DOI:
10.1039/D1RA07173D
(Review Article)
RSC Adv., 2021,
11, 36023-36033
Chemistry and biological activities of hetisine-type diterpenoid alkaloids†
Received
25th September 2021
, Accepted 17th October 2021
First published on 8th November 2021
Abstract
Hetisine-type C20-diterpenoid alkaloids (DAs) are one of the most important DA subtypes. During the past decades, a total of 157 hetisine-type DAs were obtained from plants from seven genera in three families, most of which were isolated from the genera Aconitum and Delphinium in the Ranunculaceae family. Structurally, hetisine-type DAs are characterized by a heptacyclic hetisane skeleton formed by the linkage of C(14)–C(20) and N–C(6) bonds in an atisine-type DA, and their structural diversity is created by the states of the N atom and various substituents. Pharmacological studies have revealed a wide range of pharmacological actions for hetisine-type DAs, including antiarrhythmic, antitumor, antimicrobial and insecticidal activities, as well as effects on peripheral vasculature, which are closely related to their chemical structures. In particular, the prominent antiarrhythmic effects and low toxicity of hetisine-type DAs highlight their potential in antiarrhythmic drug discovery. Hetisine-type DAs with diverse bioactivities are promising lead structures for further development as commercial agents in medicine.
1. Introduction
Diterpenoid alkaloids (DAs) are a large group of highly specialized metabolites that are distributed mainly in plants from the genera Aconitum, Delphinium, and Consolida in the Ranunculaceae family, as well as the genus Spiraea in the Rosaceae family.1–3 For centuries, the complex structures and multiple prominent pharmacological activities of DAs have attracted extensive and lasting attention from scientists.4–6 DAs are the active components of several widely used traditional Chinese medicines (TCMs) from Ranunculaceae, such as Fuzi (Aconitum carmichaelii), which has been used medicinally for thousands of years in China and other Asian countries7–9. Modern pharmacological studies have demonstrated a wide range of remarkable pharmacological activities for DAs, especially prominent anti-inflammatory, analgesic, and antiarrhythmic effects, emphasizing their great potential for drug discovery.10,11 Currently, a series of DAs have been developed as new drugs and are used extensively in the clinic, for example the analgesic agents 3-acetylaconitine, lappaconitine, and crassicauline A.12
DAs are also well known for their complex chemical structures, which feature a polycyclic fused cage-like skeleton along with various kinds of substituents. Structurally, DAs are usually classified into four types according to the number of carbons on their skeleton: C18–, C19–, C20– or bis-type, which can be further divided into several dozen subtypes. Among them, C20-DAs are famed for their abundant structurally diverse skeletons: nearly thirty C20-DA subtypes have already been reported, and large numbers of C20-DAs with new skeletons continue to emerge.13,14 Hetisine-type DAs are one of the most important C20-DA subtypes. Since the time when hetisine-type was defined in 1960s,15 numerous compounds have been isolated and identified, making this type the most abundant subtype of C20-DAs in terms of quantity. In addition, preliminary studies have revealed that hetisine-type DAs have a wide range of pharmacological actions, including antiarrhythmic,16,17 antitumor,18,19 antimicrobial and insecticidal activities,20,21 as well as effects on peripheral vasculature.22 In particular, hetisine-type DAs possess more prominent antiarrhythmic effects than the other DAs along with low toxicity, which highlights their potential in antiarrhythmic drug discovery. Fortunately, a representative compound of hetisine-type DAs, Guan-fu base A (49), has been discovered and introduced as a new antiarrhythmic drug (acehytisine hydrochloride injection) by Chinese scientists.12 These findings underscore the still largely untapped potential of hetisine-type DAs and encourage their further extensive investigation.
Several previously published review articles and monographs have involved the hetisine-type DAs.1,14,23 However, these literatures mainly focus on the research advances of all types of DAs or C20-DAs, and only a small portion of contents has been devoted to the hetisine-type DAs. Deserving more attention are the works by Wang and Liang,13 in which there are plentiful descriptions on structures and bioactivities of hetisine-type DAs with literatures coverage to the end of 2000. Besides, Bessonova and Saidkhodzhaeva listed 107 hetisine-type C20-DAs that had been isolated up to 1998.104 During the past two decades, a number of new hetisine-type DAs have been discovered, and their bioactivities were also studied. Hence, this review was prepared to summarize the structural features and biological activities of natural hetisine-type DAs, intending to provide a complete overview of the existing knowledge of the chemical structures and biological properties of hetisine-type DAs, which will facilitate further research and exploitation of these types of compounds.
