 94–101, DOI:10.1016/j.carbon.2014.01.017
.
- G. Ren, S. Li, Z. Fan, M. N. F. Hoque and Z. Fan, Ultrahigh-rate Supercapacitors with Large Capacitance Based on Edge Oriented Graphene Coated Carbonized Cellulous Paper As Flexible Freestanding Electrodes, J. Power Sources, 2016, 325, 152–160, DOI:10.1016/j.jpowsour.2016.06.021
.
- G. Ren, M. N. F. Hoque, J. Liu, J. Warzywoda and Z. Fan, Perpendicular Edge Oriented Graphene Foam Supporting Orthogonal TiO2(B) Nanosheets As Freestanding Electrode for Lithium Ion Battery, Nano Energy, 2016, 21, 162–171, DOI:10.1016/j.nanoen.2016.01.010
.
- H. Wang, B. Qi, B. Lu, X. Bo and L. Guo, Comparative Study on the Electrocatalytic Activities of Ordered Mesoporous Carbons and Graphene, Electrochim. Acta, 2011, 56, 3042–3048, DOI:10.1016/j.electacta.2010.12.099
.
- W. Xing, S. Qiao, R. Ding, F. Li, G. Lu, Z. Yan and H. Cheng, Superior Electric Double Layer Capacitors Using Ordered Mesoporous Carbons, Carbon, 2006, 44, 216–224, DOI:10.1016/j.carbon.2005.07.029
.
- L. Li, H. Song and X. Chen, Pore Characteristics and Electrochemical Performance of Ordered Mesoporous Carbons for Electric Double-layer Capacitors, Electrochim. Acta, 2006, 51, 5715–5720, DOI:10.1016/j.electacta.2006.03.005
.
- Y. Korenblit, M. Rose, E. Kockrick, L. Borchardt, A. Kvit, S. Kaskel and G. Yushin, High-Rate Electrochemical Capacitors Based on Ordered Mesoporous Silicon Carbide-Derived Carbon, ACS Nano, 2010, 4, 1337–1344, DOI:10.1021/nn901825y
.
- S. Woo, K. Dokko, H. Nakano and K. Kanamura, Preparation of Three Dimensionally Ordered Macroporous Carbon with Mesoporous Walls for Electric Double-layer Capacitors, J. Mater. Chem., 2008, 18, 1674–1680, 10.1039/b717996k
.
- H. Liu, J. Wang, C. Wang and Y. Xia, Ordered Hierarchical Mesoporous/Microporous Carbon Derived from Mesoporous Titanium-Carbide/Carbon Composites and Its Electrochemical Performance in Supercapacitor, Adv. Energy Mater., 2011, 1, 1101–1108, DOI:10.1002/aenm.201100255
.
- J. Zhi, S. Deng, Y. Wang and A. Hu, Highly Ordered Metal Oxide Nanorods Inside Mesoporous Silica Supported Carbon Nanomembranes: High Performance Electrode Materials for Symmetrical Supercapacitor Devices, J. Phys. Chem. C, 2015, 119, 8530–8536, DOI:10.1021/acs.jpcc.5b01230
.
- W. Li, G. Nong, A. Lu and H. Hu, Synthesis of Nanocast Ordered Mesoporous Carbons and Their Application As Electrode Materials for Supercapacitor, J. Porous Mater., 2009, 18, 23–30, DOI:10.1007/s10934-009-9352-x
.
- W. Kim, M. Y. Kang, J. B. Joo, N. D. Kim, I. K. Song, P. Kim, J. R. Yoon and J. Yi, Preparation of Ordered Mesoporous Carbon Nanopipes with Controlled Nitrogen Species for Application in Electrical Double-layer Capacitors, J. Power Sources, 2010, 195, 2125–2129, DOI:10.1016/j.jpowsour.2009.09.080
.
- J. Zhang, L. Kong, J. Cai, Y. Luo and L. Kang, Nanoflake-like Cobalt Hydroxide/ordered Mesoporous Carbon Composite for Electrochemical Capacitors, J. Solid State Electrochem., 2010, 14, 2065–2075, DOI:10.1007/s10008-010-1035-7
.
- Y. Wang, D. Zhou, D. Zhao, M. Hou, C. Wang and Y. Xia, High Performance Hybrid Supercapacitor Based on Graphene-Supported Ni(OH)2-Nanowires and Ordered Mesoporous Carbon CMK-5, J. Electrochem. Soc., 2012, 160, A98, DOI:10.1149/2.012302jes
.
- C. Yuan, S. Xiong, X. Zhang, L. Shen, F. Zhang, B. Gao and L. Su, Template-free Synthesis of Ordered Mesoporous NiO/poly(sodium-4-styrene Sulfonate) Functionalized Carbon Nanotubes Composite for Electrochemical Capacitors, Nano Res., 2009, 2, 722–732, DOI:10.1007/s12274-009-9079-7
.
- X. Dong, Z. Guo, Y. Song, M. Hou, J. Wang, Y. Wang and Y. Xia, Flexible and Wire-Shaped Micro-Supercapacitor Based on Ni(OH)2-Nanowire and Ordered Mesoporous Carbon Electrodes, Adv. Funct. Mater., 2014, 24, 3405–3412, DOI:10.1002/adfm.201304001
.
- H. Li, R. Wang and R. Cao, Physical and Electrochemical Characterization of Hydrous Ruthenium Oxide/ordered Mesoporous Carbon Composites As Supercapacitor, Microporous Mesoporous Mater., 2008, 111, 32–38, DOI:10.1016/j.micromeso.2007.07.002
.
- J. C. Ndamanisha and L. Guo, Ordered Mesoporous Carbon for Electrochemical Sensing: A Review, Anal. Chim. Acta, 2012, 747, 19–28, DOI:10.1016/j.aca.2012.08.032
.
- D. A. C. Brownson, D. K. Kampouris and C. E. Banks, Graphene Electrochemistry: Fundamental Concepts Through to Prominent Applications, Chem. Soc. Rev., 2012, 41, 6944–6976, 10.1039/c2cs35105f
.
- A. Ambrosi, C. K. Chua, A. Bonanni and M. Pumera, Electrochemistry of Graphene and Related Materials, Chem. Rev., 2014, 114, 7150–7188, DOI:10.1021/cr500023c
.
- W. Yuan, Y. Zhou, Y. Li, C. Li, H. Peng, J. Zhang, Z. Liu, L. Dai and G. Shi, The Edge- and Basal-plane-specific Electrochemistry of a Single-layer Graphene Sheet, Sci. Rep., 2013, 3, 02248, DOI:10.1038/srep02248
.
- T. J. Davies, M. E. Hyde and R. G. Compton, Nanotrench Arrays Reveal Insight into Graphite Electrochemistry, Angew. Chem., 2005, 44, 5121–5126, DOI:10.1002/anie.200462750
.
- C. E. Banks, A. Crossley, C. Salter, S. J. Wilkins and R. G. Compton, Carbon Nanotubes Contain Metal Impurities Which Are Responsible for the “Electrocatalysis” Seen at Some Nanotube-Modified Electrodes, Angew. Chem., 2006, 45, 2533–2537, DOI:10.1002/anie.200600033
.
- S. Leyva-García, D. Lozano-Castelló, E. Morallón and D. Cazorla-Amorós, Silica-templated Ordered Mesoporous Carbon Thin Films As Electrodes for Micro-capacitors, J. Mater. Chem. A, 2016, 4, 4570–4579, 10.1039/c5ta10552h
.
