DOI:
10.1039/C5QI00249D
(Research Article)
Inorg. Chem. Front., 2016,
3, 320-325
Mixed-anion templated cage-like lanthanide clusters: Gd27 and Dy27†
Received
12th November 2015
, Accepted 13th December 2015
First published on 21st December 2015
Abstract
Two nanoscale clusters whose metals are exclusively lanthanides, and whose formulas are [(ClO4)@Ln27(μ3-OH)32(CO3)8(CH3CH2COO)20(H2O)40]·(ClO4)12·(H2O)50 (abbreviated as Ln27. 1, Gd; 2, Dy), were synthesized. Structural analysis showed that the 27 lanthanide ions were organized into a beautiful cage-like structure templated by eight CO32− groups, which were generated from the fixation of atmospheric CO2. Acting as a template guest, one ClO4− group was encapsulated in the Ln27 cage. The Ln27 compound is by far the largest odd-numbered lanthanide cluster synthesized to date. In magnetization studies of these two compounds, isotropic Gd27 exhibited a large MCE of 41.8 J kg−1 k−1 at 2 K for ΔH = 7 T, while anisotropic Dy27 displayed a slow relaxation of its magnetization.
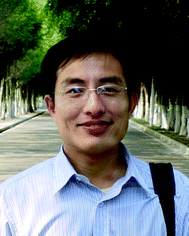 Xiang-Jian Kong | Xiang-Jian Kong received his B.S. degree in chemistry from Liaocheng University in 2003 and his Ph.D. from Xiamen University in 2009. He was a visiting scholar at The University of Arizona (2007–2008) and The University of Chicago (2014–2015). Currently he is an associate professor at Xiamen University. His research interests are in the field of synthetic and materials chemistry of lanthanide clusters and lanthanide-transition heterometallic clusters. |
Introduction
Polynuclear metal clusters whose metals are exclusively lanthanides have become the subjects of one of the intriguing research topics in current chemical studies, because of their interesting magnetic properties such as their single-molecule magnetism (SMM) and large magnetocaloric effects (MCEs).1–5 Although the rational syntheses of many lanthanide clusters via a ligand-controlled hydrolytic approach have been reported, the design and synthesis of high-nuclearity lanthanide clusters still remain a challenge because of the repulsion between the highly positive charges of the metal ions.6–10
Some high-nuclearity, exclusively lanthanide clusters including more than 20 metal ions have been reported, with such clusters including Ln22,11 Ln24,12 Ln26,13 Ln28,14 Ln36,15 Ln38,16a Ln48,16 Ln60,17 and Ln104.18 These reported high-nuclearity clusters show two interesting features: they are all even-numbered clusters, which may be due to the lack of crystallographic odd-number-fold rotational symmetries(>3);19 and they all, except for Ce22, have been assembled under templating anions, perhaps not only because the anions can act as templates to induce the formation of the cluster skeletons, but also because they can serve as negative charges to balance the positive charges of metal ions.
Recently, we reported the synthesis of Ln104, one of the largest lanthanide clusters ever made, by controlling the hydrolysis of Ln3+ ions in the presence of a simple acetate ligand. Ancillary ligands or the substituent groups of these ligands are known to have important effects on the structure of the metal cluster core. As a continuation of our work on lanthanide clusters, we selected propionate ligands to study their steric effects on the nuclearity of metal clusters. Herein, two nanoscale lanthanide clusters with the formulas [(ClO4)@Ln27(μ3-OH)32(CO3)8(CH3CH2COO)20(H2O)40](ClO4)12·(H2O)50 (1: Gd, 2: Dy) were synthesized. Structural analysis showed that the 27 lanthanide ions were organized into a beautiful cage-like structure templated by eight CO32− and one ClO4− groups. Notably, the Ln27 compound is by far the largest odd-numbered lanthanide cluster synthesized to date.
