DOI:
10.1039/D0AN02110E
(Minireview)
Analyst, 2021,
146, 803-815
Progress in electrochemiluminescence of nanoclusters: how to improve the quantum yield of nanoclusters
Received
24th October 2020
, Accepted 10th December 2020
First published on 11th December 2020
Abstract
Luminescent nanoclusters (NCs), with their easy preparation, ultrafine size, low toxicity, and excellent photostability have recently emerged as novel electrochemiluminescence (ECL) emitters. However, relatively low quantum yield (QY) in both aqueous and organic media has impeded their application in ECL emitter evolution. In this mini-review, we discuss the recent development of NCs in ECL with particular focus on their optical properties. We first classify the NCs according to composition and structure, and then summarize four aspects that affect QY, including environment effects, construction, valence state effects and aggregation-induced ECL. The ECL mechanisms based on NCs are elucidated as well. Finally, we briefly discuss the potential applications of NCs in tumor markers test, immunoassay and serum test. This review aims to provide a comprehensive summary of the progress of NCs in ECL, which will motivate researchers to develop NC chemistry and explore their future applications in ECL.
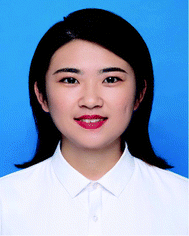 Junjun Ge | Junjun Ge received her B.S. and M.S. degrees from Qingdao University of Science and Technology in 2017 and in 2020, respectively. Currently, she is a Ph.D. candidate in Chemistry under the supervision of Prof. Yuanyuan Wang at Nanjing University. Her research focuses on the synthesis and characterization of semiconductor nanoclusters and optimization of their ECL performance. |
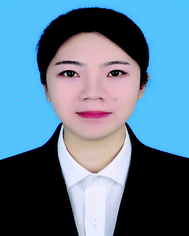 Xufeng Chen | Xufeng Chen received her B.S. degree from Nanjing Tech University in 2018 and is an M.S. degree student in Prof. Yuanyuan Wang's research group. Her research interests include self-assemblies and surface design of semiconductor nanoclusters. |
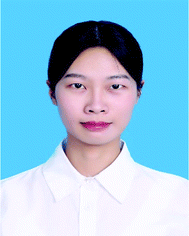 Jinling Yang | Jinling Yang received her B.Sc. degree from Chongqing University in 2019, and is an M.S. degree student in Prof. Yuanyuan Wang's research group at Nanjing University. Her research focuses on the synthesis and surface modification of metal nanoclusters. |
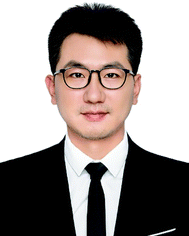 Yuanyuan Wang | Yuanyuan Wang is a Professor in the School of Chemistry and Chemical Engineering at Nanjing University. He received his B.S. (2006) and M.S. (2009) in Analytical Chemistry from Nanjing University and his Ph.D. (2014) in Inorganic Chemistry from Washington University in St. Louis. Before joining Nanjing University in 2019, Dr Wang worked as a postdoctoral fellow at the James Frank Institute of the University of Chicago. His research interests include nanocluster synthesis, nanocrystal assembly and micro- and nanocrystal-based device fabrication. |
1. Introduction
Electrochemiluminescence (ECL) has undergone more than 50 years of development since D. M. Hercules detailed and discussed the mechanism for the first time in 1964.1 As a radiative process, ECL measurement relies on the interactions between reactive radical species that are electrochemically produced around the working electrodes.2–7 Unlike conventional optical techniques, such as fluorescence,8 photoelectrochemical technology,9 surface plasmon resonance10 or surface-enhanced Raman scattering,11 ECL technology does not require an external extrinsic illumination source and exhibits greatly reduced background interference. Apart from this, the ECL signal varies between different potentials with high repeatability and accuracy, which overcomes the key limitations of electrochemical analysis.2 Therefore, ECL has been widely used in biomolecular detection,12 food and drug analysis,13 clinical diagnosis,14 and environmental monitoring.15 However, a lack of suitable ECL emitters with biocompatibility, high QY and stable ECL intensity is the latest stumbling block in ECL evolution and application.16Fig. 1A shows the time line of the representative events in the development of ECL emitters and co-reactants. Among the numerous luminescent materials investigated in ECL history, the first candidate was metal complexes, specifically tris(2,2′-bipyridine)ruthenium(II)/tri-n-propylamine (Ru(bpy)32+/TPrA), which have high ECL intensity.2,17 However, their low photochemical stability leads to the evolution of novel luminophores. Quantum dots (QDs) as ECL emitters have received tremendous attention due to their unique quantum size-dependent optical and electrochemical properties. For example, Ding and Bard used Si QDs as an ECL emitter in 2002.18 Unfortunately, many of these QDs contain toxic heavy metals (Cd in CdSe or Pb in PbS), causing serious environmental and health concerns.19 Therefore, novel nontoxic ECL emitters are urgently required.
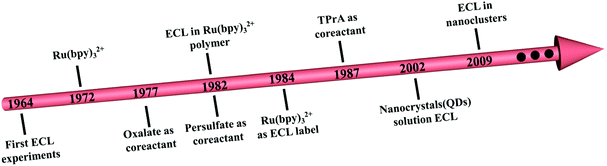 |
| Fig. 1 Time line of representative events in the development of ECL emitters and co-reactants. | |
Compared to QDs, nanoclusters (NCs), which have versatile molecule-like structures with excellent photostability, biocompatibility, ultra-small nanostructures and low biological toxicity, are still less reported as ECL emitters.20,21 The first case of ECL in NCs was Ag NCs coated with poly(methacrylic acid).22 Later, Ding and his team led the pioneer studies in ECL by using organic ligand-capped Au NCs with well-established electrochemical properties.23–26 In addition, the anodic ECL signal of Au NCs in the presence of tris(3-aminoethyl)amine was observed by Zhou and others in 2018.27 Although the aforementioned research shows the promising application of NCs in ECL, the low QY of the as-synthesized NCs still forms a barrier for their further advancement, especially in sensing and biology. Therefore, designing NCs with high QY is an inevitable step in the development of high-performance devices.
The applications of NCs in catalysis along with their growth mechanisms and physical or chemical properties have been sufficiently reviewed in the past decades.28–33 For example, Jin and his colleagues summarized the progress in the utilization of atomically precise metal NCs as electrochemical CO2 reduction reaction catalysts, hydrogenation reaction catalysts and electron-transfer catalysts at the single-atom and single-electron levels in 2020.32 Recently, we noticed some reviews discussing the developing of NCs for ECL measurements, but they merely introduced the various applications and did not summarize the methods to improve the QY.34–36 We believe that an in-depth understanding of the enhancement mechanism is also important. Therefore, we will focus on the strategies for improving the QY of NCs, which is the critical step in the further application of NCs. This mini-review will discuss the following aspects: (1) classification of nanoclusters; (2) the reasons for the low QY of NCs in ECL; (3) methods to improve ECL quantum efficiency (most important); (4) potential ECL applications of NCs; and (5) perspectives of NCs in ECL. Fig. 2 shows the main frame of this review. We hope to attract more attention from material scientists, inorganic chemists and analytical chemists to advance NC chemistry and promote NC applications in ECL.
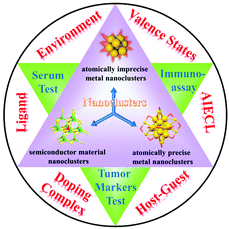 |
| Fig. 2 Classification of nanoclusters and methods to improve their quantum yield and applications. | |
2. Classification of nanoclusters
Nanoclusters of a few Å to 3 nm, a key bridge between molecular complexes and crystalized nanoparticles (Fig. 3),30,37 have been comprehensively studied in the past decades because of their aesthetically beautiful molecular structures and fascinating properties such as quantized electronic absorption, fluorescence, and catalysis, to name but a few general examples. Based on the results of current research, we have made a simple classification and summary of NCs that have been widely used as emitters in ECL. According to their composition, NCs can be classified into two groups: metal NCs and semiconductor material NCs; metal NCs comprise atomically precise and imprecise NCs.