2. Structures and classifications
Although the first hetisine-type DA, paniculatine (56), has been isolated in the early 1920s,24 and several representative compounds, such as hetisine (13) and kobusine (9), were found during the 1940s,25,26 the structures of hetisine-type DAs were established much later due to their complex fused polycyclic skeleton. The chemical skeleton of hetisine-type DAs was first proposed in 1963 and finally established by single crystal X-ray diffraction studies of several representative compounds.15 Hetisine-type DAs possess a heptacyclic hetisane-type C20-diterpenoid framework (Fig. 1), whereas hetisane-type diterpenoid itself has never been found from plants. It is generally assumed that atisine-type DAs, which formed by amination of serine at atisane-type diterpenoid skeleton, are the biosynthetic precursors of hetisine-type DAs.13 Atisine-type DAs might undergo Mannich reaction to generate hetidine-type DAs, and a subsequent linkage of N–C(6) bond led to the formation of hetisine-type DAs.27 Besides, the cleavage of the N–C(19) bond of hetisine-type DAs could generate vakognavine-type DAs. Although vakognavine-type has been regarded as a subtype of hetisine-type DAs in some situations in the past mainly due to their small quantity, this review exclusively examined vakognavine-type DAs considering the discovery of numerus vakognavine-type DAs over the past decades.9,14,28 Additionally, several hetisine-derived compounds with new skeletons are not included in this review, for example anthriscifolsine A from Delphinium anthriscifolium var. majus with a cleaved N–C(20) bond,29 and grandiflodine A from D. grandiflorum bearing a seco C ring.30
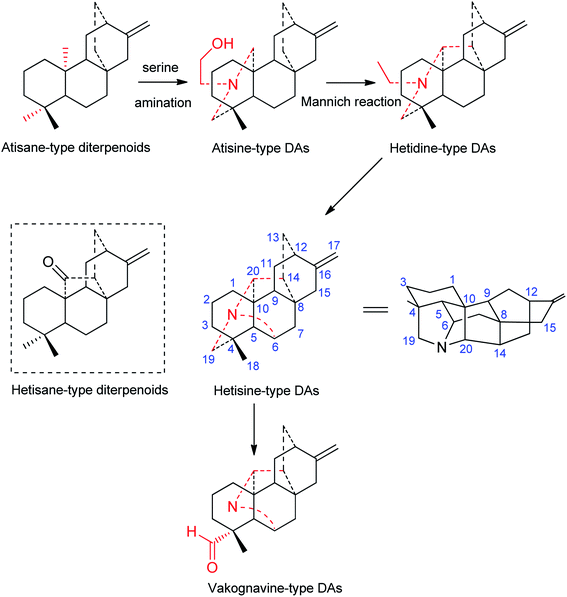 |
| Fig. 1 The skeletons of hetisine-type and its closely related types of C20-DAs. | |
According to single crystal X-ray diffraction analysis of corresponding hetisine-type DAs for example hetisine (13),15 the six-membered cyclohexane rings A (C(1), C(2), C(3), C(4), C(5), C(10)) and B (C(5), C(6), C(7), C(8), C(9), C(10)) found in hetisine-type DAs have the chair conformation (projection formula in Fig. 1), while piperidine ring F (C(4), C(5), C(10), C(20), N, C(19)) exists in a boat conformation. Rings C (C(8), C(9), C(11), C(12), C(13), C(14)), D (C(8), C(9), C(11), C(12), C(16), C(15)) and E (C(8), C(14), C(13), C(12), C(16), C(15)) form the bicyclo-[2,2,2]-octane system, which is fixed in the boat form. The five-membered G ring (C(4), C(5), C(6), N, C(19)) adopts the twist form, while ring H (C(5), C(6), N, C(20), C(10)) is in the envelope form; and ring I (C(8), C(9), C(10), C(20), C(14)) is found in an equal mixture of the twist and envelope conformations. The fusion of rings A, B, and C is identical for all hetisine-type DAs: A/B, trans; B/C, cis.