Experimental
Materials and physical measurements
Caution! Perchlorates are potentially explosive. Only a small amount should be used, and they should be handled with great care. In our experiments, all reagents were of commercial origin and were used as received. Aqueous solutions of lanthanide perchlorates were prepared by digesting lanthanide oxides in concentrated perchloric acid. A suitable concentration was achieved by diluting the concentrated solution with deionized water. Microanalyses of C, H, and N were carried out with a CE Instruments EA 1110 elemental analyser. An infrared spectrum was recorded on a Nicolet AVATAR FT-IR360 spectrophotometer with pressed KBr pellets. Thermogravimetric analysis (TGA) curve was generated by using a SDT Q600 thermal analyzer. Magnetic susceptibility was measured by using a Quantum Design MPMS superconducting quantum interference device (SQUID).
Preparation of aqueous solutions of Ln(ClO4)3 (1.0 mol L−1)
An aqueous solution of perchloric acid (70.0%–72.0%, 64.0 mL) was slowly added to gadolinium oxide (0.125 mol, 45.313 g) at about 80 °C to form a concentrated solution. A 1.0 mol L−1 aqueous solution of Gd(ClO4)3 was obtained by diluting the concentrated solution to a volume of 250.00 mL with deionized water. Dy(ClO4)3 (1.0 mol L−1) was prepared following the preceding procedure, but using dysprosium oxide (0.125 mol, 46.625 g) instead of gadolinium oxide.
Syntheses of compounds 1 and 2
[ClO4@Gd27(μ3-OH)32(CO3)8(CH3CH2COO)20(H2O)40] (ClO4)12·(H2O)50 (1).
Sodium propionate (0.096 g, 1.0 mmol) and Gd(ClO4)3 (3 mL, 3.0 mmol) were added to a mixture of 10 mL anhydrous ethanol and 3 mL deionized water. The resulting solution was heated to about 80 °C and a freshly prepared NaOH solution (aq. 1.0 M) was added dropwise to the point of an incipient but permanent precipitation. The mixture was then stirred under reflux for two hours. The resulting solution was filtered while hot. Evaporation of the filtrate under ambient conditions afforded colorless crystals (yield 30% based on Gd). C68H310Cl13Gd27O247 (FW = 9787.76): C: 8.34; H: 3.19. Found: C: 7.97 H: 2.92. IR (KBr, cm−1): 2978 (w), 1555 (s), 1430 (m), 1300 (s), 1245 (w), 1084 (s), 936 (w), 891 (w), 630 (s).
[ClO4@Dy27(μ3-OH)32(CO3)8(CH3CH2COO)20(H2O)40] (ClO4)12·(H2O)50 (2).
Compound 2 was prepared using the same procedure as described for the synthesis of 1 but using Dy(ClO4)3 instead of Gd(ClO4)3. Anal. Calcd For C68H310Cl13Dy27O247 (FW = 9929.43): C: 8.22; H: 3.14. Found: C: 7.87; H: 2.87. IR (KBr, cm−1): 2978 (w), 1555 (s), 1430 (m), 1300 (s), 1245 (w), 1084 (s), 936 (w), 891 (w), 630 (s).
X-ray crystallography
X-ray diffraction data of compounds 1 and 2 were collected on an Oxford Gemini S Ultra CCD area detector with monochromatic Mo Kα radiation (λ = 0.71073 Å). Absorption corrections were applied by using the multi-scan program CrysAlis Red.20 The structures were solved by direct methods, and non-hydrogen atoms were refined anisotropically by least-squares on F2 using the SHELXTL-97 program.21 The hydrogen atoms of the organic ligand were generated geometrically (C–H, 0.96 A). Crystal data as well as details of data collection and refinement for the complexes are summarized in Table S1.† Selected bond lengths and bond angles are given in Tables S2 and S3.† Balancing the charge led to 12ClO4− counter anions per formula unit. EA and TGA suggested the presence of ca. 50 guest water molecules per formula unit.22 The Cambridge Crystallographic Data Centre (CCDC) contains the supplementary crystallographic data for this paper with a deposition number of 1435437–1435438 for 1 and 2.