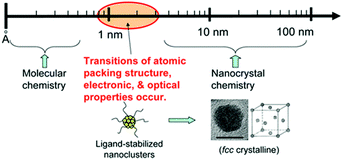 |
| Fig. 3 NCs bridge between organometallic complexes and crystalized nanoparticles. Reproduced with permission from ref. 37. Copyright 2010 Royal Society of Chemistry. | |
The main difference between metal NCs and semiconductor NCs is in their composition. Metal NCs are composed of metal cores and organic ligands on the surface. The metals can be Cu, Au and/or Ag, and the ligands include 2-phenylethanethiol (SR), glutathione (GSH), L-cysteine (L-Cys), etc. Semiconductor NCs are composed of semiconductor elements such as Cd, Zn, and In, while primary and secondary amines function as the ligands. The main difference between atomically precise metal NCs and imprecise metal NCs is in their structures and surface states. Atomically precise NCs have a definite number of metal cores and well-defined surface ligands. Due to the precisely controlled composition, atomically precise NCs usually exhibit excellent uniformity and mono-dispersity. As a result of molecule-like formulas and structures, atomically precise NCs show unique molecule-like properties, such as HOMO–LOMO transition, quantized charging, strong luminescence, and intrinsic chirality, which exhibit strong size/structure dependence.38–40 However, imprecise metal NCs are typically aggregates and their structure and the specific number of atoms are not clear. This review will mainly discuss the ECL properties and applications of these types of NCs.
2.1. Atomically precise metal nanoclusters
Because of the well-defined surface and precisely controlled composition, atomically precise MNCs are efficient ECL light-emitting materials. This type of MNCs is constituted by a core with a definite number of metal atoms and surrounded by ligand shells with organic molecules.38–40 Therefore, changes in the number of metal atoms of the precise core–shell structure could significantly affect their ECL properties. When the size of the MNCs decreases to a few metal atoms (<10 atoms), it is comparable to the Fermi wavelength and exhibits molecule-like properties, such as a HOMO–LUMO one-electron transition.41,42 Among the MNCs, Au NCs have received extensive attention due to their stable optical and electrochemical properties and low and well-defined LUMO energy gaps. In 2011, Zhu's group first reported the ECL emission of bovine serum albumin (BSA)-stabilized Au25.43 After that, the ECL performance of Au NCs with different molecular composition has been investigated including that of Au8,44 Au25,45 Au38,46 and Au144.47 It is worth noting that a spooling ECL spectroscopy technique was created by Ding's lab where the ECL intensity–wavelength curves were collected by potential scanning (Fig. 4).6,23 Using this detection method, they obtained strong and sustainable ECL signals from 2-phenylethanethiol (SR, ligand)-protected Au25z (i.e., Au25(SR)18z, z = 1−, 0, 1+), Au38(SR)24 and Au144(SR)60. Here, we want to specify some key points. First, among the Au25 NCs with different charge states, the ECL intensities of Au25+, Au250, and Au25− show a descending trend. Second, for the neutral forms of the MNCs with various core sizes, the obtained ECL efficacies follow the order Au38 > Au25 > Au144 in the TPrA co-reactant systems. Third, the concentrations of co-reactants around the working electrode controlled by the applied potential determine the wavelength of the ECL emissions. All of these will be discussed in the ECL mechanism of the NCs.
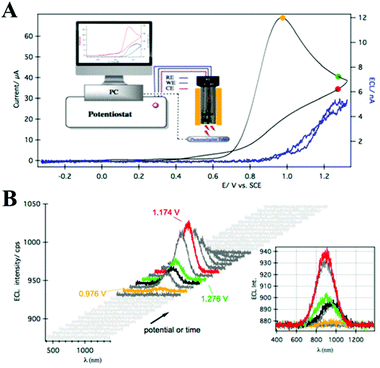 |
| Fig. 4 (A) ECL–voltage curve and CV of 0.1 mM Au25− with 12.5 mM TPrA at a scan rate of 100 mV s−1. The inset shows a schematic of the apparatus. (B) Spooling ECL spectra from the potential scanning in (A). The inset shows the stacked spooling spectra. Adapted with permission from ref. 23. Copyright 2014 Royal Society of Chemistry. | |
The bimetallic NCs of atomically precise MNCs have also received extensive attention in recent years. With ECL standard Ru(bpy)3 as reference, rod-shaped [Ag13Au12(PPh3)10(SC2H4Ph)5Cl2]2+ NCs generated a dramatic 10 and 400 times higher ECL of the self-annihilation path and co-reactant path, respectively.48 The strong ECL signal from Au12Ag13 is ascribed to the 13th Ag atom at the central position which not only stabilized the charges on the LUMO orbital, but also made the rod-shaped Au12Ag13 core more rigid (Fig. 5).
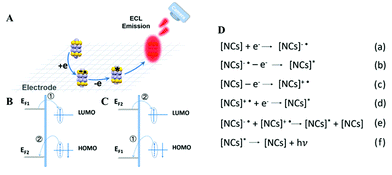 |
| Fig. 5 Experimental setup (A); proposed self-annihilation ECL reaction pathways for the oxidative (B) and reductive (C) ECL and the corresponding reactions (D) of Au12Ag13. Reprinted with permission from ref. 48. Copyright 2019, American Chemical Society. | |
2.2. Atomically imprecise metal nanoclusters
Atomically imprecise metal NCs with a size of 2–3 nm do not contain a specific number of atoms or ratio of metal atoms to surface ligands but show optical and electrical properties different from nanocrystals. Benefiting from their unique optoelectrical properties and low toxicity, MNCs such as Au NCs,49 Ag NCs,22 and Cu NCs19 are promising ECL luminophores. Here, we highlight several representative MNCs. Using bilayer graphene as a template, Zhu and his collaborators prepared a dispersible graphene/gold NC hybrid in the aqueous phase which peaked at −1.5 V with an intensity of 1900 a.u.50 The high affinity of Ag+ has enabled the creation of short oligonucleotide-encapsulated Ag NCs without the formation of large nanoparticles. Inspired by this finding, Dickson achieved in situ synthesis of NCs using DNA as a template.51 After that, Jie et al. introduced Ag NCs to ECL sensing to detect thrombin, resulting in a low detection limit of 4.5 fM at 3σ.52 However, the consumption of noble silver and gold is high. Therefore, a low-cost candidate with high-efficiency ECL emission is urgently needed, which has promoted the development of Cu NCs. The anodic and blue ECL emission of Cu NCs were observed for the first time with the efficient co-reactant hydrazine by Yuan's group.19 The possible luminescence mechanism of Cu NCs/HZ ECL system was studied in detail and is shown in the following section. Their team later designed a well-defined DNA nanocrane for the oriented immobilization of Cu NCs to programmably tune the sequence-dependent formation.53 They adjusted the longitudinal distance to 8 units of A–Cu–T and the lateral distance to 24 bases to ensure the best ECL efficiency for Cu NCs.
However, weakened ECL emission intensity caused by the short lifetime of the radical species restricted the further applications of monometallic NCs.54 Introducing another metal element into MNCs to form bimetallic NCs (BNCs) is an efficient method, which improves the performances of the illuminator, and therefore enhances the ECL efficiency.55 Similar to the silver effect on the increased fluorescence intensity of Au NCs, synergetic effects between the two metal elements can also improve the ECL performance.56 A series of bovine serum albumin-protected Au–Ag BNCs with different Au/Ag ratios was prepared in 2017 (Fig. 6(A)). Notably, the doped Ag atoms not only blue-shifted the emission of the Au NCs but also decreased the peak and onset potential. As a result, Au–Ag BNCs achieved a five-fold higher efficiency compared to monometallic NCs.57 Later, Zou's group explored a mechanistic route to enhance the ECL of Au–Ag BNCs over 30 times through synergetic effects by engineering the bandgap and surface defects (Fig. 6(B)).58
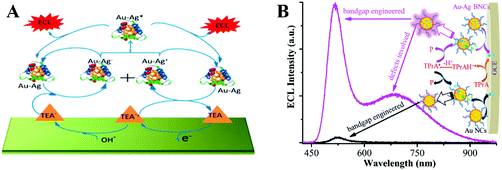 |
| Fig. 6 (A) Enhanced ECL mechanisms of Au–Ag bimetallic NCs. Reproduced with permission from ref. 57. Copyright 2017 American Chemical Society. (B) Schematic illustration for the synergetic effects enhanced ECL of Au–Ag BNCs than Au NCs. Reproduced with permission from ref. 58. Copyright 2020 Royal Society of Chemistry. | |
2.3. Semiconductor material nanoclusters
Metal chalcogenide NCs are among the most advanced solid-state materials because of their excellent chemical and physical properties. The first investigation of ECL behavior of molecule-type supertetrahedral metal chalcogenide nanoclusters (CdInS NCs) in aqueous solution was designed by Wu and his co-workers in 2016.59 Compared to MNCs, the CdInS NC supertetrahedron showed different ECL behaviors, due to the intrinsic vacancy point defect at the core site and the antisite point defects at the surface (we will come back to this point later). They also found that precisely doped mono-manganese ion at the vacant site can adjust the electronic structure of the host and tune the optical properties. Therefore, a relatively high ECL efficiency of 2.1% was achieved (Fig. 7).