- Z. Guo, D. Zhou, X. Dong, Z. Qiu, Y. Wang and Y. Xia, Ordered Hierarchical Mesoporous/Macroporous Carbon: a High-Performance Catalyst for Rechargeable Li–O Batteries, Adv. Mater., 2013, 25, 5668–5672, DOI:10.1002/adma.201302459
.
- Y. Yang, P. Li, S. Wu, X. Li, E. Shi, Q. Shen, D. Wu, W. Xu, A. Cao and Q. Yuan, Hierarchically Designed Three-Dimensional Macro/Mesoporous Carbon Frameworks for Advanced Electrochemical Capacitance Storage, Chem. – Eur. J., 2015, 21, 6157–6164, DOI:10.1002/chem.201406199
.
- G. S. Chai, I. S. Shin and J. Yu, Synthesis of Ordered, Uniform, Macroporous Carbons with Mesoporous Walls Templated by Aggregates of Polystyrene Spheres and Silica Particles for Use As Catalyst Supports in Direct Methanol Fuel Cells, Adv. Mater., 2004, 16, 2057–2061, DOI:10.1002/adma.200400283
.
- S. Zhu, H. Zhou, M. Hibino, I. Honma and M. Ichihara, Synthesis of MnO2 Nanoparticles Confined in Ordered Mesoporous Carbon Using a Sonochemical Method, Adv. Funct. Mater., 2005, 15, 381–386, DOI:10.1002/adfm.200400222
.
- X. Zhang, W. Dong, A. Lu and W. Li, Rational Design of Mesoporous Carbon Electrodes with High Mass Loading for Binder-Free Supercapacitors, Energy Technol., 2015, 3, 234–241, DOI:10.1002/ente.201402161
.
- J. Hu, M. Noked, E. Gillette, F. Han, Z. Gui, C. Wang and S. B. Lee, Dual-template Synthesis of Ordered Mesoporous Carbon/Fe O Nanowires: High Porosity and Structural Stability for Supercapacitors, J. Mater. Chem. A, 2015, 3, 21501–21510, 10.1039/c5ta06372h
.
- F. Pico, E. Morales, J. Fernandez, T. Centeno, J. Ibañez, R. Rojas, J. Amarilla and J. Rojo, Ruthenium Oxide/carbon Composites with Microporous or Mesoporous Carbon As Support and Prepared by Two Procedures. A Comparative Study As Supercapacitor Electrodes, Electrochim. Acta, 2009, 54, 2239–2245, DOI:10.1016/j.electacta.2008.10.028
.
- X. Jia, X. Zhu, Y. Cheng, Z. Chen, G. Ning, Y. Lu and F. Wei, Aerosol-Assisted Heteroassembly of Oxide Nanocrystals and Carbon Nanotubes into 3D Mesoporous Composites for High-Rate Electrochemical Energy Storage, Small, 2015, 11, 3135–3142, DOI:10.1002/smll.201403196
.
- S. Yoon, S. M. Oh, C. Wee Lee and J. H. Ryu, Pore Structure Tuning of Mesoporous Carbon Prepared by Direct Templating Method for Application to High Rate Supercapacitor Electrodes, J. Electroanal. Chem., 2011, 650, 187–195, DOI:10.1016/j.jelechem.2010.10.008
.
- Y. Gogotsi and P. Simon, True Performance Metrics in Electrochemical Energy Storage, Science, 2011, 334, 917–918, DOI:10.1126/science.1213003
.
- H. Li, J. Luo, X. Zhou, C. Yu and Y. Xia, An Ordered Mesoporous Carbon with Short Pore Length and Its Electrochemical Performances in Supercapacitor Applications, J. Electrochem. Soc., 2007, 154, A731, DOI:10.1149/1.2741198
.
- H. Li, R. Liu, D. Zhao and Y. Xia, Electrochemical Properties of an Ordered Mesoporous Carbon Prepared by Direct Tri-constituent Co-assembly, Carbon, 2007, 45, 2628–2635, DOI:10.1016/j.carbon.2007.08.005
.
- T. V. Magdesieva, P. V. Shvets, O. M. Nikitin, E. A. Obraztsova, F. T. Tuyakova, V. G. Sergeyev, A. R. Khokhlov and A. N. Obraztsov, Electrochemical Characterization of Mesoporous Nanographite Films, Carbon, 2016, 105, 96–102, DOI:10.1016/j.carbon.2016.04.028
.
- G. Shen, X. Sun, H. Zhang, Y. Liu, J. Zhang, A. Meka, L. Zhou and C. Yu, Nitrogen-doped Ordered Mesoporous Carbon Single Crystals: Aqueous Organic–organic Self-assembly and Superior Supercapacitor Performance, J. Mater. Chem. A, 2015, 3, 24041–24048, 10.1039/c5ta06129f
.
- J. Li, E. Fiset, J. Yang, P. Yuan, X. Ling, D. Hulicova-Jurcakova, C. Yu and L. Wang, Formation of Graphitic Tubules from Ordered Mesoporous Carbon and Their Effect on Supercapacitive Energy Storage, J. Mater. Chem., 2012, 22, 21472–21480, 10.1039/c2jm33889k
.
- S. Inagaki, Y. Yokoo, T. Miki and Y. Kubota, Improvement of Electric Double-layer Capacitance of Ordered Mesoporous Carbon CMK-3 by Partial Graphitization Using Metal Oxide Catalysts, Microporous Mesoporous Mater., 2013, 179, 136–143, DOI:10.1016/j.micromeso.2013.05.024
.
- X. Yu, J. Wang, Z. Huang, W. Shen and F. Kang, Ordered Mesoporous Carbon Nanospheres As Electrode Materials for High-performance Supercapacitors, Electrochem. Commun., 2013, 36, 66–70, DOI:10.1016/j.elecom.2013.09.010
.
- C. Zheng, L. Qi, M. Yoshio and H. Wang, Cooperation of Micro- and Meso-porous Carbon Electrode Materials in Electric Double-layer Capacitors, J. Power Sources, 2010, 195, 4406–4409, DOI:10.1016/j.jpowsour.2010.01.041
.
- D. Zhou, W. Li, X. Dong, Y. Wang, C. Wang and Y. Xia, A Nitrogen-doped Ordered Mesoporous Carbon Nanofiber Array for Supercapacitors, J. Mater. Chem. A, 2013, 1, 8488–8496, 10.1039/c3ta11667k
.
- E. Kang, G. Jeon and J. K. Kim, Free-standing, Well-aligned Ordered Mesoporous Carbon Nanofibers on Current Collectors for High-power Micro-supercapacitors, Chem. Commun., 2013, 49, 6406–6408, 10.1039/c3cc42436g
.
- Q. Zhang, L. Li, Y. Wang, Y. Chen, F. He, S. Gai and P. Yang, Uniform Fibrous-structured Hollow Mesoporous Carbon Spheres for High-performance Supercapacitor Electrodes, Electrochim. Acta, 2015, 176, 542–547, DOI:10.1016/j.electacta.2015.06.154
.
- J. Xue, C. Henry, J. Lee and B. D. Vogt, Large Area, Flexible Ordered Mesoporous Carbon Films from Soft Templating on Polymer Substrates, RSC Adv., 2014, 4, 3669–3677, 10.1039/c3ra44723e
.
- Y. J. Oh, J. J. Yoo, Y. I. Kim, J. K. Yoon, H. N. Yoon, J. Kim and S. B. Park, Oxygen Functional Groups and Electrochemical Capacitive Behavior of Incompletely Reduced Graphene Oxides As a Thin-film Electrode of Supercapacitor, Electrochim. Acta, 2014, 116, 118–128, DOI:10.1016/j.electacta.2013.11.040
.