Results and discussion
Synthesis
Recently, we reported the syntheses of two Ln104 complexes that were accomplished by controlling the hydrolysis of Ln3+ ions in the presence of a simple acetate ligand.18 Because of steric effects, different ligands can change the structure of the cluster. In this work, we selected the large propionate ligand to control the hydrolysis of Ln3+ ions, in order to study the steric effects of different ligands on the cluster structure.9 Herein, we obtained two lanthanide clusters, each containing 27 lanthanide ions.
In these two Ln27 structures, eight CO32− groups and one ClO4− group together played an important template role in the assembly of the cage-like high-nuclearity clusters. The use of a simple single anion as a template has been studied most extensively. However, compared with single anions, the use of a mixture of anions as the template would be a better approach to construct high-nuclearity 4f metal clusters because of the multiple anions template disperse the positive charges of metal ions.
The carbonate anion is a very common anion template for 4f clusters, because of its versatile coordination modes and the relatively strong coordination-type bonds it makes with lanthanide ions. However, because of the insolubility of the lanthanide carbonate, only a few carbonate-templated 4f clusters have been reported. To avoid the lanthanide carbonate precipitate, carbonate has been introduced in a few special ways. First, ligand decomposition has been used as an efficient method to slowly release carbonate ions. For example, we reported work on an Er60 cluster featuring 8 μ6-CO32− ions, which probably derived from the decomposition of threonine.17 Second, fixation of atmospheric CO2 in a basic medium is another efficient way to slowly introduce the carbonate.23 Some research groups have found that bubbling CO2 through a reaction solution is also an efficient way to slowly introduce the CO32− group.24 In addition, by adding Na2CO3 or NaHCO3 deliberately, the yield of a serendipitously obtained product can be obviously improved.
Crystal structures
Single crystal X-ray diffraction revealed compounds 1 and 2 to be iso-structural. As a representative example, only the structure of 1 is discussed here to illustrate the structural features of both compounds. Compound 1 was determined to consist of one cationic cluster of [ClO4−@Ln27(μ3-OH)32(CO3)8(CH3CH2COO)20(H2O)40]12+ (Fig. 1), 12ClO4− groups and about 50 guest water molecules.
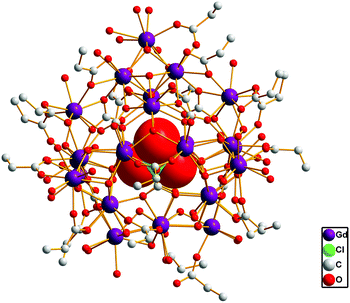 |
| Fig. 1 Ball-and-stick view of the cationic cluster [ClO4−@Ln27(μ3-OH)32(CO3)8(CH3CH2COO)20(H2O)40]13+. | |
The cationic cluster core of compound 1 can be viewed as being constructed from a cage-like core [Gd27(μ3-OH)32(CO3)8]33+ protected and stabilized by 20 propionate ligands and 40 water molecules, and encapsulating a ClO4− anion. As shown in Fig. 2, 27 Gd3+ ions were observed to be organized into three types of cluster units: [Gd4(μ3-OH)4]8+ (type I), [Gd4(μ3-OH)7]5+ (type II), and [Gd7(μ3-OH)5(CO3)4]8+ (type III) units. Type I [Gd4(μ3-OH)4]8+ was found to be a cubane-like structural unit (Fig. 2a), which is the most common unit in lanthanide clusters. The four Gd3+ atoms formed a nearly perfect tetrahedron with each face capped by one μ3-OH ligand. Four type I units were observed to be connected together by four CO32− anions, forming a ring-like [Gd16(μ3-OH)16(CO3)4]24+ structure (Fig. 2d). The type II structure, formulated as [Gd4(μ3-OH)7]5+, was found to be a planar square structure, in which four Gd3+ ions were observed to be linked by three OH− groups in a μ3-OH fashion and four OH− groups in a μ2-OH fashion (Fig. 2b). As shown in Fig. 2c, type III [Gd7(μ3-OH)5(CO3)4]8+ is composed of one cubane-shaped [Gd4(μ3-OH)4]4+ unit and three Gd3+ ions through four bridging CO32− anions and one μ3-OH. The ring-like [Gd16(μ3-OH)16(CO3)4]24+ and one type II structure were joined together by four CO32− groups, generating a bowl-like [Gd20(μ3-OH)23(CO3)4]29+ unit (Fig. 2e). The bowl-like unit was seen to be further capped with the type III unit, resulting in the [Gd27(μ3-OH)32(CO3)8]33+ core (Fig. 2f). One ClO4− anion, acting as a template by being hydrogen-bonded to four μ3-OH− groups along the inner cavity, was observed to be encapsulated in a cage-like cavity. Although the ClO4− ion and CO32− ion acting as mixed templates have been reported in the 3d–4f clusters, the ClO4− ion and CO32− ion synergetically templating an exclusively lanthanide cluster has not been reported.