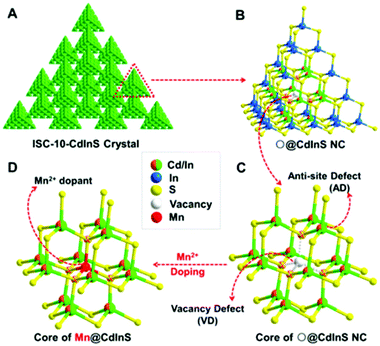 |
| Fig. 7 Structure of ISC-10-CdInS: (A) Composed of coreless ○@CdInS NC, (B) with intrinsic internal defect and (C) anti-site defects, which can trap one Mn2+ dopant at its vacant core site (D). Reprinted with permission from ref. 59. Copyright 2016, American Chemical Society. | |
3. Why the QY of NCs used in ECL is low
ECL is a kind of solution-based luminescence triggered by the high-energy electron transfer between electro-generated species. NCs with size-dependent HOMO–LUMO transition are regarded as a promising ECL emitter, since the dimension of NCs falls within the range of the Fermi wavelength of the conduction electrons (Fig. 8A). Understanding the luminescence mechanism of NCs during electrochemical reactions will help us to design and optimize strategies to improve NC performance in ECL. The annihilation pathway and the co-reactant pathway are two dominant mechanisms in ECL (Table 1). In the annihilation mechanism, as shown in eqn (1)–(4), the NCs on the electrode surface are first reduced or oxidized to radicals. Then the cation and anion radicals annihilate to form ground state NCs and excited state NCs, where the excited state NCs further covert to ground state NCs by emitting light.54 However, the short lifetime of the free radicals results in a low luminescence efficiency. Therefore, it is necessary to introduce either reducing intermediates or oxidizing intermediates to stabilize the chemically unstable luminescent radicals.5 Since the reducing intermediate species are generated after an electrochemical oxidation of a co-reactant (such as TPrA), the corresponding ECL reactions are referred to as “oxidative-reduction” ECL. Similarly, “reductive-oxidation” ECL denotes the reaction in which highly oxidizing intermediates are produced after an electrochemical reduction (such as BPO). Eqn (5)–(8) shows a general “oxidative-reduction” ECL mechanism while the “reductive-oxidation” one is listed in (9)–(12). What is noteworthy is that the mechanism of each specific luminophore NCs will be slightly different.
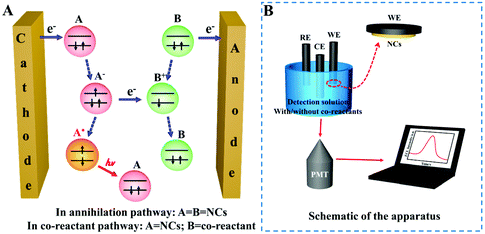 |
| Fig. 8 General principles schematic diagrams of ECL (A) and a schematic of the apparatus (B). | |
Table 1 ECL luminescence equation of NCs
♣ Annihilation mechanism: |
NCs − e → NCs˙+ (oxidation at electrode) |
(1) |
NCs + e → NCs˙− (reduction at electrode) |
(2) |
NCs˙+ + NCs˙− → NCs + NCs* (excited state formation) |
(3) |
NCs* → NCs + hν (light emission) |
(4) |
|
♠ “Oxidative-reduction” ECL mechanism: |
NCs + e → NCs˙− (reduction at electrode) |
(5) |
R − e → R˙+ (oxidation at electrode) |
(6) |
R ˙+ + NCs˙− → R + NCs* (excited state formation) |
(7) |
NCs* → NCs + hν (light emission) |
(8) |
|
♥ “reductive-oxidation” ECL mechanism: |
NCs − e → NCs˙+ (oxidation at electrode) |
(9) |
R + e → R˙− (reduction at electrode) |
(10) |
NCs˙+ + R˙− → R + NCs* (excited state formation) |
(11) |
NCs* → NCs + hν (light emission) |
(12) |
At present, the most used ECL detection instrument is the MPI-E ECL analyzer (Remex Electronic Instrument Co., Ltd, Xi'an, China). In addition, some groups have assembled their own instruments with new functions. For example, the spooling ECL spectroscopy created by Ding's lab collected the ECL intensity–wavelength curves by potential scanning. All the detections were implemented on a three-electrode system containing a Pt wire as the counter electrode, a saturated calomel or Ag/AgCl (saturated KCl) reference electrode, and gold, platinum or glassy carbon as the working electrode. The ECL detection solution often includes co-reactants in the co-reactant pathway. In an aqueous system, the detection solution is PBS or Tris-HCl buffer, while in the organic phase system, the mixed solution of toluene and acetonitrile including tetrabutylammonium perchlorate electrolyte is commonly used. As shown in Fig. 8b, the drop-cast NCs on the surface of the working electrode transit to their excited state after accepting electrons, and emit light when returning to the ground state. This light signal can be amplified by the photomultiplier tube (PMT) and collected on the display and finally becomes the ECL intensity.
For photochemical reactions, quantum yield is defined as the ratio of photons undergoing a photochemical reaction to the total number of photons absorbed. However, ECL is excited by electricity and not by absorbed photons. Therefore, ECL quantum yield is determined as the photons emitted per redox event relative to the NC system. It can be obtained by comparing the integrated ECL intensity (number of emitted photons) to the current value (number of consumed charges), using [Ru(bpy)3]2+ as a reference.60 Like quantum yield, ECL efficiency can also be used to characterize the luminous efficiency of materials. Therefore, ECL efficiency ≠ QY, but ECL efficiency = ECL QY.
where
r represents the reference system (Ru(bpy)
32+-TPrA) and
x is the nanocluster sample.
Although the luminescent NCs are potential candidates for ECL emitters, current ECL applications of NCs are almost in the NIR range where non-radiative decay pathways tend to surpass radiative pathways, resulting in low ECL quantum yields.
4 How to improve the quantum yield of nanoclusters
A high quantum yield (QY) of ECL in nanomaterials is necessary for a wide range of applications. The efforts of our predecessors to improve the QY of NC-based emitters have been reported (listed in Table 2). Herein, we will describe how to effectively enhance the quantum yield of NCs in four aspects: (I) environment effects; (II) construction; (III) valence state effects and (IV) aggregation-induced ECL.