- S. Li, S. Yang, Y. Wang, H. Tsai, H. Tien, S. Hsiao, W. Liao, C. Chang, C. M. Ma and C. Hu, N-doped Structures and Surface Functional Groups of Reduced Graphene Oxide and Their Effect on the Electrochemical Performance of Supercapacitor with Organic Electrolyte, J. Power Sources, 2015, 278, 218–229, DOI:10.1016/j.jpowsour.2014.12.025
.
- G. Park, S. K. Park, J. Han, T. Y. Ko, S. Lee, J. Oh, S. Ryu, H. S. Park and S. Park, Finely Tuning Oxygen Functional Groups of Graphene Materials and Optimizing Oxygen Levels for Capacitors, RSC Adv., 2014, 4, 36377–36384, 10.1039/c4ra02873b
.
- F. Lufrano and P. Staiti, Influence of the Surface—Chemistry of Modified Mesoporous Carbon on the Electrochemical Behavior of Solid-State Supercapacitors, Energy Fuels, 2010, 24, 3313–3320, DOI:10.1021/ef901447y
.
- T. Zhu, Y. Lu, S. Zheng, Y. Chen and H. Guo, Influence of Nitric Acid Acitivation on Structure and Capacitive Performances of Ordered Mesoporous Carbon, Electrochim. Acta, 2015, 152, 456–463, DOI:10.1016/j.electacta.2014.11.161
.
- A. Eftekhari and B. Yazdani, Initiating Electropolymerization on Graphene Sheets in Graphite Oxide Structure, J. Polym. Sci., Part A: Polym. Chem., 2010, 48, 2204–2213, DOI:10.1002/pola.23990
.
- G. Lota, K. Lota and E. Frackowiak, Nanotubes Based Composites Rich in Nitrogen for Supercapacitor Application, Electrochem. Commun., 2007, 9, 1828–1832, DOI:10.1016/j.elecom.2007.04.015
.
- W. Li, D. Chen, Z. Li, Y. Shi, Y. Wan, J. Huang, J. Yang, D. Zhao and Z. Jiang, Nitrogen Enriched Mesoporous Carbon Spheres Obtained by a Facile Method and Its Application for Electrochemical Capacitor, Electrochem. Commun., 2007, 9, 569–573, DOI:10.1016/j.elecom.2006.10.027
.
- F. Su, C. K. Poh, J. S. Chen, G. Xu, D. Wang, Q. Li, J. Lin and X. W. Lou, Nitrogen-containing Microporous Carbon Nanospheres with Improved Capacitive Properties, Energy Environ. Sci., 2011, 4, 717–724, 10.1039/c0ee00277a
.
- D. Yuan, T. Zhou, S. Zhou, W. Zou, S. Mo and N. Xia, Nitrogen-enriched Carbon Nanowires from the Direct Carbonization of Polyaniline Nanowires and Its Electrochemical Properties, Electrochem. Commun., 2011, 13, 242–246, DOI:10.1016/j.elecom.2010.12.023
.
- M. Yang, B. Cheng, H. Song and X. Chen, Preparation and Electrochemical Performance of Polyaniline-based Carbon Nanotubes As Electrode Material for Supercapacitor, Electrochim. Acta, 2010, 55, 7021–7027, DOI:10.1016/j.electacta.2010.06.077
.
- P. F. Fulvio, M. Jaroniec, C. Liang and S. Dai, Polypyrrole-Based Nitrogen-Doped Carbon Replicas of SBA-15 and SBA-16 Containing Magnetic Nanoparticles, J. Phys. Chem. C, 2008, 112, 13126–13133, DOI:10.1021/jp8045164
.
- W. Kim, J. B. Joo, N. Kim, S. Oh, P. Kim and J. Yi, Preparation of Nitrogen-doped Mesoporous Carbon Nanopipes for the Electrochemical Double Layer Capacitor, Carbon, 2009, 47, 1407–1411, DOI:10.1016/j.carbon.2009.01.043
.
- G. P. Mane, S. N. Talapaneni, C. Anand, S. Varghese, H. Iwai, Q. Ji, K. Ariga, T. Mori and A. Vinu, Preparation of Highly Ordered Nitrogen-Containing Mesoporous Carbon from a Gelatin Biomolecule and Its Excellent Sensing of Acetic Acid, Adv. Funct. Mater., 2012, 22, 3596–3604, DOI:10.1002/adfm.201200207
.
- Y. Song, D. Zhou, Y. Wang, C. Wang and Y. Xia, Preparation of Nitrogen-containing Mesoporous Carbons and Their Application in Supercapacitors, New J. Chem., 2013, 37, 1768–1775, 10.1039/c3nj00261f
.
- Y. Dong, N. Nishiyama, M. Kodama, Y. Egashira and K. Ueyama, Nitrogen-containing Microporous Carbons Prepared from Anionic Surfactant-melamine/formaldehyde Composites, Carbon, 2009, 47, 2138–2141, DOI:10.1016/j.carbon.2009.04.011
.
- Y. Song, L. Li, Y. Wang, C. Wang, Z. Guo and Y. Xia, Nitrogen-Doped Ordered Mesoporous Carbon with a High Surface Area, Synthesized Through Organic-Inorganic Coassembly, and Its Application in Supercapacitors, ChemPhysChem, 2014, 15, 2084–2093, DOI:10.1002/cphc.201402250
.
- K. Kailasam, Y. Jun, P. Katekomol, J. D. Epping, W. H. Hong and A. Thomas, Mesoporous Melamine Resins by Soft Templating of Block-co-Polymer Mesophases, Chem. Mater., 2010, 22, 428–434, DOI:10.1021/cm9029903
.
- J. Yang, Y. Zhai, Y. Deng, D. Gu, Q. Li, Q. Wu, Y. Huang, B. Tu and D. Zhao, Direct Triblock-copolymer-templating Synthesis of Ordered Nitrogen-containing Mesoporous Polymers, J. Colloid Interface Sci., 2010, 342, 579–585, DOI:10.1016/j.jcis.2009.10.037
.
- Y. Wang, X. Wang, M. Antonietti and Y. Zhang, Facile One-Pot Synthesis of Nanoporous Carbon Nitride Solids by Using Soft Templates, ChemSusChem, 2010, 3, 435–439, DOI:10.1002/cssc.200900284
.
- J. Wei, D. Zhou, Z. Sun, Y. Deng, Y. Xia and D. Zhao, A Controllable Synthesis of Rich Nitrogen-Doped Ordered Mesoporous Carbon for CO Capture and Supercapacitors, Adv. Funct. Mater., 2013, 23, 2322–2328, DOI:10.1002/adfm.201202764
.
- D. Hulicova-Jurcakova, M. Kodama, S. Shiraishi, H. Hatori, Z. H. Zhu and G. Q. Lu, Nitrogen-Enriched Nonporous Carbon Electrodes with Extraordinary Supercapacitance, Adv. Funct. Mater., 2009, 19, 1800–1809, DOI:10.1002/adfm.200801100
.
- Y. Hu, H. Liu, Q. Ke and J. Wang, Effects of Nitrogen Doping on Supercapacitor Performance of a Mesoporous Carbon Electrode Produced by a Hydrothermal Soft-templating Process, J. Mater. Chem. A, 2014, 2, 11753–11758, 10.1039/c4ta01269k
.
