DOI:
10.1039/D3QI00910F
(Research Article)
Inorg. Chem. Front., 2023,
10, 5584-5590
Facile access to mid-valent Group 5 and 6 metal synthons†
Received
17th May 2023
, Accepted 18th June 2023
First published on 19th June 2023
Abstract
Group 5 and 6 metal chlorides, MClx (M = Nb, Ta, Mo, W) are easily and controllably reduced, in a stepwise fashion, by stoichiometric PhMe2SiH, yielding only PhMe2SiCl, a useful reagent, and H2 as the byproducts. Addition of n moles of PhMe2SiH to toluene solutions of MClx yields stepwise uncoordinated reduction products of the form MClx−n (M = Nb, Ta, Mo, W; x = 5 for Nb, Ta, Mo, x = 6 for W; n = 1 for Nb and Ta, n = 1, 2 for Mo and W). The reactions proceed cleanly furnishing quantitative, analytically pure yields of the desired mid-valent binary chlorides. The obtained products are very reactive and can be further derivatized with coordinating ethers or phosphines for the on-demand preparation of desired inorganic synthons.
Introduction
When a specific oxidation state is targeted for the synthesis of a transition metal complex, it is typically preferred to begin with a precursor of that oxidation state and follow simple ligation or metathesis type routes to install the desired ligand framework. This necessitates either the availability of stable but reactive commercial precursors of variable oxidation states, typically halide salts, or facile routes to access them synthetically. An area of particular interest are the group 5 and 6 metals which can exist in a wide range of oxidation states, e.g., Mo displays states covering 0, +1, +2, +3, +4, +5, and +6. Therein, and germane to the content of this report, Nb, Ta, Mo, and W have drawn decades worth of interest for the synthesis of coordination compounds in service of fundamental structural chemistry,1 catalysis,2 and biomimetics.3 More recently with a growing interest in vapor-phase growth processes (chemical vapor deposition and atomic layer deposition)4 for the fabrication of metal oxides and 2-D semiconductor transition metal dichalcogenides,5 the demand for group 56 and 67 molecular precursors with diverse oxidation states and ligand topologies has increased. The synthesis of these molecular precursors, and the aforementioned coordination compounds is reliant on the availability of their MClx (M = Nb, Ta, Mo, W) synthons. Unfortunately, for the group 5 and 6 metals, commercial availability of mid-valent halides is comparatively sparse compared to high- and low-valent analogues. Correspondingly, those which are available are often significantly more costly, and can often be less reactive due to a combination of their polymeric nature, and/or high degree of crystallinity stemming from their preparative routes.
Over the past seven decades, a variety of routes targeting mid-valent early transition metal chlorides have been reported; encompassing both high temperature and in situ reduction protocols (Scheme 1).8 The high temperature routes allow for the straightforward preparation of the desired oxidation state. However, resultant of the high temperature process, these materials are typically highly crystalline and often display lower reactivity. Conversely, as the behaviour of high-valent transition metal chlorides is generally well-understood, in situ reductions to the desired oxidation state become more appealing. They can be carried out via both heterogeneous or homogeneous reducing agents, and in the presence of a coordinating solvent or ligand to capture the desired product as a discreet molecular complex. A perfect example of this is the diethyl ether adduct of molybdenum(IV) chloride, MoCl4(Et2O)2. Heterogenous tin metal8c and homogenous allyltrimethylsilane9 are both capable of reducing MoCl5 in diethyl ether, leading to the formation of MoCl4(Et2O)2. A downside to these in situ reactions is that strict control of reaction conditions is necessary to prevent unwanted side/by-products, e.g., the formation of MoOCl3(Et2O)2.9,10
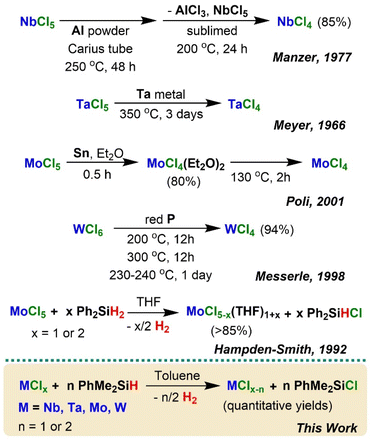 |
| Scheme 1 Select examples of common group 5 and 6 MClx reduction processes. | |
Alternatively, amorphous, uncoordinated MClx can be prepared via reductions of high-valent starting materials, in non-coordinating solvents, with heterogenous reducing agents, e.g., the preparation of amorphous WCl4via reduction of WCl6 with tin metal.8d Although reliable, such methods utilize tin metal as the reductant which generates chlorinated tin waste. In general, most in situ reductions necessitate reagents that are either difficult to handle (pyrophoric, toxic) and/or generate unwanted by-products (metal/salt wastes). An elegant potential solution was first reported by Hampden-Smith in 1992, whereby MoCl5 was reacted with Ph2SiH2 under mild conditions in thf to generate MoCl4(thf)2 and Ph2SiHCl.