Table 2 Summary of methods for improving the ECL quantum yield of NCs
Emitter |
Method |
QY or the times of ECL intensity increased |
Ref. |
NAC-Au NCs |
Valence state regulation |
30-fold, 4.11% |
49
|
(Au NCs/LDH)n |
Localization of Au NCs on LDHs nanosheets |
From 2.69% to 14.11% |
61
|
Mn1.36Zn5.64In28S56 |
Mn2+-doped |
27.1% |
62
|
Ox-Met-Au NCs |
Pre-oxidation |
66% |
63
|
ARG/ATT-Au NCs |
Host–Guest recognition |
0.03% to 67.02% |
64
|
ATT-Au NCs |
Electrocatalytic excitation and aggregation-induced emission |
78% |
7
|
Eu3+-Cu NCs |
Eu(III)-doped |
About 2.5 times higher of Cu NCs |
65
|
Au-LA-DEDA |
Covalent attachment of co-reactant DEDA onto Au-LA |
About 15 times higher of Au-LA |
66
|
ATT-Au-Ag BNCs |
Enhanced band gap engineered and surface defect |
About 30 times higher of Au NCs |
58
|
Ag NCs/TiO2 NFs |
TiO2 NFs as the co-reaction accelerator |
About 100 times higher of Ag NCs |
67
|
4.1. Environment
A co-reactant is a chemical species which decomposes immediately upon electrochemical oxidation or reduction. The produced strong reducing or oxidizing intermediates later react with an oxidized or reduced ECL luminophore to generate excited states. Because the co-reactant controls the generation of excited states, the ECL intensity of NCs is different with a variety of different co-reactants. Zou's team proved this by exploring the effects of different co-reactants on the ECL intensity of Met-Au NCs.16 We can see from Fig. 9(A) that triethanolamine (TEOA) is the most effective co-reactant for the ECL of Met-AuNCs among the seven co-reactants (TEOA, TEA, TPrA, TMA, DEA, DEAE, and TPIA) tested. Previous experiments have proved that both the type of the co-reactant and its concentration affect ECL efficiency. Ding's team explored the effect of the concentration of co-reactant TPrA on the ECL quantum efficiency of Au25 and Au38.23,46 It is interesting to note that the ECL QY was influenced by the concentration of the co-reactant within a certain range. For example, the ECL intensity showed a positive linear relationship with TPrA concentration when [TPrA] was lower than 50 mM. However, when the concentration of TPrA was higher than 50 mM, the ECL intensity of the emitter was quenched. Not only that, when [TPrA] was larger than 100 mM, the ECL quenching in the Ru(bpy)32+/TPrA co-reactant system was more dramatic than that in the Au25/TPrA or Au38/TPrA system with high [TPrA], causing a potential overestimated QY (Fig. 9(B)). Therefore, both the type and concentration of the co-reactant are important in NC ECL. Table 3 lists the comparison of ECL QY in different co-reactant systems.
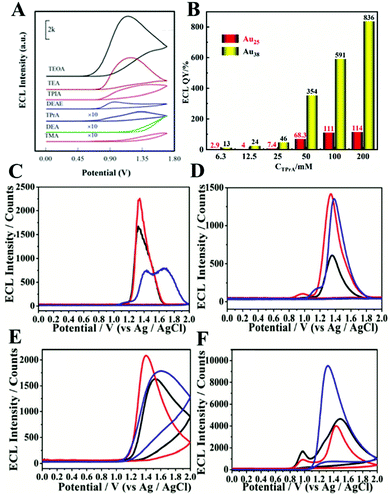 |
| Fig. 9 (A) ECL-potential profiles of the Met-Au NCs/GCE in 0.1 M, pH 7.4 PBS containing 100 mM different co-reactant and (B) ECL QY of Au25 and Au38 with different concentration of TPrA. ECL curves in different detection solution (C) PBS (95%), (D) BBS (95%), and (E) Tris-HCl (95%) in the absence of TPrA; (F) Tris-HCl (95%) in the presence of 1 mM TPrA. (A) Reprinted with permission from ref. 16. Copyright 2020, American Chemical Society. The data for (B) comes from ref. 23 and 46. Copyright 2020, American Chemical Society; (C)–(F) reprinted with permission from ref. 68. Copyright 2015, Royal Society of Chemistry. | |
Table 3 Comparison of the ECL QY in different co-reactant systems
ECL emitters |
Co-reactant |
QY(%) |
Ref. |
Met-AuNCs |
K2S2O8 |
2.23 |
69
|
Met-AuNCs |
TEA |
8 |
63
|
BSA-AuNCs |
TEA |
9.8 |
45
|
BSA-AuNCs |
K2S2O8 |
0.325 |
69
|
The effects on quantum efficiency due to other environmental conditions, such as the test solution, pH, and electrode materials, have also been investigated. Wei's group claimed that the ECL response of two windmill-like Ag3Cu5 alkynyl NCs was most sensitive in Tris-HCl-CH3CN, but not in PBS-CH3CN or BBS-CH3CN.68 They also found that the ECL intensity was enhanced with the increased pH in the range of 7.5–9.0, but diminished sharply under high pH conditions. All of the aforementioned results can demonstrate the influence of environmental factors on the ECL QY of NCs.
4.2. Construction
As we mentioned above, the NCs are composed of metal cores and organic ligand shells. Therefore, changing the number of metal atoms or the type of ligands could significantly affect their ECL properties. In this section, we will focus on the structure of NCs and explain its impact on ECL efficiency from the aspects of ligand effects, doping, composite, host–guest recognition, etc.
4.2.1. Ligand effects.
The optoelectrical properties of NCs significantly depend on their surface conditions, specifically the electron exchange between the NCs and the electrode.70 To stabilize the NCs, various ligands have been introduced, including bovine serum albumin (BSA), L-methionine (Met), glutathione (GSH), L-cysteine (L-Cys), N,N-diethylethylenediamine (DEDA), N-acetyl-L-cysteine (NAC), 6-aza-2-thiothymine (ATT) for atomically imprecise MNCs and 2-phenylethanethiol (PET) for atomically precise MNCs. By adjusting the surface ligands, the ECL performance of NCs can be systematically studied. As shown in Fig. 10(A) and (B), compared with BSA-Au NCs and CR BSA-Au NCs, Met-Au NCs had a strong anodic ECL intensity (about 75 and 3.8 times higher than BSA-Au NCs and CR BSA-Au NCs, respectively).16 This could be attributed to the nonconductive protein shell in BSA-Au NCs which had much higher electron-transfer resistance than that of Met-Au NCs, resulting in the decrease of the electron transfer rate between the modified electrode and co-reactant. Apart from this, chemical reduction treatment of Met-Au NCs in this work could result in a red shift of the ECL wavelength, which was consistent with Kim's work.71 Similarly, four kinds of ligands consisting of poly-L-Cys, GSH, BSA, and L-Cys were employed to estimate the ECL performance of synthesized Cu NCs by Zhuo and her co-workers.72 Poly-L-Cys protected Cu NCs exhibited a wonderful ECL intensity (12
294 a.u., 2–4 times for others) and efficiency (1.2–3.7 times for others) compared to other ligand protected ones. They explained that a porous structure provided by poly-L-Cys could regulate the generation of Cu NCs, which not only impeded the intramolecular vibration and rotation and reduced the nonexcited relaxation of the ligands but also expedited the electron transfer near the electrode surface between the active intermediates Cu NC− and the oxidized intermediates SO4˙−. Table 4 lists the examples in which the ECL quantum yield was compared under different ligand systems.
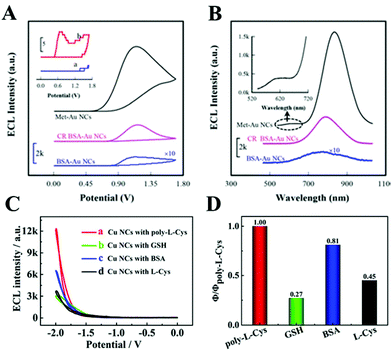 |
| Fig. 10 (A) ECL-potential profiles and (B) ECL spectra of surface confined Au NCs in 0.1 M pH 7.4 PBS containing 100 mM TEOA. Reprinted with permission from ref. 16. Copyright 2020, American Chemical Society. ECL intensity (C) and relative ECL efficiency (D) of the Cu NCs synthesized by different ligands. Reprinted with permission from ref. 72. Copyright 2020, American Chemical Society. | |