Based on the state of the N atom, hetisine-type DAs can be divided into four subtypes, namely, the amine subtype (I), the N,O-mixed acetal subtype (II), the N-oxide derivatives (III), and the quaternary ammonium bases (IV). Table S1† listed the names, subtypes, plant sources, and the references of a total of 157 natural-occurring hetisine-type DAs reported in the past decades. Herein, the structural features of hetisine-type DAs are discussed by category.
2.1. The amine subtype compounds
The amine subtype with 94 members constitutes the majority (60%) of the hetisine-type C20-DAs (Fig. 2). The chemical diversity of these amines (and other subtypes of hetisines) depends mainly on the variety, quantity, position, and orientation of the oxygenated substituents. The common oxygenated substituents found in hetisine-type DAs are hydroxyl (OH), ketone carbonyl (
O), and ester groups such as acetyl (Ac), isobutyl (iBu), 2-methylbutyryl (MeBu), and benzoyl (Bz) groups. Rarely, palmasine (20) and palmadine (21) from A. palmatum feature an uncommon cinnamoyl (Cn) group at C-13,31 and acoridine (48), Guan-fu bases R (39) and O (50) from A. coreanum possess a propionyl (Pr) group at C-2.32–34 Most hetisine-type DAs have an exocyclic terminal olefinic bond at C-16 and C-17. However, four hetisines, including Guan-fu bases S (91), N (92),35 and W (93)36 from A. coreanum and zeraconine (94) from A. zeravschanicum,37 possess a cyclic olefinic bond at C-15 and C-16. Guan-fu base S (91) has an extra double bond at C-2 and C-3,33,38 while zeraconine (94) features a rare hordenine substituted at C-17.37
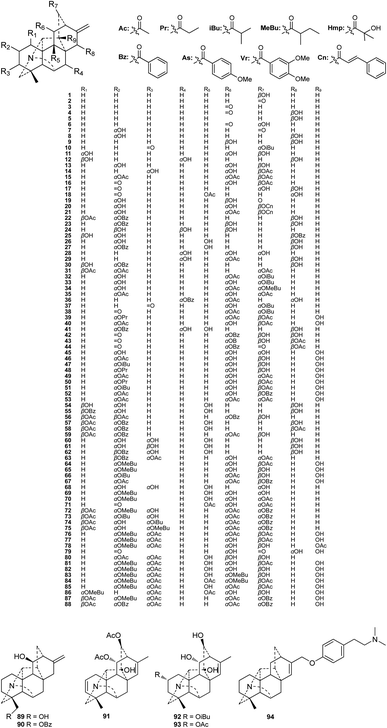 |
| Fig. 2 The amine subtype alkaloids (1–94) of hetisine (the names of the alkaloids are given in ESI Table S1†). | |
Only three monosubstituted amines have been reported, namely, spirasines XI (1), IV (2), and IX (3), from Spiraea japonica var. fortunei.39 The other amine subtype compounds possess between two and six oxygenated substituents at different positions in the molecular skeleton. The most likely substituted positions are C-2, C-6 and C-7, with probabilities greater than 50%. The other positions, including C-1, C-3, C-5, C-6, and C-15, are also easy to substitute. The reported amine subtype hetisines mainly contain oxygenated substituents at the abovementioned positions, with the exception of davisinol (89) and 18-benzoyldavisinol (90), which have an oxygenated substituent at C-18, a rare substituted position.40 The orientation of these oxygenated substituents may vary when they are substituted on a methylene, for example on C-1, C-2, C-3, C-11, C-13, C-15, or C-19. However, these oxygenated substituents at rigid bridged-ring methines such as C-6, C-9, and C-14 fix the β-orientation for all of hetisine-type DAs.