This process utilizes a common organo-reductant that is easy to handle and yields the desired product with a readily separated chlorosilane as by-product; the latter is also synthetically useful. Unfortunately, this methodology has not been expounded on adequately in the decades since its first report. We surmise that this is likely due to the lower importance given to green chemistry at the time of publication, and looser restrictions placed on use of hazardous reagents in both industrial, academic, and national laboratory settings.
Leveraging this finding, our group recently reported the use of commercially available PhMe2SiH, as a stoichiometric reducing agent for the preparation of dimeric Mo(III) synthons.11 Herein, we report an expansion of this process as a general methodology for the reduction of high-valent Group 5 and 6 transition metal chlorides (Scheme 1). Our approach requires mild reaction conditions and generates highly reactive, uncoordinated reduced metal chlorides as a precipitate for facile separation, and only PhMe2SiCl as by-product; itself a very useful reagent for a variety of processes.12 Critically, the degree of reduction is readily controlled by reductant stoichiometry: 1e− for 1 equiv. of PhMe2SiH. To the best of our knowledge there are no examples of uncoordinated transition metal halides being formed and isolated in a similar manner. Inspired by the reduction of MoCl4(dme) to ‘MoCl3(dme)’ (dme = dimethoxyethane) using PhMe2SiH, we sought to explore the generality of this reduction process with other Mo, W, Nb, and Ta chlorides, and target the generation of their uncoordinated mid-valent products.
Experimental
Materials
All chemical manipulations were performed using standard glovebox and Schlenk techniques in ultra-high purity (UHP) nitrogen environments. Basic alumina (50–200 μm) was purchased from Acros Organics and activated by heating to 220 °C under vacuum (10−2 Torr) for 12 h. Molecular sieves were purchased from Fisher Scientific and dried at 150 °C for 24 h prior to use. Diethyl ether, hexanes, and toluene were collected from an mBraun solvent purification system (SPS) and stored over basic alumina for 12 h in the glovebox prior to use. Dimethoxyethane (dme) was purchased from Sigma-Aldrich and dried over activated basic alumina for 12 h prior to use. Molybdenum pentachloride (99.6%), tungsten hexachloride (99.9%-W), niobium pentachloride (99+%-Nb), and tantalum pentachloride (99.9%-Ta) were purchased and used as received from STREM Chemicals, Inc. Dimethylphenylsilane (≥98%) was purchased from Sigma-Aldrich and stored over activated 3 Å molecular sieves for 12 h prior to use. Benzene-d6 was purchased from Sigma-Aldrich and was dried over activated basic alumina for 12 h prior to use. Triethylphosphine (99%) was purchased and used as received from STREM Chemicals, Inc.
Characterization
The purity of organic reagents was checked via1H NMR. Elemental analyses (C, H, Cl) were performed by Galbraith Laboratories, Inc. (Knoxville, TN). 1H NMR spectra were recorded on a Bruker Avance III HD 600 instrument equipped with a cryoprobe and were processed using Mestrelab's MestReNova software. Spectra were referenced to residual C6D5H (1H δ 7.16).
General preparation of MCl4
When starting from an MCl5 precursor, a typical experiment proceeded as follows: inside the glovebox, an Erlenmeyer flask was charged with 1 g of the commercial grade MCl5, a stir bar, and approximately 25 ml of toluene. Whilst stirring, 1 molar equivalent of dimethylphenylsilane was introduced by syringe. The flask was then capped with a rubber septum, which was then punctured with an 18-gauge needle to function as a ventilation port for the H2 gas by-product. In the case of preparing WCl4 from WCl6, the only experimental modification is the addition of 2 molar equivalents of dimethylphenylsilane. The reactions were then left to stir overnight. The preparation of MoCl4 is an exception, where complete conversion occurs in approximately 1 h. The next day, the resulting suspensions were filtered through fine porosity sintered glass frits and washed with approximately 25 mL of fresh toluene. Then, the collected solid was washed with approximately 25 mL of hexanes and dried in vacuo for 0.5 hours. The resulting yields were quantitative, with slight deviations due to mechanical loss. Elemental Analyses of each MCl4 product are as follows: Anal. Calcd for NbCl4: Cl, 60.42. Found: Cl, 60.38; Anal. Calcd for TaCl4: Cl, 43.94. Found: Cl, 43.48; Anal. Calcd for MoCl4: Cl, 59.64. Found: Cl, 59.69; Anal. Calcd for WCl4: Cl, 43.55. Found: Cl, 43.45. Species-specific observations can be found in the ESI.†
Preparation of MoCl3
Addition of two equivalents of dimethylphenylsilane to the yellow-brown suspension of MoCl5 in toluene results in the immediate, visual release of H2 and the precipitation of MoCl4. If left to sit with no stirring, the reduction of MoCl4 to MoCl3 does not proceed. However, with vigorous stirring, MoCl4 is fully reduced to MoCl3 which is recovered as a dark, red-brown solid. This reduction is also achievable via the addition of one equivalent of dimethylphenylsilane to MoCl4 prepared via the silane reduction route described above. Anal. Calcd for MoCl3: Cl, 52.57. Found: Cl, 52.66.