- A. Castro-Muñiz, Y. Hoshikawa, T. Kasukabe, H. Komiyama and T. Kyotani, Real Understanding of the Nitrogen-Doping Effect on the Electrochemical Performance of Carbon Materials by Using Carbon-Coated Mesoporous Silica As a Model Material, Langmuir, 2016, 32, 2127–2135, DOI:10.1021/acs.langmuir.5b03667
.
- Q. Shi, R. Zhang, Y. Lv, Y. Deng, A. A. Elzatahrya and D. Zhao, Nitrogen-doped Ordered Mesoporous Carbons Based on Cyanamide As the Dopant for Supercapacitor, Carbon, 2015, 84, 335–346, DOI:10.1016/j.carbon.2014.12.013
.
- R. Guo, R. Lin, W. Yue and H. Ma, Enhanced Electrochemical Performance of Mesoporous Carbon with Increased Pore Size and Decreased Pore Wall Thickness, Electrochim. Acta, 2015, 174, 1050–1056, DOI:10.1016/j.electacta.2015.06.113
.
- K. Huang, Y. Yao, X. Yang, Z. Chen and M. Li, Fabrication of Flexible Hierarchical Porous Nitrogen-doped Carbon Nanofiber Films for Application in Binder-free Supercapacitors, Mater. Chem. Phys., 2016, 169, 1–5, DOI:10.1016/j.matchemphys.2015.11.024
.
- X. Huang, Q. Wang, X. Y. Chen and Z. J. Zhang, N-doped Nanoporous Carbons for the Supercapacitor Application by the Template Carbonization of Glucose: the Systematic Comparison of Different Nitridation Agents, J. Electroanal. Chem., 2015, 748, 23–33, DOI:10.1016/j.jelechem.2015.04.024
.
- B. Xu, S. Hou, F. Zhang, G. Cao, M. Chu and Y. Yang, Nitrogen-doped Mesoporous Carbon Derived from Biopolymer As Electrode Material for Supercapacitors, J. Electroanal. Chem., 2014, 712, 146–150, DOI:10.1016/j.jelechem.2013.11.020
.
- X. Yang, C. Li and R. Fu, Nitrogen-enriched Carbon with Extremely High Mesoporosity and Tunable Mesopore Size for High-performance Supercapacitors, J. Power Sources, 2016, 319, 66–72, DOI:10.1016/j.jpowsour.2016.04.037
.
- D. Qu, J. Wen, D. Liu, Z. Xie, X. Zhang, D. Zheng, J. Lei, W. Zhong, H. Tang, L. Xiao and D. Qu, Hydrogen Ion Supercapacitor: a New Hybrid Configuration of Highly Dispersed MnO in Porous Carbon Coupled with Nitrogen-Doped Highly Ordered Mesoporous Carbon with Enhanced H-Insertion, ACS Appl. Mater. Interfaces, 2014, 6, 22687–22694, DOI:10.1021/am506816b
.
- S. Shrestha, N. Morse and W. E. Mustain, Effect of Surface Chemistry on the Double Layer Capacitance of Polypyrrole-derived Ordered Mesoporous Carbon, RSC Adv., 2014, 4, 47039–47046, 10.1039/c4ra09119a
.
- T. Cai, M. Zhou, D. Ren, G. Han and S. Guan, Highly Ordered Mesoporous Phenol–formaldehyde Carbon As Supercapacitor Electrode Material, J. Power Sources, 2013, 231, 197–202, DOI:10.1016/j.jpowsour.2012.12.072
.
- J. Gao, X. Wang, Y. Zhang, J. Liu, Q. Lu and M. Liu, Boron-doped Ordered Mesoporous Carbons for the Application of Supercapacitors, Electrochim. Acta, 2016, 207, 266–274, DOI:10.1016/j.electacta.2016.05.013
.
- D. Wang, F. Li, Z. Chen, G. Q. Lu and H. Cheng, Synthesis and Electrochemical Property of Boron-Doped Mesoporous Carbon in Supercapacitor, Chem. Mater., 2008, 20, 7195–7200, DOI:10.1021/cm801729y
.
- M. Enterría, M. Pereira, J. Martins and J. Figueiredo, Hydrothermal Functionalization of Ordered Mesoporous Carbons: the Effect of Boron on Supercapacitor Performance, Carbon, 2015, 95, 72–83, DOI:10.1016/j.carbon.2015.08.009
.
- T. Panja, D. Bhattacharjya and J. Yu, Nitrogen and Phosphorus Co-doped Cubic Ordered Mesoporous Carbon As a Supercapacitor Electrode Material with Extraordinary Cyclic Stability, J. Mater. Chem. A, 2015, 3, 18001–18009, 10.1039/c5ta04169d
.
- H. Xue, T. Wang, J. Zhao, H. Gong, J. Tang, H. Guo, X. Fan and J. He, Constructing a Multicomponent Ordered Mesoporous Carbon for Improved Electrochemical Performance Induced by In-situ Doping Phosphorus, Carbon, 2016, 104, 10–19, DOI:10.1016/j.carbon.2016.03.026
.
- X. Zhao, Q. Zhang, C. Chen, B. Zhang, S. Reiche, A. Wang, T. Zhang, R. Schlögl and D. S. Su, Aromatic Sulfide, Sulfoxide, and Sulfone Mediated Mesoporous Carbon Monolith for Use in Supercapacitor, Nano Energy, 2012, 1, 624–630, DOI:10.1016/j.nanoen.2012.04.003
.
- V. G. Bairi, U. B. Nasini, S. Kumar Ramasahayam, S. E. Bourdo and T. Viswanathan, Electrocatalytic and Supercapacitor Performance of Phosphorous and Nitrogen Co-doped Porous Carbons Synthesized from Aminated Tannins, Electrochim. Acta, 2015, 182, 987–994, DOI:10.1016/j.electacta.2015.10.011
.
- U. B. Nasini, V. G. Bairi, S. Kumar Ramasahayam, S. E. Bourdo, T. Viswanathan and A. U. Shaikh, Phosphorous and Nitrogen Dual Heteroatom Doped Mesoporous Carbon Synthesized Via Microwave Method for Supercapacitor Application, J. Power Sources, 2014, 250, 257–265, DOI:10.1016/j.jpowsour.2013.11.014
.
- X. Zhao, Q. Zhang, B. Zhang, C. Chen, A. Wang, T. Zhang and D. S. Su, Dual-heteroatom-modified Ordered Mesoporous Carbon: Hydrothermal Functionalization, Structure, and Its Electrochemical Performance, J. Mater. Chem., 2012, 22, 4963–4969, 10.1039/c2jm15820e
.
- D. Zhang, Y. Hao, L. Zheng, Y. Ma, H. Feng and H. Luo, Nitrogen and Sulfur Co-doped Ordered Mesoporous Carbon with Enhanced Electrochemical Capacitance Performance, J. Mater. Chem. A, 2013, 1, 7584–7591, 10.1039/c3ta11208j
.
- J. Zhang, J. Zhou, D. Wang, L. Hou and F. Gao, Nitrogen and Sulfur Codoped Porous Carbon Microsphere: a High Performance Electrode in Supercapacitor, Electrochim. Acta, 2016, 191, 933–939, DOI:10.1016/j.electacta.2016.01.150
.
- W. Chen, J. Shi, T. Zhu, Q. Wang, J. Qiao and J. Zhang, Preparation of Nitrogen and Sulfur Dual-doped Mesoporous Carbon for Supercapacitor Electrodes with Long Cycle Stability, Electrochim. Acta, 2015, 177, 327–334, DOI:10.1016/j.electacta.2015.01.093
.