2.2. The N,O-mixed acetal subtype compounds
A total of 55 alkaloids belonging to the N,O-mixed acetal subtype (II) have been reported (Fig. 3). Among them, delatisine (95) from D. elatum possesses an N–C(19)–O–C(2) mixed acetal unit,41 and orgetine (96) from A. recemulosum42 features an N–C(20)–OH mixed acetal group. Generally, methoxy groups are rarely found in C20-DAs. However, racemulosine A (97)43 and flexiosine (146)44 have methoxy groups at C-6 and C-19, respectively, forming a special kind of N,O-mixed acetal unit. The other N,O-mixed acetal subtype compounds can be classified into two groups, namely, alkaloids with N–C(6)–OH units (98–130), and the remaining alkaloids (131–149) that contain an N–C(19)–OH mixed acetal group. Among them, several possess uncommon substituent groups, for example, 15-veratroylpseudokobusine (113) from A. yesoense var. macroyesoense has a veratroyl group at C-15,45 and tanguticuline D (141) A. tanguticum from features a sulfonate moiety (SO3H) at C-11.46 Besides, anthriscifolmine J (145) from D. anthriscifolium var. savatieri has an uncommon 2-hydroxy-2-methylpropionyl (Hmp) group at C-3.47
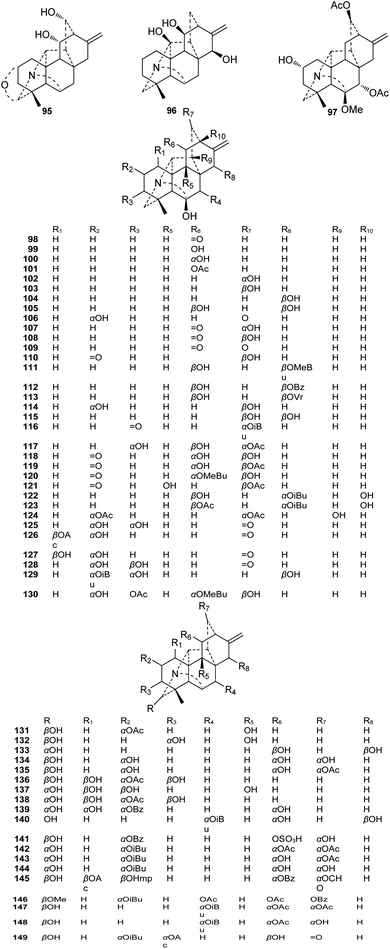 |
| Fig. 3 The N,O-mixed acetal subtype alkaloids (95–149) of hetisine. | |
2.3. The N-oxide derivatives
Currently, only four N-oxygenated hetisine-type DAs, namely, 14-hydroxyhetisinone N-oxide (150) from D. gracile,48 Guan-fu bases Z and F N-oxide (151 and 152),49–51 and Guan-fu base X (153) from A. coreanum,35,36 have been reported (Fig. 4). In addition, compound 154 is a derivative of zeraconine (94),37 an N-oxide at the nitrogen atom of the dimethylamino group.
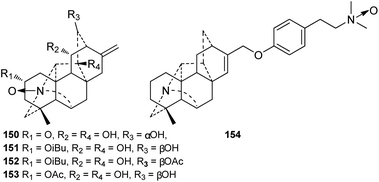 |
| Fig. 4 The N-oxide derivatives (150–154) of hetisine. | |
2.4. The quaternary ammonium bases
A series of alkaloids were reported as quaternary ammonia bases or salt forms of hetisine-type DAs in the past decades. It has been well documented that hetidine-type DAs with a ketone at C-6 and vakognavine-type alkaloids (bearing an aldehyde group at C-19) can transform into their salt form bearing an N,O-acetal in the presence of acids and can return to their free alkaloid form after treatment with bases.52 Since DAs in plants are mainly extracted by using acids, primarily dilute hydrochloric acid, these quaternary ammonia bases or hetisine salts might be artifact products of the corresponding hetidine-type or vakognavine-type DAs.53 For example, chemical transformation experiments have suggested that orochrine and 2-O-acetylorochrine are artifacts of the corresponding hetidine-type DAs heterophylloidine and deacetylheterophylloidine, respectively,54,55 and carmichaelines B–D are indeed the trifluoroacetates of corresponding vakognavine-type C20-DAs.56–58 Particularly, three hetisine-type DAs with 7,8-diol unit (Fig. 5), namely, vilmorrianines F and G (155 and 156) from A. vilmorrianum54 and 2-O-acetyl-7R-hydroxyorochrine (157) from A. orochryseum,55 cannot be transformed into their free alkaloid form possibly due to the intramolecular hydrogen bond between OH-7 and OH-8, indicating that they might be naturally-occurring quaternary ammonia bases.