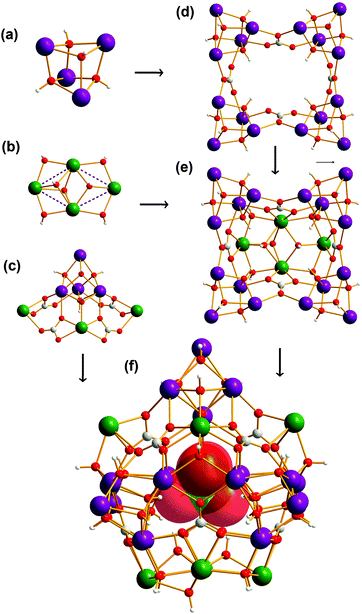 |
| Fig. 2 (a) Ball-and-stick views of type I cubane-like [Gd4(μ3-OH)4]8+, (b) the type II [Gd4(μ3-OH)7]5+ unit, (c) the type III [Gd7(μ3-OH)5(CO3)4]8+ unit, (d) the [Gd16(μ3-OH)16(CO3)4]24+ unit, (e) the [Gd20(μ3-OH)23(CO3)4]29+ unit, and (f) the [ClO4−@Gd27(μ3-OH)32(CO3)8]32+ cluster core. | |
The metal skeleton of the Gd27 cluster is shown in Fig. 3a. Five tetrahedra (20 Gd3+) and one quadrangle (4 Gd3+) were observed to be linked by three metal ions, generating the framework of the cage cluster. The inner cage containing 22 Gd3+ ions was found to consist of 8 pentagonal and 16 triangular faces, as shown in Fig. 3b. The 8 pentagonal faces were templated by 8 CO32− anions.
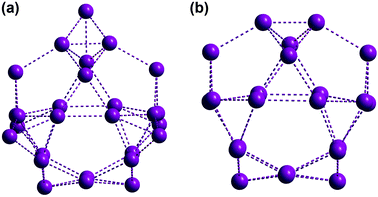 |
| Fig. 3 (a) Ball-and-stick views of the metal skeleton of Gd27. (b) The inner cage structure containing 22 Gd3+ ions with 8 pentagonal and 16 triangular faces. | |
The ranges of the Gd–O bond lengths and the Gd⋯Gd distances were measured to be 2.148–2.939 Å and 3.5229–3.9915 Å, respectively, which are comparable to the corresponding values in the previously reported Gd cluster.18 Compound 2 was found to be isostructural to 1, with the main differences being the bond lengths and angles, with the ranges of the Dy–O bond lengths and Dy⋯Dy distances being 2.202–2.904 Å and 3.4681–3.9475 Å, respectively.