- Y. Li, G. Wang, T. Wei, Z. Fan and P. Yan, Nitrogen and Sulfur Co-doped Porous Carbon Nanosheets Derived from Willow Catkin for Supercapacitors, Nano Energy, 2016, 19, 165–175, DOI:10.1016/j.nanoen.2015.10.038
.
- D. S. Dhawale, G. P. Mane, S. Joseph, C. Anand, K. Ariga and A. Vinu, Enhanced Supercapacitor Performance of N-Doped Mesoporous Carbons Prepared from a Gelatin Biomolecule, ChemPhysChem, 2013, 14, 1563–1569, DOI:10.1002/cphc.201300132
.
- Z. Peng, D. Zhang, L. Shi and T. Yan, High Performance Ordered Mesoporous Carbon/carbon Nanotube Composite Electrodes for Capacitive Deionization, J. Mater. Chem., 2012, 22, 6603–6612, 10.1039/c2jm16735b
.
- H. Lu, W. Dai, M. Zheng, N. Li, G. Ji and J. Cao, Electrochemical Capacitive Behaviors of Ordered Mesoporous Carbons with Controllable Pore Sizes, J. Power Sources, 2012, 209, 243–250, DOI:10.1016/j.jpowsour.2012.02.041
.
- Y. Liang, D. Wu and R. Fu, Preparation and Electrochemical Performance of Novel Ordered Mesoporous Carbon with an Interconnected Channel Structure, Langmuir, 2009, 25, 7783–7785, DOI:10.1021/la9016646
.
- X. Dong, W. Shen, J. Gu, L. Xiong, Y. Zhu, H. Li and J. Shi, MnO-Embedded-in-Mesoporous-Carbon-Wall Structure for Use As Electrochemical Capacitors, J. Phys. Chem. B, 2006, 110, 6015–6019, DOI:10.1021/jp056754n
.
- L. Hao, J. Wang, L. Shen, J. Zhu, B. Ding and X. Zhang, Synthesis and Electrochemical Performances of Mixed-valence Vanadium Oxide/ordered Mesoporous Carbon Composites for Supercapacitors, RSC Adv., 2016, 6, 25056–25061, 10.1039/c5ra22520e
.
- Y. Wang, H. Li and Y. Xia, Ordered Whiskerlike Polyaniline Grown on the Surface of Mesoporous Carbon and Its Electrochemical Capacitance Performance, Adv. Mater., 2006, 18, 2619–2623, DOI:10.1002/adma.200600445
.
- Z. Zhang, G. Wang, Y. Li, X. Zhang, N. Qiao, J. Wang, J. Zhou, Z. Liu and Z. Hao, A New Type of Ordered Mesoporous Carbon/polyaniline Composites Prepared by a Two-step Nanocasting Method for High Performance Supercapacitor Applications, J. Mater. Chem. A, 2014, 2, 16715–16722, 10.1039/c4ta03351e
.
- J. Zhang, L. Kong, J. Cai, Y. Luo and L. Kang, Nano-composite of Polypyrrole/modified Mesoporous Carbon for Electrochemical Capacitor Application, Electrochim. Acta, 2010, 55, 8067–8073, DOI:10.1016/j.electacta.2010.01.052
.
- N. K. Sidhu and A. Rastogi, Electrochemical Performance of Supercapacitors Based on Carbon Nanofoam Composite and Microporous Poly(3,4-ethylenedioxythiophene) Thin Film Asymmetric Electrodes, Mater. Chem. Phys., 2016, 176, 75–86, DOI:10.1016/j.matchemphys.2016.03.028
.
- Y. Yan, Q. Cheng, V. Pavlinek, P. Saha and C. Li, Fabrication of Polyaniline/mesoporous Carbon/MnO2 Ternary Nanocomposites and Their Enhanced Electrochemical Performance for Supercapacitors, Electrochim. Acta, 2012, 71, 27–32, DOI:10.1016/j.electacta.2012.03.101
.
- J. Gao, X. Wang, Y. Zhang, J. Liu, Q. Lu, M. Chen and Y. Bai, Preparation and Supercapacitive Performance of Nanosized Manganese Dioxide/ordered Mesoporous Carbon Composites, Electrochim. Acta, 2016, 192, 234–242, DOI:10.1016/j.electacta.2016.01.205
.
- Y. Ding, J. Yang, G. Yang and P. Li, Fabrication of Ordered Mesoporous Carbons Anchored with MnO Nanoparticles Through Dual-templating Approach for Supercapacitors, Ceram. Int., 2015, 41, 9980–9987, DOI:10.1016/j.ceramint.2015.04.078
.
- D. Xiang, L. Yin, C. Wang and L. Zhang, High Electrochemical Performance of RuO2–Fe2O3 Nanoparticles Embedded Ordered Mesoporous Carbon As a Supercapacitor Electrode Material, Energy, 2016, 106, 103–111, DOI:10.1016/j.energy.2016.02.141
.
- C. Zhao, J. Li, W. Chen, Y. Yang, K. Chiang and N. Burke, Synthesis and Electrochemical Properties of Ordered Mesoporous Carbon Supported Well-dispersed Cobalt Oxide Nanoparticles for Supercapacitor, Mater. Res. Bull., 2015, 64, 55–60, DOI:10.1016/j.materresbull.2014.12.047
.
- J. Hu, M. Noked, E. Gillette, Z. Gui and S. B. Lee, Capacitance Behavior of Ordered Mesoporous Carbon/Fe2O3 Composites: Comparison Between 1D Cylindrical, 2D Hexagonal, and 3D Bicontinuous Mesostructures, Carbon, 2015, 93, 903–914, DOI:10.1016/j.carbon.2015.06.019
.
- C. Zhang, Y. Xie, M. Zhao, A. E. Pentecost, Z. Ling, J. Wang, D. Long, L. Ling and W. Qiao, Enhanced Electrochemical Performance of Hydrous RuO/Mesoporous Carbon Nanocomposites Via Nitrogen Doping, ACS Appl. Mater. Interfaces, 2014, 6, 9751–9759, DOI:10.1021/am502173x
.
- S. Sun, C. M. Ghimbeu, R. Janot, J. Le Meins, A. Cassel, C. Davoisne, C. Masquelier and C. Vix-Guterl, One-pot Synthesis of LiFePO4–carbon Mesoporous Composites for Li-ion Batteries, Microporous Mesoporous Mater., 2014, 198, 175–184, DOI:10.1016/j.micromeso.2014.07.039
.
- H. Jung, J. Shin, C. Chae, J. K. Lee and J. Kim, FeF/Ordered Mesoporous Carbon (OMC) Nanocomposites for Lithium Ion Batteries with Enhanced Electrochemical Performance, J. Phys. Chem. C, 2013, 117, 14939–14946, DOI:10.1021/jp4023162
.
- W. Zhang, X. Hou, J. Shen, S. Hu, Q. Ru and K. Lam, Magnetic PSA-Fe3O4@C 3D Mesoporous Microsphere As Anode for Lithium Ion Batteries, Electrochim. Acta, 2016, 188, 734–743, DOI:10.1016/j.electacta.2015.10.023
.
- Y. Dai, H. Jiang, Y. Hu, Y. Fu and C. Li, Controlled Synthesis of Ultrathin Hollow Mesoporous Carbon Nanospheres for Supercapacitor Applications, Ind. Eng. Chem. Res., 2014, 53, 3125–3130, DOI:10.1021/ie403950t
.