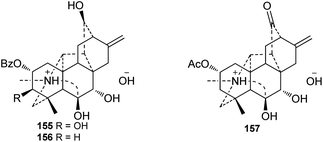 |
| Fig. 5 The quaternary ammonium bases (155–157) of hetisine. | |
3. Distribution
Currently, hetisine-type DAs have been found in plants from seven genera in three families. As shown in Table 1, the richest sources are plants from the genera Aconitum and Delphinium in the family Ranunculaceae, as approximately 55% and 39% of the reported hetisine-type DAs have been found in plants of the genera Aconitum and Delphinium, respectively. In addition, fifteen hetisine-type DAs (9%) have been proven to exist in the genus Consolida (Ranunculaceae),59–62 a genus closely related to Delphinium. Only one hetisine-type DA was identified in the genus Thalictrum (Ranunculaceae), namely, spiradine A (98) from Thalictrum sessile.63 In general, the family Ranunculaceae can be regarded as the main source of hetisine-type DAs.
Table 1 The distributions of hetisine-type DAsa
Family |
Genus |
Number of hetisine-type DAs |
The numbers of hetisine-type DAs were counted independently in different genera in the case that a particular alkaloid exists in plants in different genera. |
Ranunculaceae |
Aconitum |
86 |
Delphinium |
62 |
Consolida |
15 |
Thalictrum |
1 |
Rosaceae |
Spiraea |
11 |
Polygonaceae |
Rumex |
3 |
Polygonum |
1 |
Apart from plants of the Ranunculaceae family, the genus Spiraea in the family Rosaceae is another important source of hetisines. Eleven hetisine-type DAs (7%) have been obtained from the genus Spiraea, and they are distributed exclusively in the species S. japonica64–68 and its variety S. japonica var. fortunei.39,69–73 It is also noteworthy that hetisine-type DAs from Spiraea plants possess relatively few and simple oxygenated substituents: they usually possess only one or two hydroxyl or ketone carbonyl substituents at C-6, C-11, and C-13, which is of chemotaxonomic significance.3 In addition, hetisine-type DAs have been found in plants of the genera Rumex and Polygonum in the family Polygonaceae, including orientinine (79), panicudine (106), and acorientine (115) from Rumex pictus74 and panicudine (106) from Polygonum aviculare.20 Hetisine-type DA is the only type of DA that has been found in the polygonaceae family, which widens the distribution of DAs.
In terms of the distribution of certain compounds, hetisine (13) is the most widely distributed compound, which has been reported to be isolated from 26 species of plants and 3 varieties in three genera (Table 1S†), followed by hetisinone (16) distributed in 19 species and 2 varieties in three genera. The wide distribution of hetisine (13) and hetisinone (16) could support the hypothesis that hetisine (13) is the biosynthetic precursor of other hetisine-type compounds.
4. Bioactivities
4.1. Antiarrhythmic activity
Since 1981, when Liu et al. first discovered the hetisine-type DA Guan-fu base A (49) from A. coreanum bearing considerable antiarrhythmic activity, the antiarrhythmic activity of guan-fu base A and its analogs has attracted much interest from pharmaceutical scientists.75 Through a 20 year persistent effort by Chinese researchers, mainly Liu and coworkers, Guan-fu base A has been developed into a new antiarrhythmic drug (acehytisine hydrochloride injection) after passing through phase II and III clinical studies to treat paroxysmal supraventricular tachycardia, and this compound was approved by the China Food and Drug Administration (CFDA) in 2005.12,75