Preparation of WCl5
Addition of one equivalent of dimethylphenylsilane to a stirred, royal blue suspension of WCl6 in toluene results in the immediate evolution of H2. This reaction is rapid, and amorphous, black WCl5 settles to the bottom of the reaction vessel and a clear supernatant is observed. Anal. Calcd for WCl5: Cl, 49.09. Found: Cl, 48.73.
Preparation of MCl4(PEt3)2 (M = Nb, Ta)
0.5 g of starting MCl4 powder was suspended in approximately 50 mL of dry toluene inside of a Schlenk flask equipped with a stir bar. This suspension was then cycled onto the Schlenk line and kept under a UHP N2 environment. While under direct nitrogen flow, 2 equivalents (0.63 mL for NbCl4, 0.46 mL for TaCl4) of triethylphosphine were syringed into the reaction vessel at room temperature and the reaction was allowed to stir overnight. The next day, the reaction was filtered on the Schlenk line through a freshly dried, fine porosity swivel frit to remove any insoluble materials. The obtained dark, red-yellow (Nb) and clear, yellow-green (Ta) solutions were then stripped under reduced pressure, which afforded 0.65 g of dark red NbCl4(PEt3)2 (65%) and 0.43 g of beige TaCl4(PEt3)2 (50%). Anal. Calcd for NbCl4P2C12H30: Cl, 30.10; C, 30.60; H, 6.42. Found: Cl, 30.03; C, 30.22; H, 6.12. Anal. Calcd for TaCl4P2C12H30: Cl, 25.36; C, 25.78; H, 5.41. Found: Cl, 25.64; C, 24.63; H, 4.53. C, H analyses were conducted in the presence of a combustion agent (V2O5) to obtain reliable data.
Preparation of MoCl4(dme)
0.4 g of MoCl4 powder were added to a 22 mL scintillation vial, along with a freshly dried stir bar. Then, approximately 10 mL of dry dme were added and the mixture was stirred for 1 h. At the end of the period, a homogenous solution was obtained. This solution was then treated with an equal volume of dry hexanes which resulted in the precipitation of a dull orange powder. The powder was then isolated via vacuum filtration and washed with fresh hexanes and dried in vacuo, yielding 0.52 g (95%) of MoCl4(dme). Purity was assessed via1H NMR in d8-toluene: δ 16.32 (s, 6H), 1.17 (s, 4H).
Preparation of WCl4(dme)
2 g of WCl4 powder were charged to a freshly dried 125 mL Erlenmeyer flask equipped with a stir bar. Approximately 45 mL of dry dme was then added to the flask, which was then capped with a glass stopper and stirred overnight. The next day, the green reaction mixture was passed through a 30 mL fine porosity sintered glass frit charged with a quarter volume of dried Celite, via vacuum filtration, to remove any unreacted WCl4. The Celite filter cake was then washed with 2 × 10 mL of fresh dme to recover any adduct left behind. The obtained emerald-green filtrate was then transferred to a freshly dried 100 mL Schlenk flask and cycled onto the Schlenk line. The solution is then stripped under reduced pressure with gentle heating to afford 2.17 g of a brown solid. Yield: 85%. The isolated material is then ready for employment in subsequent syntheses. Purity was assessed via1H NMR in d8-toluene: δ 9.93 (s, 6H), 0.77 (s, 4H).