- X. Chen, K. Kierzek, Z. Jiang, H. Chen, T. Tang, M. Wojtoniszak, R. J. Kalenczuk, P. K. Chu and E. Borowiak-Palen, Synthesis, Growth Mechanism, and Electrochemical Properties of Hollow Mesoporous Carbon Spheres with Controlled Diameter, J. Phys. Chem. C, 2011, 115, 17717–17724, DOI:10.1021/jp205257u
.
- Z. Li, N. Liu, X. Wang, C. Wang, Y. Qi and L. Yin, Three-dimensional Nanohybrids of Mn3O4/ordered Mesoporous Carbons for High Performance Anode Materials for Lithium-ion Batteries, J. Mater. Chem., 2012, 22, 16640–16648, 10.1039/c2jm33195k
.
- C. Chae, J. H. Kim, J. M. Kim, Y. Sun and J. K. Lee, Highly Reversible Conversion-capacity of MnOx-loaded Ordered Mesoporous Carbon Nanorods for Lithium-ion Battery Anodes, J. Mater. Chem., 2012, 22, 17870–17877, 10.1039/c2jm32441e
.
- Z. Li, X. Wang, C. Wang and L. Yin, Hybrids of Iron Oxide/ordered Mesoporous Carbon As Anode Materials for High-capacity and High-rate Capability Lithium-ion Batteries, RSC Adv., 2013, 3, 17097–17104, 10.1039/c3ra40682b
.
- X. Wang, Z. Li and L. Yin, Nanocomposites of SnO2@ordered Mesoporous Carbon (OMC) As Anode Materials for Lithium-ion Batteries with Improved Electrochemical Performance, CrystEngComm, 2013, 15, 7589–7597, 10.1039/c3ce41256c
.
- G. Xu, S. Chen, J. Li, F. Ke, L. Huang and S. Sun, A Composite Material of SnO2/ordered Mesoporous Carbon for the Application in Lithium-ion Battery, J. Electroanal. Chem., 2011, 656, 185–191, DOI:10.1016/j.jelechem.2010.11.029
.
- Y. Liang, L. Cai, L. Chen, X. Lin, R. Fu, M. Zhang and D. Wu, Silica Nanonetwork Confined in Nitrogen-doped Ordered Mesoporous Carbon Framework for High-performance Lithium-ion Battery Anodes, Nanoscale, 2015, 7, 3971–3975, 10.1039/c4nr06611a
.
- J. Zhu, J. Yang, R. Miao, Z. Yao, X. Zhuang and X. Feng, Nitrogen-enriched, Ordered Mesoporous Carbons for Electrochemical Energy Storage, J. Mater. Chem. A, 2016, 4, 2286–2292, 10.1039/c5ta09073c
.
- Z. Wang, Y. Li and X. Lv, N-doped Ordered Mesoporous Carbon As a High Performance Anode Material in Sodium Ion Batteries at Room Temperature, RSC Adv., 2014, 4, 62673–62677, 10.1039/c4ra09084e
.
- S. Han, M. Song, H. Lee, H. Kim, H. Ahn and J. Lee, Effect of Multiwalled Carbon Nanotubes on Electrochemical Properties of Lithium/Sulfur Rechargeable Batteries, J. Electrochem. Soc., 2003, 150, A889, DOI:10.1149/1.1576766
.
- W. Zheng, Y. Liu, X. Hu and C. Zhang, Novel Nanosized Adsorbing Sulfur Composite Cathode Materials for the Advanced Secondary Lithium Batteries, Electrochim. Acta, 2006, 51, 1330–1335, DOI:10.1016/j.electacta.2005.06.021
.
- J. Shim, K. A. Striebel and E. J. Cairns, The Lithium/Sulfur Rechargeable Cell, J. Electrochem. Soc., 2002, 149, A1321, DOI:10.1149/1.1503076
.
- J. Wang, L. Liu, Z. Ling, J. Yang, C. Wan and C. Jiang, Polymer Lithium Cells with Sulfur Composites As Cathode Materials, Electrochim. Acta, 2003, 48, 1861–1867, DOI:10.1016/S0013-4686(03)00258-5
.
- M. R. Busche, P. Adelhelm, H. Sommer, H. Schneider, K. Leitner and J. Janek, Systematical Electrochemical Study on the Parasitic Shuttle-effect In lithium-sulfur-cells at Different Temperatures and Different Rates, J. Power Sources, 2014, 259, 289–299, DOI:10.1016/j.jpowsour.2014.02.075
.
- X. Ji, K. T. Lee and L. F. Nazar, A Highly Ordered Nanostructured Carbon–sulphur Cathode for Lithium–sulphur Batteries, Nat. Mater., 2009, 8, 500–506, DOI:10.1038/nmat2460
.
- G. He, X. Ji and L. Nazar, High “C” Rate Li–S Cathodes: Sulfur Imbibed Bimodal Porous Carbons, Energy Environ. Sci., 2011, 4, 2878–2883, 10.1039/c1ee01219c
.
- N. Jayaprakash, J. Shen, S. S. Moganty, A. Corona and L. A. Archer, Porous Hollow Carbon@Sulfur Composites for High-Power Lithium-Sulfur Batteries, Angew. Chem., 2011, 50, 5904–5908, DOI:10.1002/anie.201100637
.
- S. Chen, Y. Zhai, G. Xu, Y. Jiang, D. Zhao, J. Li, L. Huang and S. Sun, Ordered Mesoporous Carbon/sulfur Nanocomposite of High Performances As Cathode for Lithium–sulfur Battery, Electrochim. Acta, 2011, 56, 9549–9555, DOI:10.1016/j.electacta.2011.03.005
.
- Y. Xu, J. Wang, Z. Chang, B. Ding, Y. Wang, L. Shen, C. Mi, H. Dou and X. Zhang, Facile Synthesis of Nitrogen-Containing Mesoporous Carbon for High-Performance Energy Storage Applications, Chem. – Eur. J., 2016, 22, 4256–4262, DOI:10.1002/chem.201503917
.
- N. Moreno, A. Caballero, L. Hernán, J. Morales and J. Canales-Vázquez, Ordered Mesoporous Carbons Obtained by a Simple Soft Template Method As Sulfur Immobilizers for Lithium–sulfur Cells, Phys. Chem. Chem. Phys., 2014, 16, 17332–17340, 10.1039/c4cp02829e
.
- S. Li, T. Mou, G. Ren, J. Warzywoda, B. Wang and Z. Fan, Confining Sulfur Species in Cathodes of Lithium–Sulfur Batteries: Insight into Nonpolar and Polar Matrix Surfaces, ACS Energy Lett., 2016, 1, 481–489, DOI:10.1021/acsenergylett.6b00182
.
- S. Zhang, F. Yao, L. Yang, F. Zhang and S. Xu, Sulfur-doped Mesoporous Carbon from Surfactant-intercalated Layered Double Hydroxide Precursor As High-performance Anode Nanomaterials for Both Li-ion and Na-ion Batteries, Carbon, 2015, 93, 143–150, DOI:10.1016/j.carbon.2015.04.091
.
- S. Chen, B. Sun, X. Xie, A. K. Mondal, X. Huang and G. Wang, Multi-chambered Micro/mesoporous Carbon Nanocubes As New Polysulfides Reserviors for Lithium–sulfur Batteries with Long Cycle Life, Nano Energy, 2015, 16, 268–280, DOI:10.1016/j.nanoen.2015.05.034
.