Table 4 Comparison of the ECL QY of different NCs ligands
ECL emitters |
Co-reactant |
QY (%) |
Ref. |
Met-AuNCs |
K2S2O8 |
2.23 |
69
|
BSA-AuNCs |
K2S2O8 |
0.325 |
69
|
NAC-AuNCs |
K2S2O8 |
4.11 |
49
|
GSH-AuNCs |
TEA |
0.42 |
71
|
BSA-AuNCs |
TEA |
9.8 |
45
|
Met-AuNCs |
TEA |
8 |
63
|
4.2.2. Doping.
Inserting transition metal dopants intentionally can adjust the electronic states of NCs and tune their optoelectronic properties.73 Studies of modulating the electro-generated ECL intensity and ECL efficiency of host materials by introducing metal atomic dopants have been reported.35 For example, Cui's group conducted a series of in-depth research studies on this aspect. They obtained an ECL efficiency of 27.1% from in situ Mn2+-doped Zn6In28S56 supertetrahedral NCs in 2018, which was 90 times higher than that of undoped Zn6In28S56 NCs (Fig. 11(A) and (B)).62 With the assistance of DFT calculations, they further explored the mechanism of the enhancement. The heteroatom Mn2+ ion with half-occupied 3d orbitals exhibited considerable variability compared to the Zn2+ ion, which indicated that the unoccupied orbitals from the dopants may affect the QY of NCs. Along this line, a mono-copper ion-doped Cu@CdInS was obtained an efficiency of 21.72%, as compared to [Ru(bpy)3]2+ in the same year.74 The same phenomenon was also observed in MNCs. The rare-earth element Eu(III) with rich electronic structures improved the stability of Cu NCs by forming an Eu(III) complex on the surface of Cu NCs. The formed new surface states promoted effective energy transfer from the host to the Eu3+ dopant, and thereby improved three-fold the ECL intensity of Cu NCs (Fig. 11(C) and (D)).65
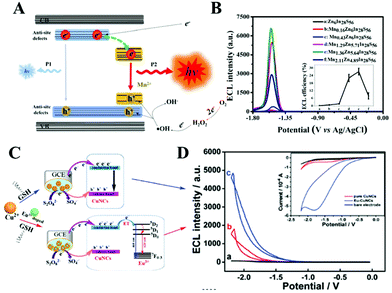 |
| Fig. 11 Proposed ECL radiation paths in the in situ Mn2+-doped MnxZnyIn28S56 (A) and ECL-potential curves of different Mn2+-doping concentrations. (B) Reprinted with permission from ref. 62. Copyright 2018, American Chemical Society. Comparison of ECL mechanisms of Cu NCs and Eu3+-Cu NCs. (C) and (D) Reprinted with permission from ref. 65. Copyright 2020, Royal Society of Chemistry. | |
4.2.3. Composite.
In addition to ligands and dopants, embedding NCs with other materials is also an efficient way to improve the ECL QY. One type of species is a metal–organic framework which serves as a carrier. For example, the nanocomposite of zirconium metal–organic framework (Zr-MOF, also known as UiO-66) embedded with Ag NCs showed great ECL performance with good biocompatibility, high specific surface area and strong bioaffinity.75 The other type of species can function as both carrier and co-reaction accelerator, which enhances the efficiency of ECL through the increased co-reaction intermediates. As the most typical example, TiO2 nano-flowers, a kind of prominent metal oxide semiconductor exhibiting bandgap-dependent catalytic properties, could promote the generation of the strong oxidizing intermediate radical OH˙ through the reduction of dissolved O2. The in situ generated radical OH˙ further reacted with Ag NCs to produce excited-state species Ag NCs* for emitting ECL remarkably.67 However, the ambiguity of mass transport between reactants in the conventional ECL generation pathway greatly impedes the improvement of ECL efficiency. Wang and his co-workers were committed to reduce this barrier by constructing N,N-diethylethylenediamine (DEDA)-Au nanocomposites in which DEDA served as the co-reactant and covalently attached onto lipoic acid-stabilized Au NCs (Au-LA).66 As we can see from Fig. 12, the Au NCs exhibited an extremely high ECL intensity (about 100 times) compared with the mixture of Au-LA and 5 mM DEDA after forming the nanocomposites, indicating that the enhanced ECL efficiency also benefits from the multiple energy states of Au NCs and multiple DEDA ligands in the monolayer.
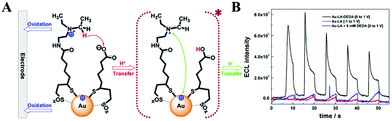 |
| Fig. 12 (A) Stepwise ECL generation of Au-LA-DEDA NCs and (B) ECL profiles of 6 μM Au-LA NCs with DEDA covalently attached (black), without co-reactant (red), and with 5 mM free diffusing DEDA (blue) in solution. Reprinted with permission from ref. 66. Copyright 2016, American Chemical Society. | |
4.2.4. Host–guest recognition.
Host–guest recognition is a molecular interaction based on host–guest chemistry. This concept is used to describe a process of constructing supramolecules, where a highly selective host and its complementary guests form complexes through unique structural relationships and non-covalent interactions. The non-covalent interactions, including electrostatic force, ionic bonding, hydrogen bonding, and van der Waals forces, can provide stability to the supramolecules in biological systems and afford inherent reversibility.76
Inspired by the fact that the Au-thiolate shell can enhance the photoluminescence (PL) of NCs,77 host–guest recognition is employed to improve the ECL efficiency of NCs.61 Yan's team introduced a strategy to place Au NCs on 2D layered double hydroxide (LDHs) nanosheets through a layer-by-layer assembly process. Due to the host–guest interaction, Au NCs were stabilized in the confined environment and the nonradiative transitions were greatly reduced. This process increased the content of emissive Au(I)–thiol units in the NCs, resulting in a greatly improved QY of Au NCs (up to 14.11%). Moreover, much prominent fluorescence can be observed after formation of the assembled complexes, (Au NCs/LDH)n (n = 0–20), with various layers of LDH nanosheets. Similar to LDH, L-arginine (ARG) can form the rigid host–guest assemblies with ATT-Au NCs to improve the ECL QY from 0.03% (ATT-Au NCs) to 67.02% (ARG/ATT-Au NCs).64Fig. 13 shows various ECL intensities from Au NCs with different surface states; typically, the ECL QY of ARG/ATT-Au NCs with co-reactant ECLa1 was about 70 times higher than that of the ATT-Au NCs. Therefore, the authors make a conclusion that rigid host–guest assemblies can hasten the radiative charge transfer between the oxidized Au NCs and the reduced TPrA˙ radicals while keeping their original excited states.
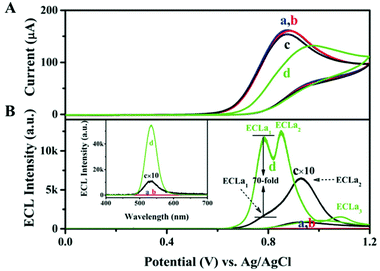 |
| Fig. 13 (A) CV and (B) potential-resolved ECL of GCE in 50 mmol L−1 pH 7.4 Tris-HCl containing (a) 10 mmol L−1 TPrA, (b) (a) +3 mmol L−1 ARG, (c) (a) +ATT-Au NCs, (d) (a) +ARG/ATT-Au NCs at 10 mV s−1. Inset: Corresponding accumulated ECL spectra by collecting all photons generated during the whole course of potential cycling over 320 s. Reprinted with permission from ref. 64. Copyright 2019, Wiley Online Library. | |
4.3. Valence state effect
The valence states of the main metal atoms in NCs, determining their ability to gain and lose electrons, affect the ECL intensity and QY. We name this influence as the valence state effect. For example, Au NCs have different valence states, typically as Au(I) and Au (0).49 In recent years, studies on the QY of Au NCs have demonstrated the vital role of the valence state in ECL performance. The electrochemical method to reduce the Au NCs from Au(I) to Au (0) was first employed to obtain 30 times higher ECL intensity by Chen's group in 2017 (Fig. 14(A)).49 They demonstrated that the reduction potential determines the electrochemical reduction degree of Au NCs, which affected the ECL intensity. Specifically, the increase of the ECL intensity was positively correlated with the ratio of the Au (0) and a good linear relationship between the ratio of Au (0) and ECL intensity can be established, as shown in Fig. 14(B). In 2019, a record high ECL QY of 66% achieved by pre-oxidation of Au NCs was reported, which was about 8.25-fold higher than that of Met-Au NC (8%) (Fig. 14(C) and (D)).63 To confirm the role of the oxidation state of Au in the enhanced ECL, the Ox-Met-Au NC/GCE (glass carbon electrode) was then electrochemically reduced at −1.5 V for 50 s. After the reduction, the ECL almost returns to the initial value, which can be explained by the depletion of the Ox-Met-Au NCs by the reduction. This further proves that the valence state plays an important role in the ECL performance of the NCs.