It was observed in experiments that Guan-fu base A can effectively antagonize aconitine-induced arrhythmia in rats, significantly reduce the CaCl2-induced incidence of ventricular fibrillation in rats,75 markedly raise the ventricular fibrillation threshold to electrical stimulation in rabbits and cats,75 and increase the ouabain dose necessary to cause ventricular premature beats, ventricular fibrillation, and cardiac arrest in anesthetized guinea pigs.75 The antiarrhythmic effects of Guan-fu base A might be due to its electrophysiological properties, which has been verified as a multi-ion channel blocker (sodium channel,76,77 calcium channel,77 potassium channel,78,79 and hyperpolarization-activated cyclic nucleotide-gated (HCN) channel80). In clinical studies, Guan-fu base A (49) showed unambiguous therapeutic efficacy on paroxysmal supraventricular tachycardia (PSVT), paroxysmal atrioventricular reentrant tachycardia, and ventricular tachycardia,81 along with reliable safety in humans.81,82 In addition, it was found that Guan-fu base A did not affect the function of the atrionector when it exerted other electrophysiological effects, which is beneficial to its application.83
Guan-fu bases G (53) and I (Guan-fu base Y or acorine, 46) in the same plant also exhibited antiarrhythmic activity in animal models with the decreasing order of 53 > 49 > 46,16 which are consistent with their acylation degrees. Besides, four hetisine-type DAs (4, 13, 52 and 59) from A. zeravschanicum exhibited antiarrhythmic activity in aconitine-introduced arrhythmia rat model greater than heteratisine, a C19-DA with pronounced antiarrhythmic activity (ED50, 12.5 mg kg−1).17 It is worth noting that the diester alkaloids tadzhaconine (59, ED50 = 0.3 mg kg−1) and zeravshanisine (52, ED50 = 0.5 mg kg−1) showed much better effects than the alcohol amines hetisine (13, ED50 = 1 mg kg−1) and nominine (4, ED50 = 5 mg kg−1). Moreover, it was found that the all acetylated derivatives of Guan-fu alcoholamine (tangutisine, 45) by different ester groups such as Ac, Pro and Bz could effectively enhance its antiarrhythmic activity.84 The above findings indicated that the antiarrhythmic activity of Guan-fu base A analogues is closely related to the number and variety of acyl groups on certain positions, and enhanced antiarrhythmic activity can be achieved by further chemical modifications. Another structure–activity relationship (SAR) study revealed that the propylene glycol amine group might be the key pharmacophore responsible for the antiarrhythmic activity of guan-fu base A analogues (Fig. 6).85
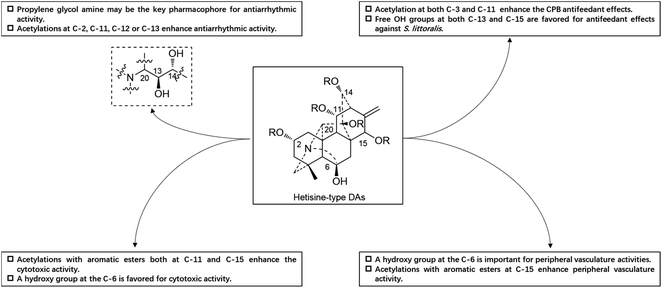 |
| Fig. 6 The SAR of hetisine-type DAs. | |
Since the sodium current plays a crucial role in the early depolarization and duration of the cardiac action potential and is involved in the propagation of electrical impulses from one cell to another, sodium current blockers could be a useful candidate target for new potential therapeutic agents against arrhythmia. The sodium current inhibitory effects of 21 compounds isolated from A. coreanum were tested using a whole-cell patch voltage-clamp technique, and five hetisine-type DAs exerted significant blocking activities.35 Among them, Guan-fu base S (91, IC50 = 3.48 μM) showed a strong inhibitory effect on sodium current, which could suppress the sodium current of guinea pig ventricular myocytes in a dose-dependent manner by binding to open channels and exhibited potential for further development as an antiarrhythmic agent. Guan-fu base Q (126, IC50 = 82.65 μM), hetisine (13, IC50 = 75.72 μM), Guan-fu bases A (49, IC50 = 41.17 μM) and G (53, IC50 = 23.81 μM) showed moderate antiarrhythmic activities and were more effective than the positive control acehytisine hydrochloride (crude drug of Guan-fu base A injection, IC50 = 78.26 μM). Besides, it was reported that hetisinone (16) showed moderate inhibitory activity on the Nav 1.2 channel (inhibition rate 28% at 10 μM) in another study.86
4.2. Antitumor activities