Single crystal X-ray diffraction
Detailed tables of crystallographic data, structural refinement information, and bond lengths and angles can be found in the ESI.† Single crystals of NbCl4(dme) were obtained by creating a concentrated solution of NbCl4 in dry dme and setting up a vapor diffusion crystallization with hexanes as the counter solvent. Over a period of two days, well-formed, orange needles precipitated from the solution. Single crystals of NbCl4(Et2O)2 were obtained by saturating a solution of diethyl ether with NbCl4. The resulting orange solution was then passed through a filter plug made of glass wool and Celite to remove any insoluble material. The homogenous solution was then chilled in a −35 °C freezer over a period of two days and orange, block-like crystals formed. Single crystals of TaCl4(Et2O)2 and Ta2Cl8(Et2O)2 were obtained via the dissolution of TaCl4 in minimal, dry diethyl ether. The resulting teal-green solution was then placed in the freezer and two crystalline morphologies precipitated over a period of 30 minutes. Lime green needles corresponding to TaCl4(Et2O)2 and teal needles corresponding to Ta2Cl8(Et2O)2 were obtained. Single crystals of [Ta2Cl6(dme)2][TaCl6] were obtained by the addition of minimal dme to TaCl4. The resulting suspension was filtered through a filter plug consisting of glass wool and Celite to obtain a homogenous, dark blue solution. The solution was then placed in a −35 °C freezer and left to sit overnight, during which time yellow blocks and thin, blue plates corresponding to [Ta2Cl6(dme)2][TaCl6] precipitated. X-ray diffraction studies of NbCl4(dme) were conducted on a XtaLAB Synergy, single source at offset/far, HyPix diffractometer with Mo Kα (λ = 0.7103 Å) micro-focus sealed X-ray tube PhotonJet source and a mirror detector at 100(1) K. X-ray diffraction studies of NbCl4(Et2O)2, TaCl4(Et2O)2, Ta2Cl8(Et2O)2, and [Ta2Cl6(dme)2][TaCl6] were conducted on a Rigaku XTA-Lab Mini II diffractometer using a Mo Kα (λ = 0.7103 Å) source and a CCD plate detector at 100(1) K. Data collection, cell parameter determinations, data reduction, and absorption corrections were performed via CrysAlis Pro.13 Structure solution, refinement, and publication materials were generated via SHELXL, SHELXLT, and Olex2.14–16 All hydrogen atoms were attached via the riding model at calculated positions.
Results and discussion
Synthesis
The addition of PhMe2SiH to small quantities of crystalline MoCl5, partially dissolved in toluene to form a brown-yellow suspension, resulted in the vigorous evolution of gas and the precipitation of a black powder with a clear supernatant. A droplet of the supernatant was then suspended in d8-toluene and analysed by 1H NMR. The septet at 4.42 ppm, corresponding to the hydridosilane hydride, had disappeared and the doublet at 0.21 ppm, corresponding to the methyl groups collapsed to a singlet and shifted to 0.43 ppm, indicating the formation of the chlorinated by-product, PhMe2SiCl (Scheme 2i). The formation of the chlorinated by-product and the vigorous evolution of gas lead us to believe that this process proceeds via the reductive elimination of H2 (vide infra). Elemental analysis of the isolated and dried product confirmed that we had made analytically pure MoCl4. This process was then scaled to multigram quantities, with 5 grams of starting MoCl5, and left to react overnight which ultimately yielded 4.14 g (95%) of MoCl4. Any unreacted MoCl5 can be removed by washing with anhydrous dichloromethane or anhydrous toluene.
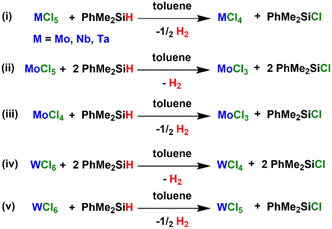 |
| Scheme 2 List of demonstrated 1e− and 2e− MClx (M = Mo, W, Nb, Ta) reduction processes with stoichiometric PhMe2SiH. | |
Next, we sought to understand the extent of reduction, and the degree of stoichiometric control available with PhMe2SiH. Reaction of MoCl5 with 2 equiv. of PhMe2SiH, or a suspension of isolated MoCl4 (vide supra) with 1 equiv. of PhMe2SiH accompanied by vigorous stirring overnight yielded dark red MoCl3 (Scheme 2ii and iii). Notably, the MoCl4 → MoCl3 sequence is not accompanied by vigorous gas evolution, indicating a slower reaction rate.
Both reaction sequences were conducted on the gram scale affording near quantitative yields with high purity product as confirmed by elemental analysis (see Experimental section and ESI†).