Magnetic properties
The temperature dependence of the magnetic susceptibilities of 1 and 2 were measured between 2 K and 300 K with an applied direct-current (dc) magnetic field of 1000 Oe. The plots of χMT and χM−1vs. T are displayed in Fig. 4a. The χMT values of 217.22 cm3 K mol−1 for 1 and 357.08 cm3 K mol−1 for 2 at 300 K are close to the calculated spin-only values of 212.63 cm3 K mol−1 for 27 uncorrelated Gd3+ ions (S = 7/2, g = 2) and 382.50 cm3 K mol−1 for 27 uncorrelated Dy3+ ions (S = 5/2, g = 4/3) respectively. For 1, the χMT value slightly decreased upon lowering the temperature to 50 K, and then sharply decreased to a minimum value of 116.92 cm3 K mol−1 at 2 K, suggesting the presence of dominant antiferromagnetic interactions between the Gd3+ ions.25 To estimate the intramolecular exchange constant, fitting the curve of 1/χMvs. T in the range of 2–300 K yielded C = 219.3 cm3 K mol−1 and θ = −3.0 K. For 2, upon cooling, the χMT values slowly decreased at first, and then decreased rapidly to a minimum value of 206.3 cm3 K mol−1 at 2 K. The profile of the χMT value versus T may be attributed to the presence of strong spin–orbit coupling and the thermal depopulation of excited Stark sublevels of Dy3+.5c,26
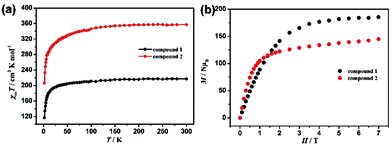 |
| Fig. 4 (a) Plots of the temperature dependence of χMT for Gd27 and Dy27 under Hdc = 1000 Oe between 2 and 300 K. (b) Magnetization versus H/T for Gd27 and Dy27 at 2.0 K and at indicated fields. | |
Measurements of the field (H) dependence of the magnetizations (M) of Gd27 and Dy27 in the range of 0–7 T at low temperature (2–10 K) were also taken (Fig. 4b). The magnetization value rapidly increased for Gd27 and Dy27 as the magnetic field was decreased. The magnetization value for Gd27 was measured to be as high as 185.3NμB, which is its magnetization saturation level and is consistent with the theoretical value 189NμB. But for Dy27, the M value only increased slightly, and peaked at a sub-saturation value of 144.7NμB at 2 K and 7 T. The lack of saturation may have resulted from the strong magnetic anisotropy or low-lying excited states of Dy3+.27
High-nuclearity Gd clusters are usually associated with large changes in entropy, and such clusters are very good candidates for magnetic coolers because of the isotropy of Gd3+ ion and the high magnetic density of the high-nuclearity Gd cluster. Therefore, the MCE of Gd27 was studied using the Maxwell equation
.28 As shown in Fig. 5, the value of S for Gd27 was determined to be 41.8 J kg−1 K−1 at 2 K for ΔH = 7 T. This value is smaller than the 56.1 R value calculated for the spins of 27 uncorrelated Gd3+ ions (S = 7/2) using the equation −ΔSm = nR
ln(2S + 1), where R is the gas constant and S is the spin state.28 Due to its high density of 2.878 g cm−3, the Gd27 cluster was also found to display a very large volumetric magnetic entropy change of 120.4 mJ cm−3 K−1, which is comparable to the largest value attained to date for any lanthanide clusters (Table S6†).
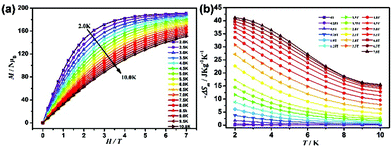 |
| Fig. 5 For 1: (a) The magnetization versus the dc field in the temperature range of 2–10 K; (b) The temperature dependencies of −ΔSm for selected ΔH values obtained from the magnetization measurements. | |
In view of the significant anisotropy inherent in Dy3+, the temperature dependence of the alternating-current (ac) magnetic susceptibility was also measured to probe the dynamics of Dy27 magnetization. Such measurements were carried out in a zero dc field for a temperature range of 2.0–10.0 K and between 10 and 1500 Hz, and the plots of χ′ and χ′′ vs. T are given in Fig. S5.† Due to fast quantum tunneling of the magnetization, the maximum of χ′′ for 2 was not observed in the test temperature range and the energy barrier could not be obtained from fitting the Arrhenius expression. But if it is assumed that there is only a kind of characteristic relaxation for the compound, the energy barrier and the relaxation time can be roughly estimated from the slope and the intercept of fitting the ac data by using the Debye model and the equation ln(χ′′/χ′) = ln(ωτ0) + Ea/kBT.29,30 The calculated values are τ0 = 3.55 × 10−7 s and Ea = 3.81 K.