A certain number of naturally occurring hetisine-type DAs have been screened for their cytotoxic activities against various human cancer cell lines.87 However, only a few have exhibited significant antiproliferative activity.88 Trichodelphinines A–E (32–35, 38) from D. trichophorum were evaluated for their in vitro cytotoxicity against the A549 cell line using the MTT method.18 All of the above-mentioned compounds showed cytotoxicity against A549 cells with IC50 values ranging from 12.0 to 52.8 μM. The most active compounds, 33 and 38, had low IC50 values of 18.64 and 12.03 μM, respectively, while the positive control doxorubicin showed an IC50 value of 0.60 μM. In addition, nagaconitine D (124) from A. nagarum var. heterotrichum showed cytotoxicity against the human ovarian cancer cell line SK-OV-3 with an inhibition rate of 24% (IC50 = 32.1 μM) compared to the reference drug cisplatin (89%) with an IC50 value of 11.6 μM.89
While natural hetisine-type DAs themselves are rarely active against certain human cancer cell lines, increasing cytotoxic activity has been observed after the corresponding hetisine-type DAs undergo acetylation.88,90 Wada et al. synthesized a series of acylated derivatives of the natural hetisine-type DAs kobusine (9) and pseudokobusine (105), the main constituents of A. japonicum, and evaluated their cytotoxic activities against three human cancer cell lines: lung (A549), prostate (DU145), and nasopharyngeal (KB).91–93 Some of these hetisine derivatives displayed impressive cytotoxicity against all of the tested cancer cells, with IC50 values ranging from 3.1 to 20.1 μM (Table 2). More significantly, all of active compounds displayed potency against vincristine-resistant nasopharyngeal (KB-VIN) cell line with ratios of KB to KB-VIN greater than 0.7, in contrast with the positive control paclitaxel (KB/KB-VIN ratio, 0.0067). Structurally, the most active kobusine derivatives were those with both C-11 and C-15 acylated, and the pseudokobusine derivatives containing a free hydroxy group at C-6 were more active than their acylated products. The results indicated that an acyl group replacement at both C-11 and C-15 resulted in the enhancement of activity of the parent alkaloids compared to hydroxy groups at these positions, and the presence of a hydroxy group at the C-6 position was required for cytotoxic effects (Fig. 6).
Table 2 Cytotoxicity of kobusine (9) and pseudokobusine (105) and their acylated derivatives (IC50, μM)
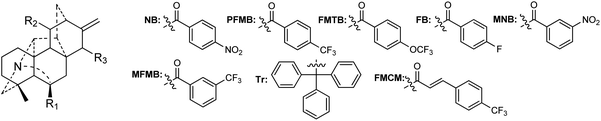
|
R1–R3 |
A549 |
DU145 |
KB |
KB-VIN |
KB/KB-VIN ratio |
R2 = R3 = OH (9) |
>40 |
>40 |
>40 |
>40 |
— |
R2 = R3 = OBz (9a) |
8.4 |
9.3 |
6.0 |
7.5 |
0.80 |
R2 = R3 = OAs (9b) |
6.7 |
7.1 |
5.3 |
5.2 |
1.02 |
R2 = ONB, R3 = OH (9c) |
19.5 |
15.3 |
13.9 |
17.9 |
0.78 |
R2 = R3 = ONB (9d) |
6.9 |
7.0 |
5.3 |
5.5 |
0.96 |
R2 = OPFMB, R3 = OH (9e) |
17.2 |
13.2 |
12.7 |
14.1 |
0.90 |
R2 = OFMTB, R3 = OH (9f) |
14.1 |
9.6 |
11.7 |
10.9 |
1.07 |
R2 = R3 = OFB (9g) |
8.1 |
6.8 |
5.2 |
7.1 |
0.73 |
R2 = R3 = OFMCM (9h) |
5.5 |
6.2 |
4.1 |
3.1 |
1.32 |
R1 = R2 = R3 = OH (105) |
>40 |
>40 |
>40 |
>40 |
— |
R1 = R2 = OBz, R3 = OH (105a) |
19.3 |
15.3 |
12.8 |
10.2 |
1.25 |
R1 = OH, R2 = R3 = OBz (105b) |
8.8 |
7.6 |
5.2 |
6.3 |
0.83 |
R1 = R3 = OH, R2 = OAs (105c) |
15.4 |
13.2 |
11.1 |
15.7 |
0.70 |
R1 = R3 = OH, R2 = OVr (105d) |
8.0 |
15.3 |
14.9 |
20.1 |
0.74 |
R1 = R2 = OVr, R3 = OH (105e) |
16.0 |
16.9 |
19.7 |
14.7 |
1.34 |
R1 = R3 = OVr, R2 = OH (105f) |
15.2 |
16.6 |
18.1 |
12.2 |
1.48 |
R1 = R2 = OH, R3 = ONB (105g) |
5.8 |
7.2 |
6.4 |
6.4 |
1.00 |
R1 = OH, R2 = R3 = OMNB (105h) |
5.0 |
5.2 |
5.6 |
5.6 |
1.00 |
R1 = R3 = OH, R2 = OMFMB (105i) |
6.8 |
7.7 |
8.9 |
6.2 |
1.44 |
R1 = R3 = OH, R2 = OFMTB (105j) |
17.9 |
14.5 |
15.7 |
13.9 |
1.13 |