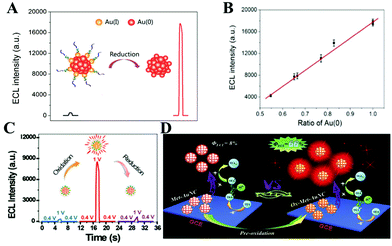 |
| Fig. 14 Illustration of the improved ECL performance (A). (B) Linear relationship between the ratio of Au(0) and the ECL intensity. Reprinted with permission from ref. 49. Copyright 2017, American Chemical Society. (C) and (D) Schematic of enhanced ECL performance after the pre-oxidation treatment. Reprinted with permission from ref. 63. Copyright 2019, Wiley Online Library. | |
4.4. Aggregation-induced luminescence
Aggregation-induced emission or AIE was discovered by Tang and his colleagues in 2001. Unlike aggregation-caused quenching, or ACQ, AIE describes an interesting light emission phenomenon that luminophores only show strong fluorescence in the aggregated state, but appear weakly fluorescent (PL) or non-fluorescent in the solution state.78–85 Since restriction of intramolecular motion can enhance the PL of NCs efficiently, aggregation-induced ECL (AIECL) has emerged. To test the superiority and extensibility of AIE materials, the bright aggregated state cathodic ECLs of TPE-based derivatives was reported through a co-reactant approach for the first time.86 Liu's work demonstrated that drying ATT-Au NCs on electrodes enhanced by both electrochemical excitation by an electrocatalytic effect and emission by AIECL, resulted in a record high ECL efficiency of 78%.7 They speculated that drying could not only suppress the vibration and rotation of ATT but also reduce non-radiative relaxation from excited state to ground state.
5. Application
As a new emerging class of nanomaterials, NCs with excellent optical and electronic properties have found great applications in many areas, such as tumor markers test, immunoassay, and serum test (Fig. 15(A)).
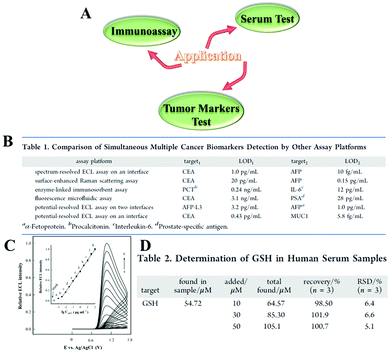 |
| Fig. 15 (A) Application diagram of ECL NCs. (B) Comparison of simultaneous multiple cancer biomarkers detection by other assay platforms. Reprinted with permission from ref. 87. Copyright 2019, American Chemical Society. (C) ECL response of the immunosensor to samples containing AFP of different concentrations. Inset: Corresponding calibration curve. Reprinted with permission from ref. 16. Copyright 2020, American Chemical Society. (D) Determination of GSH in Human Serum Samples. Reprinted with permission from ref. 88. Copyright 2020, American Chemical Society. | |
Tumor markers are biochemical substances that indicate the presence of tumors in the body and reflect certain biological characteristics. Common tumor markers include carcinoembryonic antigen, α-fetoprotein, miRNA, etc., and their abnormal expression is related to one or more cancers. Yuan and his team used Au25 NCs as an efficient bipolar ECL probe to establish a one-step method for simultaneous determination of carcinoembryonic antigen (CEA) and mucin 1 (MUC1).87 The calibration curve showed a linear relationship between the ECL response and the logarithmic values of the dual biomarkers. The detection range is from 1 pg mL−1 to 1 ng mL−1 for CEA and 0.43 pg mL−1 and 5.8 fg mL−1 for MUC1, which suggested better sensitivity and accuracy compared with other reported assay platforms for simultaneous multiple biomarker detection (Fig. 15(B)).
The immunoassay with antibody–antigen–antibody sandwich structure has attracted wide attention due to its advantages such as rapid detection, stable results and high sensitivity. In 2020, Zou's group reported an immunoassay using methionine-stabilized Au nanoclusters (Met-Au NCs) as tags to detect a model analyte, α-fetoprotein (AFP).16 This sandwich-type immunosensor had a wide linear range from 3 fg mL−1 to 0.1 ng mL−1 with a detection limit of 1 fg mL−1 (S/N = 3) and desired selectivity (Fig. 15(C)).
Zhuo and his colleagues built a ternary AuAg NCs/triethylamine/Ag NPs system for ECL detection of glutathione (GSH), and obtained a wide linear range from 5 to 200 μM with the detection limit as low as 0.90 μM.88 GSH is a tripeptide containing γ-amide bonds and sulfhydryl groups which are composed of glutamic acid, cysteine and glycine and exists in almost every cell of the body. However, the overexpression of GSH could disrupt the redox balance and cause many diseases, such as cancer, aging, and other ailments. Therefore, they carried out a recovery experiment in human serum samples to verify the applicability of the developed biosensor. According to Fig. 15(D), the recoveries were in the range of 98.5%–101.9%, which indicated that the developed biosensor was feasible for measuring GSH in biological systems.
6. Conclusions and future prospects
Impressive progress in the ECL technique for detecting faint bioelectricity and evaluating novel electrocatalysts has been achieved in the past few decades. At present, NCs, as a newly emerging family of ECL emitters show excellent stability, low biological toxicity, and tunable optical/electrochemical features. In this mini-review, we presented an overview of the recent progress in ECL performance of NCs. In particular, the annihilation and co-reactant ECL mechanism of NCs was explained and various methods that have been developed to improve the QY, and to enhance the ECL signal and stability of the biosensor were summarized. However, most of the aforementioned studies merely target novel metal NCs and Cd based semiconductor NCs, whereas other types of NCs with various compositions and structures along with mechanisms and properties of photoluminescence are also deserving of systematic studies.
Future studies need to focus on the following aspects: (a). Synthesis development. As discussed above, most of the studies were concentrated in the field of MNCs and Cd based semiconductor NCs. Other semiconductor NCs such as ME (where M = Al, Ga, In; E = P, As) III–V group non-toxic material NCs are still required to be studied carefully. In addition, ternary or multi-element NCs obtained by ion exchange or doping is also an important branch. As we all know, most of the NCs emit in the near-IR range by taking advantage of lower background interference and longer penetration depth. However, ECL in near-IR has received far less attention compared to near-IR fluorescence. There are three main reasons that have caused the ECL in near-IR to receive far less attention compared to near-IR fluorescence at present. First, the ECL emissions of currently available NIR-emitting QDs are relatively weak. Second, the phosphors are unstable. Besides, the detectors of ECL instruments toward NIR luminescence are insensitive. Thus, the main challenges of ECL NIR compared to that of fluorescence are to find stable and strong NIR ECL emitters and then explore effective approaches to enhance NIR ECL. (b). Mechanism development. Semiconductor NCs can be classified into two groups: stoichiometric (such as (CdSe)13,89 (CdSe)3490) and non-stoichiometric (such as In37P2091) semiconductor NCs. Therefore, it is worth studying the relationship between NC structures and their ECL performance and further exploring the mechanism of vacancy point defects induced ECL behavior in semiconductor NCs. For example, what is the difference in performance between ECL induced by the ordered intrinsic vacancy point defect in NCs and conventional ECL? (c). Application development. With fast developments of luminophore and optical components, the visualization of ECL at the electrode surface, and in even single cells and nanoparticles is a new direction. The luminous bodies currently used in ECL imaging include reduced graphene oxide,92 Au@L012 and g-C3N4,93 quantum dots,94etc. NCs are expected to become novel ECL imaging luminous bodies due to their single particles and high dispersion. The premise is that the QY of NCs should be improved to enhance the ECL intensity.
In spite of the current challenges and shortcomings of NC application in ECL technique, we believe that research efforts toward a better understanding of the relationship between the NC structures and luminescence mechanisms, procedures in the near-infrared ECL and ECL imaging will lead us to advance NC chemistry in next-generation ECL applications.
Conflicts of interest
There are no conflicts to declare.
Notes and references
- D. M. Hercules, Science, 1964, 145, 808–809 CrossRef CAS.
- M. M. Richter, Chem. Rev., 2004, 104, 3003–3036 CrossRef CAS.
- W. Miao, Chem. Rev., 2008, 108, 2506–2553 CrossRef CAS.
- A. B. Nepomnyashchii and A. J. Bard, Acc. Chem. Res., 2012, 45, 1844–1853 CrossRef CAS.