The isolated MoCl4 can be used to obtain solvated complexes. As an example, MoCl4 was suspended in dme and stirred for one hour, furnishing MoCl4(dme) in 95% yield (Scheme 3i). The silane can also be employed as a reducing agent for previously reported Mo-based systems: the syntheses of MoCl4(Et2O)2 and MoCl3(thf)3 in lieu of Me3Si(C3H5) and Ph2SiH2, respectively. For MoCl4(Et2O)2, a comparable yield of 86% is obtained in 30 min vs. the reported 2 h.9 A 70% yield of MoCl3(thf)3 is obtained in 1 h, vs. the reported 85% yield in 4 h.8e
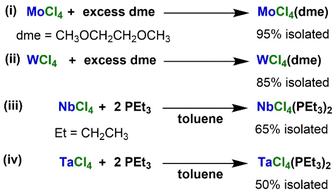 |
| Scheme 3 Derivatization reactions for amorphous MCl4 (M = Nb, Ta, Mo, W) to form MCl4(PEt3)2 (M = Nb, Ta) and MCl4(dme) (M = Mo, W). | |
Next, we targeted the synthesis of WCl4. Starting with WCl6 partially dissolved in toluene, stepwise reductions were performed with stoichiometric amounts of PhMe2SiH to obtain analytically pure WCl5 and WCl4, respectively, in essentially quantitative yields (Scheme 2iv and v). The reduction of WCl6 to WCl5 is complete in 2 h while WCl6 to WCl4 reduction was left to react overnight. We note here that WCl6 is not reduced to WCl3 with 3 equiv. of PhMe2SiH. As with Mo, we utilized the as synthesized WCl4 to obtain WCl4(dme) in 86% yield (2 g scale, Scheme 3ii).
We then shifted our attention to Nb and Ta chlorides. The only commercially available chlorides are MCl5 and, to the best of our knowledge, preparative routes to uncoordinated lower oxidation states are limited to high-temperature processes (Scheme 1). These are energy intensive, lengthy, and require further purification. Employing our reduction strategy, small quantities of NbCl5 and TaCl5 were added to toluene which resulted in dark orange-red and golden-yellow solutions, respectively. We then added excess PhMe2SiH; in this instance vigorous gas evolution was not observed. We left the reactions to sit overnight and returned to brown NbCl4 and olive-green TaCl4, suspended in colourless supernatants, which were isolated and determined to be analytically pure. The reactions were repeated on the 5 g scale, resulting in a 96% yield of both NbCl4 and TaCl4. We note that our obtained olive-green TaCl4 differs from the reported orange TaCl4 intermediate obtained by Hayton et al.17via the addition of triethylsilane to TaCl5 but matches the reported olive-green TaCl4 obtained by Manzer via the high-temperature reduction of TaCl5 with Al powder.8a Efforts to reduce the Nb and Ta chlorides below the (IV) oxidation state were unsuccessful, even with mild heating or vigorous stirring.
To probe the utility of our amorphous NbCl4 and TaCl4 powders, we sought to form the established bis(triethyl phosphine) adducts. Both binary halides were suspended in toluene and two equivalents of triethylphosphine (PEt3) were introduced at room temperature (Scheme 3iii and iv). Both trans-bis(triethylphosphine) complexes were formed, with 65% yield for Nb and 50% yield for Ta, comparable with reported yields of 76% and 48%, respectively.18 Next, we examined the reactivity of our NbCl4 and TaCl4 with ethereal solvents, with the goal of developing new synthons. While there are examples of mid-valent Nb and Ta complexes with N, S, P, and Se donor ligands, the libraries of neutral, ether-coordinated, non-oxo Nb and Ta synthons are limited.19
The addition of NbCl4 to cold (−35 °C) dme produced an orange solution, which readily precipitated bright orange NbCl4(dme) in near quantitative yields upon addition of hexanes. Addition of NbCl4 to thf and diethyl ether also produce the desired NbCl4(thf)2 and NbCl4(Et2O)2 adducts, respectively. Single crystal X-ray structures were readily obtained (Fig. 1A and B). These routes now provide one-step access to ethereal NbCl4L2 synthons directly from NbCl4. The reaction of as prepared TaCl4 with room temperature diethyl ether resulted in the immediate formation of a teal solution, from which two crystalline morphologies precipitated in ∼30 min at −35 °C. The first were lime green needles that were determined to be the monomeric trans-TaCl4(Et2O)2 (Fig. 1C). The second were teal needles determined to be dimeric Ta2Cl8(Et2O)2 (Fig. 1D). The addition of TaCl4 to cold dme results in the immediate formation of a blue solution. Filtration and storage of the solution at −35 °C precipitated blue crystals found to be [Ta2Cl6(dme)2][TaCl6] (Fig. 1E) and yellow crystals yet to be structurally characterized. The Ta–Cl distances of the [TaCl6]− anion closely align with those of previously reported sodium hexachlorotantalate(V),20 pointing towards the formation of a mixed valent, Ta(III)–Ta(IV) dimer stabilized by a Ta(V) counter ion. At present, we are calling this a dme-induced disproportionation; further studies will be required to fully elucidate this reaction.