Conclusions
In summary, two cage-like odd-numbered purely lanthanide-clusters of Gd27 and Dy27 were synthesized by controlling the hydrolysis of Ln3+ ions in the presence of a simple propionate ligand. Structural analysis showed that 27 lanthanide ions were organized into a cage structure templated by one ClO4− and eight CO32− anions. Eight CO32− anions supported eight pentagonal faces and sixteen triangular faces that together formed the inner cage metal skeleton. Magnetization studies indicated that Gd27 displays large changes in entropy with changing magnetic field. This work suggests that a mixed-anion template is an effective synthetic method to construct high-nuclearity lanthanide clusters with novel structures. The preparation of other high-nuclearity lanthanide clusters utilizing the mixed-anion template strategy is in progress.
Acknowledgements
This work was supported by the 973 project (Grants nos. 2012CB821704 and 2014CB845601) from the Ministry of Science and Technology of China, the National Natural Science Foundation of China (Grants No. 21422106, 21371144, 21431005, and 21390391), and the Foundation for the Author of National Excellent Doctoral Dissertation of PR China (201219) for financial support. We thank X. R. Wu for experimental help and discussions.
Notes and references
-
(a) R. E. P. Winpenny, Chem. Soc. Rev., 1998, 27, 447 RSC;
(b) J. W. Sharples and D. Collison, Coord. Chem. Rev., 2014, 260, 1 CrossRef CAS PubMed;
(c) Y. G. Huang, F. L. Jiang and M. C. Hong, Coord. Chem. Rev., 2009, 253, 2814 CrossRef CAS.
-
(a) K. Liu, W. Shi and P. Cheng, Coord. Chem. Rev., 2015, 74, 289 Search PubMed;
(b) J. C. G. Bünzli and C. Piguet, Chem. Soc. Rev., 2005, 34, 1048 RSC.
-
(a) T. Kajiwara, N. Iki and M. Yamashita, Coord. Chem. Rev., 2007, 251, 1734 CrossRef CAS;
(b) X. J. Kong, L. S. Long, Z. P. Zheng, R. B. Huang and L. S. Zheng, Acc. Chem. Res., 2010, 43, 201 CrossRef CAS PubMed;
(c) Y. N. Guo, G. F. Xu, P. Gamez, L. Zhao, Sh. Y. Lin, R. Deng, J. K. Tang and H. J. Zhang, J. Am. Chem. Soc., 2010, 132, 8538 CrossRef CAS PubMed.
-
(a) Y. Z. Zheng, G. J. Zhou, Z. Zheng and R. E. Winpenny, Chem. Soc. Rev., 2014, 43, 1462 RSC;
(b) D. I. Alexandropoulos, S. Mukherjee, C. Papatriantafyllopoulou, C. P. Raptopoulou, V. Psycharis, V. Bekiari, G. Christou and T. C. Stamatatos, Inorg. Chem., 2011, 50, 11276 CrossRef CAS PubMed;
(c) P. H. Lin, W. B. Sun, M. F. Yu, G. M. Li, P. F. Yan and M. Murugesu, Chem. Commun., 2011, 47, 10993 RSC.
-
(a) P. W. Roesky, G. Canseco-Melchor and A. Zulys, Chem. Commun., 2004, 738 RSC;
(b) J. W. Sharples, Y. Z. Zheng, F. Tuna, E. J. McInnes and D. Collison, Chem. Commun., 2011, 47, 7650 RSC;
(c) S. D. Han, X. H. Miao, S. J. Liu and X. H. Bu, Inorg. Chem. Front., 2014, 1, 549 RSC;
(d) S. D. Han, X. H. Miao, S. J. Liu and X. H. Bu, Chem. – Asian J., 2014, 9, 3116 CrossRef CAS PubMed;
(e) S. J. Liu, J. P. Zhao, J. Tao, J. M. Jia, S. D. Han, Y. Li, Y. C. Chen and X. H. Bu, Inorg. Chem., 2013, 52, 9163 CrossRef CAS PubMed.