- M. Hesari and Z. Ding, J. Electrochem. Soc., 2015, 163, H3116–H3131 CrossRef.
- M. Hesari and Z. Ding, Acc. Chem. Res., 2017, 50, 218–230 CrossRef CAS.
- H. Peng, Z. Huang, H. Deng, W. Wu, K. Huang, Z. Li, W. Chen and J. Liu, Angew. Chem., 2020, 59, 9982–9985 CrossRef CAS.
- N. M. Andoy, X. Zhou, E. Choudhary, H. Shen, G. Liu and P. Chen, J. Am. Chem. Soc., 2013, 135, 1845–1852 CrossRef CAS.
- Y. Zhao, L. Tan, X. Gao, G. Jie and T. Huang, Biosens. Bioelectron., 2018, 110, 239–245 CrossRef CAS.
- D. Jiang, Y. Jiang, Z. Li, T. Liu, X. Wo, Y. Fang, N. Tao, W. Wang and H.-Y. Chen, J. Am. Chem. Soc., 2017, 139, 186–192 CrossRef CAS.
- K. Imura, H. Okamoto, M. K. Hossain and M. Kitajima, Nano Lett., 2006, 6, 2173–2176 CrossRef CAS.
- J. Ge, Y. Zhao, X. Gao, H. Li and G. Jie, Anal. Chem., 2019, 91, 14117–14124 CrossRef CAS.
- F.-F. Wu, Y. Zhou, J.-X. Wang, Y. Zhuo, R. Yuan and Y.-Q. Chai, Sens. Actuators, B, 2017, 243, 1067–1074 CrossRef CAS.
- L. Tan, J. Ge, M. Jiao, G. Jie and S. Niu, Talanta, 2018, 183, 108–113 CrossRef CAS.
- J. Zhao, Y.-M. Lei, Y.-Q. Chai, R. Yuan and Y. Zhuo, Biosens. Bioelectron., 2016, 86, 720–727 CrossRef CAS.
- L. Yu, Q. Zhang, Q. Kang, B. Zhang, D. Shen and G. Zou, Anal. Chem., 2020, 92, 7581–7587 CrossRef CAS.
- G. Jie, J. Ge, X. Gao and C. Li, Biosens. Bioelectron., 2018, 118, 115–121 CrossRef CAS.
- Z. Ding, B. M. Quinn, S. K. Haram, L. E. Pell, B. A. Korgel and A. J. Bard, Science, 2002, 296, 1293–1297 CrossRef CAS.
- M. Zhao, A. Y. Chen, D. Huang, Y. Zhuo, Y. Q. Chai and R. Yuan, Anal. Chem., 2016, 88, 11527–11532 CrossRef CAS.
- S. Roy, A. Baral, R. Bhattacharjee, B. Jana, A. Datta, S. Ghosh and A. Banerjee, Nanoscale, 2015, 7, 1912–1920 RSC.
- Z. Zhou and S. Dong, Nanoscale, 2015, 7, 1296–1300 RSC.
- I. Díez, M. Pusa, S. Kulmala, H. Jiang, A. Walther, A. S. Goldmann, A. H. E. Müller, O. Ikkala and R. H. A. Ras, Angew. Chem., Int. Ed., 2009, 48, 2122–2125 CrossRef.
- M. Hesari, M. S. Workentin and Z. Ding, Chem. Sci., 2014, 5, 3814–3822 RSC.
- C.-A. J. Lin, T.-Y. Yang, C.-H. Lee, S. H. Huang, R. A. Sperling, M. Zanella, J. K. Li, J.-L. Shen, H.-H. Wang, H.-I. Yeh, W. J. Parak and W. H. Chang, ACS Nano, 2009, 3, 395–401 CrossRef CAS.
- K. N. Swanick, M. Hesari, M. S. Workentin and Z. Ding, J. Am. Chem. Soc., 2012, 134, 15205–15208 CrossRef CAS.
- M. Hesari, M. S. Workentin and Z. Ding, Chem. – Eur. J., 2014, 20, 15116–15121 CrossRef CAS.
- Y. Zhou, S. Chen, X. Luo, Y. Chai and R. Yuan, Anal. Chem., 2018, 90, 10024–10030 CrossRef CAS.
- P. Zhang, J. Phys. Chem. C, 2014, 118, 25291–25299 CrossRef CAS.
- X. Kang and M. Zhu, Chem. Mater., 2019, 31, 9939–9969 CrossRef CAS.
- R. Jin, C. Zeng, M. Zhou and Y. Chen, Chem. Rev., 2016, 116, 10346–10413 CrossRef CAS.
- X. Kang and M. Zhu, ACS Mater. Lett., 2020, 1303–1314, DOI:10.1021/acsmaterialslett.0c00262.
- R. Jin, G. Li, S. Sharma, Y. Li and X. Du, Chem. Rev., 2020 DOI:10.1021/acs.chemrev.0c00495.
- S. Zhao, R. Jin and R. Jin, ACS Energy Lett., 2018, 3, 452–462 CrossRef CAS.
- S. He and Z. Ding, Curr. Opin. Electrochem., 2018, 7, 109–117 CrossRef CAS.
- L. Li, Y. Chen and J. J. Zhu, Anal. Chem., 2017, 89, 358–371 CrossRef CAS.
- C. Ma, Y. Cao, X. Gou and J. J. Zhu, Anal. Chem., 2020, 92, 431–454 CrossRef CAS.
- R. Jin, Nanoscale, 2010, 2, 343–362 RSC.
- Q. Yao, T. Chen, X. Yuan and J. Xie, Acc. Chem. Res., 2018, 51, 1338–1348 CrossRef CAS.
- I. Chakraborty and T. Pradeep, Chem. Rev., 2017, 117, 8208–8271 CrossRef CAS.
- Q. Yao, X. Yuan, T. Chen, D. T. Leong and J. Xie, Adv. Mater., 2018, 30, e1802751 CrossRef.
- H. Qian, M. Zhu, Z. Wu and R. Jin, Acc. Chem. Res., 2012, 45, 1470–1479 CrossRef CAS.
- S. Mustalahti, P. Myllyperkiö, S. Malola, T. Lahtinen, K. Salorinne, J. Koivisto, H. Häkkinen and M. Pettersson, ACS Nano, 2015, 9, 2328–2335 CrossRef CAS.
- L. Li, H. Liu, Y. Shen, J. Zhang and J.-J. Zhu, Anal. Chem., 2011, 83, 661–665 CrossRef CAS.
- W. Guo, J. Yuan and E. Wang, Chem. Commun., 2012, 48, 3076–3078 RSC.
- Y.-M. Fang, J. Song, J. Li, Y.-W. Wang, H.-H. Yang, J.-J. Sun and G.-N. Chen, Chem. Commun., 2011, 47, 2369–2371 RSC.
- M. Hesari, M. S. Workentin and Z. Ding, ACS Nano, 2014, 8, 8543–8553 CrossRef CAS.
- M. Hesari, Z. Ding and M. S. Workentin, Organometallics, 2014, 33, 4888–4892 CrossRef CAS.
- S. Chen, H. Ma, J. W. Padelford, W. Qinchen, W. Yu, S. Wang, M. Zhu and G. Wang, J. Am. Chem. Soc., 2019, 141, 9603–9609 CrossRef CAS.
- H. Peng, M. Jian, H. Deng, W. Wang, Z. Huang, K. Huang, A. Liu and W. Chen, ACS Appl. Mater. Interfaces, 2017, 9, 14929–14934 CrossRef CAS.
- Y. Chen, Y. Shen, D. Sun, H. Zhang, D. Tian, J. Zhang and J.-J. Zhu, Chem. Commun., 2011, 47, 11733–11735 RSC.
- J. T. Petty, J. Zheng, N. V. Hud and R. M. Dickson, J. Am. Chem. Soc., 2004, 126, 5207–5212 CrossRef CAS.
- G. Jie, L. Tan, Y. Zhao and X. Wang, Biosens. Bioelectron., 2017, 94, 243–249 CrossRef CAS.
- Y. Zhou, H. Wang, H. Zhang, Y. Chai and R. Yuan, Anal. Chem., 2018, 90, 3543–3549 CrossRef CAS.