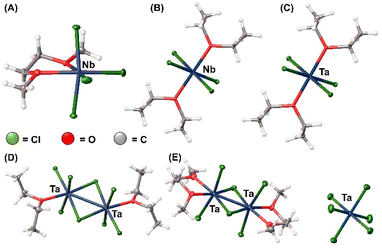 |
| Fig. 1 Single crystal X-ray structures of (A) NbCl4(dme), (B) NbCl4(Et2O)2, (C) TaCl4(Et2O)2, (D) [TaCl4(Et2O)]2, and (E) [TaCl3(dme)]2[TaCl6]. Thermal ellipsoids drawn at 50%; for bond lengths and angles, see ESI.† | |
The results highlighted herein provide a validation of the high reactivity of the in situ reduced uncoordinated metal salts and offer a proof-of-concept for potential applicability.
Conclusions
In summary, we have developed a benign, facile, solution-based route for the on-demand preparation of Group 5 and 6 mid-valent binary chlorides. This process employs the use of commercially available PhMe2SiH and affords analytically pure, quantitative yields of the desired mid-valent chloride on a short time scale. The work-up procedure for each reaction is simple and requires no further separation of heterogenous by-products. These mid-valent chlorides are highly reactive and can be leveraged to reproduce classic inorganic synthons or build towards new species as exemplified by our preliminary findings on etherates. Ongoing work is focused on elucidating the latter and expanding the scope of this process beyond Groups 5 and 6.
Author contributions
T. E. Shaw: investigation, methodology, formal analysis, writing – original draft, writing – reviewing & editing. C. L. Stern: investigation, formal analysis. A. P. Sattelberger: supervision, methodology, conceptualization, formal analysis, project administration, funding acquisition, writing – original draft, writing – reviewing & editing. T. Jurca: supervision, methodology, conceptualization, formal analysis, project administration, funding acquisition, writing – original draft, writing – reviewing & editing.
Conflicts of interest
There are no conflicts to declare.
Acknowledgements
This work was supported by the Department of Chemistry, College of Sciences, and the Faculty Cluster Initiative at the University of Central Florida. The use of the IMSERC Crystallography facility at Northwestern University, which has received support from the Soft and Hybrid Nanotechnology Experimental (SHyNE) Resource (NSF ECCS-2025633), and Northwestern University, is gratefully acknowledged. The authors acknowledge insightful input from Drs. Brian L. Scott and Samuel M. Greer (Los Alamos National Lab) and Professor Thomas M. Gilbert (Northern Illinois University).
References
- D. Singh, W. R. Buratto, J. F. Torres and L. J. Murray, Activation of Dinitrogen by Polynuclear Metal Complexes, Chem. Rev., 2020, 120, 5517–5581 CrossRef CAS PubMed.
- C. Coperet, A. Comas-Vives, M. P. Conley, D. P. Estes, A. Fedorov, V. Mougel, H. Nagae, F. Nunez-Zarur and P. A. Zhizhko, Surface Organometallic and Coordination Chemistry toward Single-Site Heterogeneous Catalysts: Strategies, Methods, Structures, and Activities, Chem. Rev., 2016, 116, 323–421 CrossRef CAS PubMed.
- S. Groysman and R. H. Holm, Biomimetic Chemistry of Iron, Nickel, Molybdenum, and Tungsten in Sulfur-Ligated Protein Sites, Biochemistry, 2009, 48, 2310–2320 CrossRef CAS PubMed.
-
(a) J. Lu, J. W. Elam and P. C. Stair, Atomic layer deposition-Sequential self-limiting surface reactions for advanced catalyst “bottom-up” synthesis, Surf. Sci. Rep., 2016, 410–472 CrossRef CAS;
(b) T. J. Kunene, L. K. Tartibu, K. Ukoba and T.-C. Jen, Review of atomic layer deposition process, application and modelling tools, Mater. Today: Proc., 2022, 62, S95–S109 Search PubMed.
- J. Cai, X. Han, X. Wang and X. Meng, Atomic Layer Deposition of Two-Dimensional Layered Materials: Processes, Growth Mechanisms, and Characteristics, Matter, 2020, 2, 587–630 CrossRef.