-
(a) R. Wang, M. D. Carducci and Z. Zheng, Inorg. Chem., 2000, 39, 1836 CrossRef CAS PubMed;
(b) R. Wang, D. Song and S. Wang, Chem. Commun., 2002, 368 RSC;
(c) K. H. Zangana, E. M. Pineda, E. J. L. McInnes, J. Schnack and R. E. P. Winpenny, Chem. Commun., 2014, 50, 1438 RSC.
-
(a) J. B. Peng, X. J. Kong, Y. P. Ren, L. S. Long, R. B. Huang and L. S. Zheng, Inorg. Chem., 2012, 51, 2186 CrossRef CAS PubMed;
(b) V. Baskar and P. W. Roesky, Dalton Trans., 2006, 676 RSC.
-
(a) M. R. Brügstein, M. T. Gamer and P. W. Roesky, J. Am. Chem. Soc., 2004, 126, 5213 CrossRef PubMed;
(b) Y. C. Chen, F. S. Guo, Y. Z. Zheng, J. L. Liu, J. D. Leng, R. Tarasenko, M. Orendáč, J. Prokleška, V. Sechovský and M. L. Tong, Chem. – Eur. J, 2013, 19, 13504 CrossRef CAS PubMed.
- D. D'Alessio, A. N. Sobolev, B. W. Skelton, R. O. Fuller, R. C. Woodward, N. A. Lengkeek, B. H. Fraser, M. Massi and M. I. Ogden, J. Am. Chem. Soc., 2014, 136, 15122 CrossRef PubMed.
- J. M. Jia, S. J. Liu, Y. Cui, S. D. Han, T. L. Hu and X. H. Bu, Cryst. Growth. Des., 2013, 13, 4631 CAS.
- I. L. Malaestean, A. Ellern, S. Baca and P. Kogerler, Chem. Commun., 2012, 48, 1499 RSC.
- L. X. Chang, G. Xiong, L. Wang, P. Cheng and B. Zhao, Chem. Commun., 2013, 49, 1055 RSC.
-
(a) X. J. Gu and D. F. Xue, Inorg. Chem., 2007, 46, 3212 CrossRef CAS PubMed;
(b) X. Gu, R. Clérac, A. Houri and D. Xue, Inorg. Chim. Acta, 2008, 361, 3873 CrossRef CAS.
- M. Romanelli, G. A. Kumar, T. J. Emge, R. E. Riman and J. G. Brennan, Angew. Chem., Int. Ed., 2008, 47, 6049 CrossRef CAS PubMed.
- M. Y. Wu, F. L. Jiang, X. J. Kong, D. Q. Yuan, L. S. Long, S. A. Al-Thabaiti and M. C. Hong, Chem. Sci., 2013, 4, 3104 RSC.
-
(a) F. S. Guo, Y. C. Chen, L. L. Mao, W. Q. Lin, J. D. Leng, R. Tarasenko, M. Orendac, J. Prokleska, V. Sechovsky and M. L. Tong, Chem. – Eur. J., 2013, 19, 14876 CrossRef CAS PubMed;
(b) M. Wu, F. Jiang, D. Yuan, J. Pang, J. Qian, S. A. Al-Thabaiti and M. Hong, Chem. Commun., 2014, 1113 RSC;
(c) L. Chen, J. Y. Guo, X. Xu, W. W. Ju, D. Zhang, D. R. Zhu and Y. Xu, Chem. Commun., 2013, 49, 9728 RSC.
- X. J. Kong, Y. L. Wu, L. S. Long, L. S. Zheng and Z. P. Zheng, J. Am. Chem. Soc., 2009, 131, 6918 CrossRef CAS PubMed.
- J. B. Peng, X. J. Kong, Q. C. Zhang, M. Orendac, J. Prokleska, Y. P. Ren, L. S. Long, Z. Zheng and L. S. Zheng, J. Am. Chem. Soc., 2014, 136, 17938 CrossRef CAS PubMed.