- Q. Zhai, J. Li and E. Wang, ChemElectroChem, 2017, 4, 1639–1650 CrossRef CAS.
- R. Ferrando, J. Jellinek and R. L. Johnston, Chem. Rev., 2008, 108, 845–910 CrossRef CAS.
- Q. Zhou, Y. Lin, M. Xu, Z. Gao, H. Yang and D. Tang, Anal. Chem., 2016, 88, 8886–8892 CrossRef CAS.
- Q. Zhai, H. Xing, X. Zhang, J. Li and E. Wang, Anal. Chem., 2017, 89, 7788–7794 CrossRef CAS.
- J. Liu, R. Zhao, X. Wang, X. Gao and G. Zou, Chem. Commun., 2020, 56, 5665–5668 RSC.
- F. Wang, J. Lin, T. Zhao, D. Hu, T. Wu and Y. Liu, J. Am. Chem. Soc., 2016, 138, 7718–7724 CrossRef CAS.
- Y. Cao, W. Zhu, L. Li, Z. Zhang, Z. Chen, Y. Lin and J. J. Zhu, Nanoscale, 2020, 12, 7321–7329 RSC.
- R. Tian, S. Zhang, M. Li, Y. Zhou, B. Lu, D. Yan, M. Wei, D. G. Evans and X. Duan, Adv. Funct. Mater., 2015, 25, 5006–5015 CrossRef CAS.
- F. Wang, J. Lin, S. Yu, X. Cui, A. Ali, T. Wu and Y. Liu, ACS Appl. Mater. Interfaces, 2018, 10, 38223–38229 CrossRef CAS.
- H. Peng, Z. Huang, Y. Sheng, X. Zhang, H. Deng, W. Chen and J. Liu, Angew. Chem., Int. Ed., 2019, 58, 11691–11694 CrossRef CAS.
- L. Yang, B. Zhang, L. Fu, K. Fu and G. Zou, Angew. Chem., Int. Ed., 2019, 58, 6901–6905 CrossRef CAS.
- X. Zhuang, X. Gao, C. Tian, D. Cui, F. Luan, Z. Wang, Y. Xiong and L. Chen, Chem. Commun., 2020, 56, 5755–5758 RSC.
- T. Wang, D. Wang, J. W. Padelford, J. Jiang and G. Wang, J. Am. Chem. Soc., 2016, 138, 6380–6383 CrossRef CAS.
- Y. Zhou, H. Wang, Y. Zhuo, Y. Chai and R. Yuan, Anal. Chem., 2017, 89, 3732–3738 CrossRef CAS.
- Y. Jiang, W. J. Guo, D. X. Kong, Y. T. Wang, J. Y. Wang and Q. H. Wei, Dalton Trans., 2015, 44, 3941–3944 RSC.
- H. Peng, H. Deng, M. Jian, A. Liu, F. Bai, X. Lin and W. Chen, Microchim. Acta, 2016, 184, 735–743 CrossRef.
- G. Valenti, E. Rampazzo, S. Kesarkar, D. Genovese, A. Fiorani, A. Zanut, F. Palomba, M. Marcaccio, F. Paolucci and L. Prodi, Coord. Chem. Rev., 2018, 367, 65–81 CrossRef CAS.
- J. M. Kim, S. Jeong, J. K. Song and J. Kim, Chem. Commun., 2018, 54, 2838–2841 RSC.
- M. C. Pan, Y. M. Lei, Y. Q. Chai, R. Yuan and Y. Zhuo, Anal. Chem., 2020, 92, 13581–13587 CrossRef CAS.
- D. Mocatta, G. Cohen, J. Schattner, O. Millo, E. Rabani and U. Banin, Science, 2011, 332, 77–81 CrossRef CAS.
- F. Wang, J. Lin, H. Wang, S. Yu, X. Cui, A. Ali, T. Wu and Y. Liu, Nanoscale, 2018, 10, 15932–15937 RSC.
- C. Guo, F. Su, Y. Song, B. Hu, M. Wang, L. He, D. Peng and Z. Zhang, ACS Appl. Mater. Interfaces, 2017, 9, 41188–41199 CrossRef CAS.
- X. Huang, M. Lan, J. Wang, L. Guo, Z. Lin, N. Sun, C. Wu and B. Qiu, Biosens. Bioelectron., 2020, 169, 112655 CrossRef CAS.
- K. Pyo, V. D. Thanthirige, K. Kwak, P. Pandurangan, G. Ramakrishna and D. Lee, J. Am. Chem. Soc., 2015, 137, 8244–8250 CrossRef CAS.
- J. Luo, Z. Xie, J. W. Y. Lam, L. Cheng, H. Chen, C. Qiu, H. S. Kwok, X. Zhan, Y. Liu, D. Zhu and B. Z. Tang, Chem. Commun., 2001, 1740–1741, 10.1039/B105159H.
- G. Yu, S. Yin, Y. Liu, J. Chen, X. Xu, X. Sun, D. Ma, X. Zhan, Q. Peng, Z. Shuai, B. Tang, D. Zhu, W. Fang and Y. Luo, J. Am. Chem. Soc., 2005, 127, 6335–6346 CrossRef CAS.
- J. Mei, N. L. C. Leung, R. T. K. Kwok, J. W. Y. Lam and B. Z. Tang, Chem. Rev., 2015, 115, 11718–11940 CrossRef CAS.
- Z. Zhao, B. He and B. Z. Tang, Chem. Sci., 2015, 6, 5347–5365 RSC.
- H. Zhang, Z. Zhao, P. R. McGonigal, R. Ye, S. Liu, J. W. Y. Lam, R. T. K. Kwok, W. Z. Yuan, J. Xie, A. L. Rogach and B. Z. Tang, Mater. Today, 2020, 32, 275–292 CrossRef CAS.
- Z. Wu, Q. Yao, O. J. H. Chai, N. Ding, W. Xu, S. Zang and J. Xie, Angew. Chem., 2020, 59, 9934–9939 CrossRef CAS.
- Z. Luo, X. Yuan, Y. Yu, Q. Zhang, D. T. Leong, J. Y. Lee and J. Xie, J. Am. Chem. Soc., 2012, 134, 16662–16670 CrossRef CAS.
- Z. N. Wu, Q. F. Yao, S. Q. Zang and J. P. Xie, Natl. Sci. Rev., 2021 DOI:10.1093/nsr/nwaa208.
- Z. Han, Y. Zhang, Y. Wu, Z. Li, L. Bai, S. Huo and X. Lu, Anal. Chem., 2019, 91, 8676–8682 CrossRef CAS.
- Y. Zhou, Y. Chai and R. Yuan, Anal. Chem., 2019, 91, 14618–14623 CrossRef CAS.
- F. Yang, X. Y. Jiang, W. B. Liang, Y. Q. Chai, R. Yuan and Y. Zhuo, Anal. Chem., 2020, 92, 2566–2572 CrossRef CAS.
- Y. Wang, Y. H. Liu, Y. Zhang, F. Wang, P. J. Kowalski, H. W. Rohrs, R. A. Loomis, M. L. Gross and W. E. Buhro, Angew. Chem., Int. Ed., 2012, 51, 6154–6157 CrossRef CAS.
- Y. Wang, Y. Zhang, F. Wang, D. E. Giblin, J. Hoy, H. W. Rohrs, R. A. Loomis and W. E. Buhro, Chem. Mater., 2014, 26, 2233–2243 CrossRef CAS.
- N. Park, M. Monahan, A. Ritchhart, M. R. Friedfeld and B. M. Cossairt, J. Visualized Exp., 2019, 147, e59425 Search PubMed.
- H. Zhu, R. Jin, D. Jiang and J.-J. Zhu, ACS Appl. Mater. Interfaces, 2019, 11, 46666–46670 CAS.
- G. Liu, B.-K. Jin, C. Ma, Z. Chen and J.-J. Zhu, Anal. Chem., 2019, 91, 6363–6370 CrossRef CAS.
- Y. Chen, J. Fu, C. Cui, D. Jiang, Z. Chen, H.-Y. Chen and J. J. Zhu, Anal. Chem., 2018, 90, 8635–8641 CrossRef.
|
This journal is © The Royal Society of Chemistry 2021 |