-
(a) K. Khumaini, H. Roh, H. Han, H.-L. Kim, H.-S. Kim, J.-H. Seok, J. W. Park and W.-J. Lee, Surface reaction mechanism of atomic layer deposition of niobium oxide: In situ characterization and first-principle study, Appl. Surf. Sci., 2023, 615, 156340 CrossRef CAS;
(b) S. J. Song, T. Park, K. J. Yoon, J. H. Yoon, D. E. Kwon, W. Noh, C. Lansalot-Matras, S. Gatineau, H.-K. Lee, S. Gautam, D.-Y. Cho, S. W. Lee and C. S. Hwang, Comparison of the Atomic Layer Deposition of Tantalum Oxide Thin Films Using Ta(NtBu)(NEt2)3, Ta(NtBu)(NEt2)2Cp, and H2O, ACS Appl. Mater. Interfaces, 2017, 9, 537–547 CrossRef CAS PubMed.
-
(a) T. Jurca, M. J. Moody, A. Henning, J. D. Emery, B. Wang, J. M. Tan, T. L. Lohr, L. J. Lauhon and T. J. Marks, Low-Temperature Atomic Layer Deposition of MoS2 Films, Angew. Chem., Int. Ed., 2017, 56, 4991–4995 CrossRef CAS PubMed;
(b) M. Mattinen, J.-L. Wree, N. Stegmann, E. Ciftyurek, M. El Achhab, P. J. King, K. Mizohata, J. Raisanen, K. D. Schierbaum, A. Devi, M. Ritala and M. Leskela, Atomic Layer Deposition of Molybdenum and Tungsten Oxide Thin Films Using Heteroleptic Imido-Amidinato Precursors: Process Development, Film Characterization, and Gas Sensing Properties, Chem. Mater., 2018, 30, 8690–8701 CrossRef CAS.
-
(a) L. E. Manzer, Preparation of the paramagnetic alkyls bis(cyclopentadienyl)dimethylniobium and bis(methylcyclopentadienyl)dimethyltantalum and some six- and eight-coordinate phosphine derivatives of niobium(IV), Inorg. Chem., 1977, 16, 525–528 CrossRef CAS;
(b) B. Bajan and H.-J. Meyer, Crystal structure of tantalum tetrachloride, TaCl4, Z. Kristallogr. – Cryst. Mater., 1996, 211, 818 CrossRef CAS;
(c) F. Stoffelbach, D. Saurenz and R. Poli, Improved Preparations of Molybdenum Coordination Compounds from Tetrachlorobis(diethyl ether)molybdenum(IV), Eur. J. Inorg. Chem., 2001, 2699–2703 CrossRef CAS;
(d) V. Kolesnichenko, D. C. Swenson and L. Messerle, Facile Reduction of Tungsten Halides with Nonconventional, Mild Reductants. I. Tungsten Tetrachloride: Several Convenient Solid-State Syntheses, a Solution Synthesis of Highly Reactive (WCl4)x, and the Molecular Structure of Polymeric Tungsten Tetrachloride, Inorg. Chem., 1998, 37, 3257–3262 CrossRef CAS;
(e) D. Zeng and M. J. Hampden-Smith, High yield routes to molybdenum(III) compounds by diphenylsilane and tin(II) chloride reduction of molybdenum(V) and molybdenum(IV) chlorides, Polyhedron, 1992, 11, 2585–2589 CrossRef CAS.
- C. Persson and C. Andersson, Reduction of tungsten(VI) and molybdenum(V) by allyltrimethylsilane and cyclopentene. Simple high yield syntheses of MoCl4(OEt2)2, MoCl4(dme), WCl4(thf)2, WCl4(dme), and WOCl3(thf)2, Inorg. Chim. Acta, 1993, 203, 235–238 CrossRef CAS.
-
(a) L. Castellani and M. C. Gallazzi, Facile preparation of diethyl ether complexes of molybdenum and tungsten tetrachloride, Transition Met. Chem., 1985, 10, 194–195 CrossRef CAS;
(b) T. E. Shaw, T. J. Diethrich, C. L. Stern, B. L. Scott, T. Jurca, T. M. Gilbert and A. P. Sattelberger, Synthesis, characterization, X-ray and electronic structures of diethyl ether and 1,2-dimethoxyethane adducts of molybdenum(IV) chloride and tungsten(IV) chloride, Dalton Trans., 2022, 51, 7856–7863 RSC;
(c) T. E. Shaw, P. LeMagueres, A. P. Sattelberger and T. Jurca, Crystal structure and Hirshfeld surface analysis of the elusive trichlorobis(diethyl ether)oxomolybdenum(V), Acta Crystallogr., Sect. C: Struct. Chem., 2020, 76, 947–951 CrossRef CAS PubMed.