- J. Xie, X. Yao, Q. Cheng, I. P. Madden, P. Dornath, C. Chang, W. Fan and D. Wang, Three Dimensionally Ordered Mesoporous Carbon As a Stable, High-Performance Li–O Battery Cathode, Angew. Chem., 2015, 54, 4299–4303, DOI:10.1002/anie.201410786
.
- B. Sun, H. Liu, P. Munroe, H. Ahn and G. Wang, Nanocomposites of CoO and a Mesoporous Carbon (CMK-3) As a High Performance Cathode Catalyst for Lithium–oxygen Batteries, Nano Res., 2012, 5, 460–469, DOI:10.1007/s12274-012-0231-4
.
- Z. Guo, C. Li, W. Li, H. Guo, X. Su, P. He, Y. Wang and Y. Xia, Ruthenium Oxide Coated Ordered Mesoporous Carbon Nanofiber Arrays: A Highly Bifunctional Oxygen Electrocatalyst for Rechargeable Zn–air Batteries, J. Mater. Chem. A, 2016, 4, 6282–6289, 10.1039/c6ta02030e
.
- N. L. Pocard, D. C. Alsmeyer, R. L. McCreery, T. X. Neenan and M. R. Callstrom, Nanoscale Platinum(0) Clusters in Glassy Carbon: Synthesis, Characterization, and Uncommon Catalytic Activity, J. Am. Chem. Soc., 1992, 114, 769–771, DOI:10.1021/ja00028a059
.
- H. D. Hutton, N. L. Pocard, D. C. Alsmeyer, O. J. A. Schueller, R. J. Spontak, M. E. Huston, W. Huang, R. L. McCreery, T. X. Neenan and M. R. Callstrom, Preparation of Nanoscale Platinum(0) Clusters in Glassy Carbon and Their Catalytic Activity, Chem. Mater., 1993, 5, 1727–1738, DOI:10.1021/cm00036a010
.
- S. H. Joo, S. J. Choi, I. Oh, J. Kwak, Z. Liu, O. Terasaki and R. Ryoo, Ordered Nanoporous Arrays of Carbon Supporting High Dispersions of Platinum Nanoparticles, Nature, 2001, 412, 169–172, DOI:10.1038/35084046
.
- J. Zhou, J. He, Y. Ji, W. Dang, X. Liu, G. Zhao, C. Zhang, J. Zhao, Q. Fu and H. Hu, CTAB Assisted Microwave Synthesis of Ordered Mesoporous Carbon Supported Pt Nanoparticles for Hydrogen Electro-oxidation, Electrochim. Acta, 2007, 52, 4691–4695, DOI:10.1016/j.electacta.2007.01.007
.
- L. Calvillo, M. Lázaro, E. García-Bordejé, R. Moliner, P. Cabot, I. Esparbé, E. Pastor and J. Quintana, Platinum Supported on Functionalized Ordered Mesoporous Carbon As Electrocatalyst for Direct Methanol Fuel Cells, J. Power Sources, 2007, 169, 59–64, DOI:10.1016/j.jpowsour.2007.01.042
.
- J. R. C. Salgado, J. J. Quintana, L. Calvillo, M. J. Lázaro, P. L. Cabot, I. Esparbé and E. Pastor, Carbon Monoxide and Methanol Oxidation at Platinum Catalysts Supported on Ordered Mesoporous Carbon: The Influence of Functionalization of the Support, Phys. Chem. Chem. Phys., 2008, 10, 6796–6806, 10.1039/b809227c
.
- D. Lin, Y. Jiang, S. Chen, S. Chen and S. Sun, Preparation of Pt Nanoparticles Supported on Ordered Mesoporous Carbon FDU-15 for Electrocatalytic Oxidation of CO and Methanol, Electrochim. Acta, 2012, 67, 127–132, DOI:10.1016/j.electacta.2012.02.007
.
- C. He, Y. Liang, R. Fu, D. Wu, S. Song and R. Cai, Nanopores Array of Ordered Mesoporous Carbons Determine Pt's Activity Towards Alcohol Electrooxidation, J. Mater. Chem., 2011, 21, 16357–16364, 10.1039/c1jm13423j
.
- W. C. Choi, S. I. Woo, M. K. Jeon, J. M. Sohn, M. R. Kim and H. J. Jeon, Platinum Nanoclusters Studded in the Microporous Nanowalls of Ordered Mesoporous Carbon, Adv. Mater., 2005, 17, 446–451, DOI:10.1002/adma.200400978
.
- E. P. Ambrosio, M. A. Dumitrescu, C. Francia, C. Gerbaldi and P. Spinelli, Ordered Mesoporous Carbons As Catalyst Support for PEM Fuel Cells, Fuel Cells, 2009, 9, 197–200, DOI:10.1002/fuce.200800082
.
- F. A. Viva, M. M. Bruno, M. Jobbágy and H. R. Corti, Electrochemical Characterization of PtRu Nanoparticles Supported on Mesoporous Carbon for Methanol Electrooxidation, J. Phys. Chem. C, 2012, 116, 4097–4104, DOI:10.1021/jp209549g
.
- J. Zhang, D. Liu, H. Song, Z. Liang, X. Guo, L. Du and S. Liao, Effects of Tailoring and Dehydrated Cross-linking on Morphology Evolution of Ordered Mesoporous Carbons, RSC Adv., 2016, 6, 19515–19521, 10.1039/c5ra26639d
.
- G. Z. Hu, F. Nitze, X. Jia, T. Sharifi, H. R. Barzegar, E. Gracia-Espino and T. Wågberg, Reduction Free Room Temperature Synthesis of a Durable and Efficient Pd/ordered Mesoporous Carbon Composite Electrocatalyst for Alkaline Direct Alcohols Fuel Cell, RSC Adv., 2014, 4, 676–682, 10.1039/c3ra42652a
.
- J. Zhou, J. He, T. Wang, D. Sun, G. Zhao, X. Chen, D. Wang and Z. Di, NiCl2 Assisted Synthesis of Ordered Mesoporous Carbon and a New Strategy for a Binary Catalyst, J. Mater. Chem., 2008, 18, 5776–5781, 10.1039/b811076j
.
- D. Xiang and L. Yin, Well-dispersed and Size-tuned Bimetallic PtFex Nanoparticle Catalysts Supported on Ordered Mesoporous Carbon for Enhanced Electrocatalytic Activity in Direct Methanol Fuel Cells, J. Mater. Chem., 2012, 22, 9584–9593, 10.1039/c2jm16641k
.
- H. Huang, Q. Wang, Q. Wei and Y. Huang, Nitrogen Doped Mesoporous Carbon Derived from Copolymer and Supporting Cobalt Oxide for Oxygen Reduction Reaction in Alkaline Media, Int. J. Hydrogen Energy, 2015, 40, 6072–6084, DOI:10.1016/j.ijhydene.2015.02.089
.
- X. Bo, Y. Zhang, M. Li, A. Nsabimana and L. Guo, NiCo2O4 Spinel/ordered Mesoporous Carbons As Noble-metal Free Electrocatalysts for Oxygen Reduction Reaction and the Influence of Structure of Catalyst Support on the Electrochemical Activity of NiCo2O4, J. Power Sources, 2015, 288, 1–8, DOI:10.1016/j.jpowsour.2015.04.110
.