-
(a) N. Hoshino, M. Nakano, H. Nojiri, W. Wernsdorfer and H. Oshio, J. Am. Chem. Soc., 2009, 131, 15100 CrossRef CAS PubMed;
(b) M. L. Baker, G. A. Timco, S. Piligkos, J. Mathieson, H. Mutka, F. Tuna, P. Kozłowski, M. Antkowiak, T. Guidi, T. Gupta, H. Rath, R. J. Woolfson, G. Kamieniarz, R. G. Pritchard, H. Weihe, L. Cronin, G. Rajaraman, D. Collison, E. J. L. McInnes and R. E. P. Winpenny, Proc. Natl. Acad. Sci. U. S. A., 2012, 109, 19113 CrossRef CAS PubMed.
-
Oxford Diffraction 2008, CrysAlis CCD and CrysAlis RED, version 1.171.32.15, Oxford Diffraction Ltd, Abingdon, Oxford, England Search PubMed.
-
(a) G. M. Sheldrick, Acta Crystallogr., Sect. A: Fundam. Crystallogr., 2008, 64, 112 CrossRef CAS PubMed;
(b)
SHELXTL 6.10, Bruker Analytical Instrumentation, Madison, WI, 2000 Search PubMed.
- L. J. Farrugia, J. Appl. Crystallogr., 1999, 32, 837 CrossRef CAS.
- B. Cristovao, B. Miroslaw, J. Klak and M. Rams, Polyhedron, 2015, 85, 697 CrossRef CAS.
- T. N. Hooper, R. Inglis, M. A. Palacios, G. S. Nichol, M. B. Pitak, S. J. Coles, G. Lorusso, M. Evangelisti and E. K. Brechin, Chem. Commun., 2014, 50, 3498 RSC.
- F. S. Guo, J. D. Leng, J. L. Liu, Z. S. Meng and M. L. Tong, Inorg. Chem., 2011, 51, 405 CrossRef PubMed.
-
(a) V. Chandrasekhar, S. Das, A. Dey, S. Hossain and J.-P. Sutter, Inorg. Chem., 2013, 52, 11956 CrossRef CAS PubMed;
(b) Y. N. Guo, G. F. Xu, P. Gamez, L. Zhao, S. Y. Lin, R. Deng, J. Tang and H. J. Zhang, J. Am. Chem. Soc., 2010, 132, 8538 CrossRef CAS PubMed;
(c) Y. L. Hou, G. Xiong, P. F. Shi, R. R. Cheng, J. Z. Cui and B. Zhao, Chem. Commun., 2013, 49, 6066 RSC.
-
(a) Y. Z. Zheng, M. Evangelisti, F. Tuna and R. E. Winpenny, J. Am. Chem. Soc., 2012, 134, 1057 CrossRef CAS PubMed;
(b) S. K. Langley, N. F. Chilton, B. Moubaraki and K. S. Murray, Inorg. Chem., 2013, 52, 7183 CrossRef CAS PubMed.
-
(a) M. Evangelisti and E. K. Brechin, Dalton Trans., 2010, 39, 4672 RSC;
(b) M. Evangelisti, F. Luis, L. De Jongh and M. Affronte, J. Mater. Chem., 2006, 16, 2534 RSC.
- Y. L. Miao, J. L. Liu, J. D. Leng, Z. J. Lin and M. L. Tong, CrystEngComm, 2011, 13, 3345 RSC.
-
(a) K. S. Cole and R. H. Cole, J. Chem. Phys., 1941, 9, 341 CrossRef CAS;
(b) S. Y. Lin, G. F. Xu, L. Zhao, Y. N. Guo, Y. Guo and J. Tang, Dalton Trans., 2011, 40, 8213 RSC.
Footnote |
† Electronic supplementary information (ESI) available: Tables S1–S4, and Fig. S1–S3. CCDC 1435437 and 1435438. For ESI and crystallographic data in CIF or other electronic format see DOI: 10.1039/c5qi00249d |
|
This journal is © the Partner Organisations 2016 |
Click here to see how this site uses Cookies. View our privacy policy here.