- T. E. Shaw, T. J. Diethrich, B. L. Scott, T. M. Gilbert, A. P. Sattelberger and T. Jurca, “MoCl3(dme)” Revisited: Improved Synthesis, Characterization, and X-ray and Electronic Structures, Inorg. Chem., 2021, 60, 12218–12225 CrossRef CAS PubMed.
-
(a) P. Deglmann, E. Ember, P. Hofmann, S. Pitter and O. Walter, Experimental and Theoretical Investigations on the Catalytic Hydrosilylation of Carbon Dioxide with Ruthenium Nitrile Complexes, Chem. – Eur. J., 2007, 13, 2864–2879 CrossRef CAS PubMed;
(b) K. Izod, C. Wills, W. Clegg and R. W. Harrington, Acyclic Dialkylstannylene and -Plumbylene Compounds That Are Monomeric in the Solid State, Organometallics, 2009, 28, 5661–5668 CrossRef CAS;
(c) X. Zhang and Z. Cheng, Performance of combined use of chlorosilanes and AlCl3 in the carboxylation of toluene with CO2, AIChE J., 2016, 63, 185–191 CrossRef.
-
Rigaku Oxford Diffraction, CrysAlis PRO, Rigaku Oxford Diffraction, Yarnton, Oxforshire, England, 2020 Search PubMed.
-
SHELXTL, ver. 6.12, Bruker AXS, Inc., Madison, WI, 2005 Search PubMed.
- Olex 2 1.2 (compiled 2014-06-27 svn.r2953 for OlexSys, GUI svn. r4855).
- O. Dolomanov, L. Bourhis, R. Gildea, J. Howard and H. Puschmann, OLEX2: a complete structure solution, refinement and analysis program, J. Appl. Crystallogr., 2009, 42, 339–341 CrossRef CAS.
- P. L. Damon, C. J. Liss, R. A. Lewis, S. Morochnik, D. E. Szpunar, J. Telser and T. W. Hayton, Quantifying the Electron Donor and Acceptor Abilities of the Ketimide Ligands in M(N=CtBu2)4 (M = V, Nb, Ta), Inorg. Chem., 2015, 54, 10081–10095 CrossRef CAS PubMed.
-
(a) F. A. Cotton, S. A. Duraj and W. J. Roth, Further studies of the phosphine complexes of niobium(IV) chloride, Inorg. Chem., 1984, 23, 3592–3596 CrossRef CAS;
(b) F. A. Cotton, S. A. Duraj and W. J. Roth, Preparation and Structural Characterization of Phosphine Adducts of Tantalum(IV) Chloride, Inorg. Chem., 1984, 23, 4046–4050 CrossRef CAS.
-
(a) E. J. Roskamp and S. F. Pedersen, The first practical niobium(III) reagent in organic synthesis. A convenient route to 2-amino alcohols via the coupling of imines with aldehydes or ketones promoted by NbCl3(DME), J. Am. Chem. Soc., 1987, 109, 6551–6553 CrossRef CAS;
(b) L. G. Hubert-Pfalzgraf, M. Tsunoda and J. G. Riess, Synthesis and characterization of niobium(III) trichloride ether and sulfide derivatives, Inorg. Chim. Acta, 1980, 41, 283–286 CrossRef CAS;
(c) G. Bresciani, S. Zacchini, F. Marchetti and G. Pampaloni, Non-precious metal carbamates as catalysts for the aziridine/CO2 coupling reaction under mild conditions, Dalton Trans., 2021, 50, 5351–5359 RSC;
(d) R. Bini, F. Marchetti, G. Pampaloni and S. Zacchini, Further insights into the chemistry of niobium and tantalum pentahalides with 1,2-dialkoxyalkanes: Synthesis of bromo-and iodoalkoxides, spectroscopic and computational studies, Polyhedron, 2011, 30, 1412–1419 CrossRef CAS;
(e) R. Bini, C. Chiappe, F. Marchetti, G. Pampaloni and S. Zacchini, Structures and Unusual Rearrangements of Coordination Adducts of MX5 (M = Nb, Ta; X = F, Cl) with Simple Diethers. A Crystallographic, Spectroscopic, and Computational Study, Inorg. Chem., 2010, 49, 339–351 CrossRef CAS PubMed.
- H. Henke, Zur kristallchemischen Einordnung von NaSbCl6, NaNbCl6, und NaTaCl6, Z. Kristallogr., 1992, 198, 1–16 CrossRef CAS.
|
This journal is © the Partner Organisations 2023 |