- J. Li, Z. Li, J. Tong, C. Xia and F. Li, Nitrogen-doped Ordered Mesoporous Carbon Sphere with Short Channel As an Efficient Metal-free Catalyst for Oxygen Reduction Reaction, RSC Adv., 2015, 5, 70010–70016, 10.1039/c5ra10484j
.
- W. Yang, X. Yue, X. Liu, J. Zhai and J. Jia, IL-derived N, S Co-doped Ordered Mesoporous Carbon for High-performance Oxygen Reduction, Nanoscale, 2015, 7, 11956–11961, 10.1039/c5nr02497h
.
- S. Jiang, Y. Sun, H. Dai, J. Hu, P. Ni, Y. Wang and Z. Li, Facile Synthesis of Nitrogen and Sulfur Dual-doped Hierarchical Micro/mesoporous Carbon Foams As Efficient Metal-free Electrocatalysts for Oxygen Reduction Reaction, Electrochim. Acta, 2015, 174, 826–836, DOI:10.1016/j.electacta.2015.06.050
.
- H. Tang, Y. Zeng, D. Liu, D. Qu, J. Luo, K. Binnemans, D. E. De Vos, J. Fransaer, D. Qu and S. Sun, Dual-doped Mesoporous Carbon Synthesized by a Novel Nanocasting Method with Superior Catalytic Activity for Oxygen Reduction, Nano Energy, 2016, 26, 131–138, DOI:10.1016/j.nanoen.2016.05.015
.
- Q. Shi, Y. Lei, Y. Wang, H. Wang, L. Jiang, H. Yuan, D. Fang, B. Wang, N. Wu and Y. Gou, B,N-codoped 3D Micro-/mesoporous Carbon Nanofibers Web As Efficient Metal-free Catalysts for Oxygen Reduction, Curr. Appl. Phys., 2015, 15, 1606–1614, DOI:10.1016/j.cap.2015.09.012
.
- X. Bo and L. Guo, Ordered Mesoporous Boron-doped Carbons As Metal-free Electrocatalysts for the Oxygen Reduction Reaction in Alkaline Solution, Phys. Chem. Chem. Phys., 2013, 15, 2459–2465, 10.1039/c2cp43541a
.
- L. Wang, W. Jia, X. Liu, J. Li and M. M. Titirici, Sulphur-doped Ordered Mesoporous Carbon with Enhanced Electrocatalytic Activity for the Oxygen Reduction Reaction, J. Energy Chem., 2016, 25, 566–570, DOI:10.1016/j.jechem.2016.02.012
.
- Y. Zhou, R. Ma, S. L. Candelaria, J. Wang, Q. Liu, E. Uchaker, P. Li, Y. Chen and G. Cao, Phosphorus/sulfur Co-doped Porous Carbon with Enhanced Specific Capacitance for Supercapacitor and Improved Catalytic Activity for Oxygen Reduction Reaction, J. Power Sources, 2016, 314, 39–48, DOI:10.1016/j.jpowsour.2016.03.009
.
- V. Perazzolo, E. Grądzka, C. Durante, R. Pilot, N. Vicentini, G. A. Rizzi, G. Granozzi and A. Gennaro, Chemical and Electrochemical Stability of Nitrogen and Sulphur Doped Mesoporous Carbons, Electrochim. Acta, 2016, 197, 251–262, DOI:10.1016/j.electacta.2016.02.025
.
- P. Li, R. Ma, Y. Zhou, Y. Chen, Z. Zhou, G. Liu, Q. Liu, G. Peng, Z. Liang and J. Wang,
In Situ Growth of Spinel CoFe O Nanoparticles on Rod-like Ordered Mesoporous Carbon for Bifunctional Electrocatalysis of Both Oxygen Reduction and Oxygen Evolution, J. Mater. Chem. A, 2015, 3, 15598–15606, 10.1039/c5ta02625c
.
- T. Wang, J. Tang, X. Fan, J. Zhou, H. Xue, H. Guo and J. He, The Oriented Growth of Tungsten Oxide in Ordered Mesoporous Carbon and Their Electrochemical Performance, Nanoscale, 2014, 6, 5359–5371, 10.1039/c4nr00396a
.
- X. Zhang, J. He, T. Wang, M. Liu, H. Xue and H. Guo, Synthesis of Ordered Mesoporous Carbon Doped with Carbon Nanotubes and a New Strategy to Use It As a Support for Pt Electrocatalysts, J. Mater. Chem. A, 2014, 2, 3072–3082, 10.1039/c3ta13732e
.
- Y. Yoo, M. Kim, J. Kim, Y. S. Kim and W. Kim, Fast-response Supercapacitors with Graphitic Ordered Mesoporous Carbons and Carbon Nanotubes for AC Line Filtering, J. Mater. Chem. A, 2016, 4, 5062–5068, 10.1039/c6ta00921b
.
- A. Eftekhari and F. Molaei, Carbon Nanotube-assisted Electrodeposition. Part I: Battery Performance of Manganese Oxide Films Electrodeposited at Low Current Densities, J. Power Sources, 2015, 274, 1306–1314, DOI:10.1016/j.jpowsour.2013.10.136
.
- A. Eftekhari and F. Molaei, Carbon Nanotube-assisted Electrodeposition. Part II: Superior Pseudo-capacitive Behavior of Manganese Oxide Film Electrodeposited at High Current Densities, J. Power Sources, 2015, 274, 1315–1321, DOI:10.1016/j.jpowsour.2013.10.144
.
- M. Si, D. Feng, L. Qiu, D. Jia, A. A. Elzatahry, G. Zheng and D. Zhao, Free-standing Highly Ordered Mesoporous Carbon–silica Composite Thin Films, J. Mater. Chem. A, 2013, 1, 13490–13495, 10.1039/c3ta12925j
.
- D. Liu, D. Zheng, L. Wang, D. Qu, Z. Xie, J. Lei, L. Guo, B. Deng, L. Xiao and D. Qu, Enhancement of Electrochemical Hydrogen Insertion in N-Doped Highly Ordered Mesoporous Carbon, J. Phys. Chem. C, 2014, 118, 2370–2374, DOI:10.1021/jp412099y
.
- S. Giraudet, Z. Zhu, X. Yao and G. Lu, Ordered Mesoporous Carbons Enriched with Nitrogen: Application to Hydrogen Storage, J. Phys. Chem. C, 2010, 114, 8639–8645, DOI:10.1021/jp101119r
.
- N. Liu, L. Yin, L. Kang, X. Zhao, C. Wang, L. Zhang, D. Xiang, R. Gao, Y. Qi and N. Lun, Enhanced Electrochemical Hydrogen Storage Capacity of Activated Mesoporous Carbon Materials Containing Nickel Inclusions, Int. J. Hydrogen Energy, 2010, 35, 12410–12420, DOI:10.1016/j.ijhydene.2010.08.099
.
- D. Xiang, R. Tang, Q. Su and L. Yin, Crystalline NiFex Nanoparticles Homogeneously Embedded in Ordered Mesoporous Carbon for Improved Electrochemical Hydrogen Storage Performance, CrystEngComm, 2013, 15, 5442–5451, 10.1039/c3ce40369f
.
- A. Kong, W. Wang, F. Yang, H. Ding and Y. Shan, In Situ Synthesis of N and Cu Functionalized Mesoporous FDU-14 Resins and Carbons for Electrochemical Hydrogen Storage, Int. J. Hydrogen Energy, 2010, 35, 7530–7538, DOI:10.1016/j.ijhydene.2010.04.164
.
|
This journal is © the Partner Organisations 2017 |
Click here to see how this site uses Cookies. View our privacy policy here.