R1 = R3 = OH, R2 = OCn (105k) |
8.4 |
6.5 |
7.0 |
6.4 |
1.09 |
R1 = R3 = OH, R2 = OTr (105l) |
6.4 |
6.0 |
6.6 |
5.2 |
1.27 |
Paclitaxel (nM) |
4.8 |
5.9 |
5.8 |
2405.4 |
0.0067 |
Some of these active pseudokobusine derivatives have been further studied for their pharmacological mechanism.19,94 It was found that 11-m-trifluoromethylbenzoyl (Mb)-pseudokobusine clearly inhibited the phosphorylation of extracellular signal-regulated kinase, induced enhanced phosphoinositide 3 kinase phosphorylation and led to the subsequent accumulation of cells in the G1 and/or sub G1 phase in Raji cells.19 In addition, no significant suppressive effects on the growth of human CD34+ hematopoietic stem/progenitor cells (HSPCs) were observed with this compound, whereas its analogue 11-As-pseudokobusine showed a significant suppressive effect on the growth of HSPCs. These findings underscore the potential of hetisine-type DAs to be developed into new, specific antitumor compounds with an optimal balance between antitumor effects and myelosuppression and encourage further extensive investigations of the antitumor effects of hetisine-type alkaloids.
4.3. Effect on peripheral vasculature
A series of hetisine-type DAs, namely, kobusine (9) and pseudokobusine (105), from A. yesoense var. macroyesoense and their derivatives have been reported to exhibit peripheral vasculature activities by laser-flowmetrically measuring the cutaneous blood flow in the hind feet of mice after intravenous administration. An earlier study in 1997 by Wada et al. reported that both kobusine (9) and pseudokobusine (105) exhibited a rapid increase in blood flow, reaching a peak with a magnitude almost equal to that produced by hydralazine when administered intravenously at the same dose of 20 mg kg−1, while other tested compounds showed only mild to moderate effects.22 This effect on blood flow is probably due to the direct action on the cutaneous microvasculature that occurs in a similar fashion to that shown by hydralazine. A subsequent study showed that only the C-11 and C-15 esterified derivatives of kobusine and pseudokobusine showed significant effect on vasculature peripheral vasculature,95 which inspired the authors to synthesize a series of esterified derivatives to search for more effective analogs.96 Conclusively, these studies have led to the discovery of a certain number of hetisines with prominent effects on cutaneous blood flow in mice.97 SAR analysis revealed that hydroxyl groups of these alkaloids, especially a free OH group at C-6, are important for action on the peripheral vasculature leading to dilatation, and that the alkaloids with a 15-aromatic ester groups, e.g., As, Vr, or NB (nitrobenzoyl), may have enhanced activity compared with the parent alkaloids.
4.4. Insecticidal activity
Traditionally, plants from the genera Aconitum, Delphinium and Consolida have been widely used as natural insecticides against various kinds of agricultural pests, which implies that their major constituents, namely, DAs, possess insecticidal activity.98,99 Hence, DA components from these plants, including a certain number of hetisine-type DAs, have been screened for their insecticidal activity.
Ulubelen et al. tested the insect repellent activities of 29 natural DA components, including six hetisines (13, 16, 17, 31, 90 and 100) isolated from Turkish Delphinium and Consolida species, against a common household pest, the red flour beetle (Tribolium castaneum Herbst.).21 It was found that hetisine (13), with a repellency of 59.12% at 3 mg mL−1, possessed the highest activity among all tested alkaloids. Alkaloids 17, 31, and 90 also showed a repellency class III effect (40.1–60%) with repellency values of 56.25%, 40.62%, and 45.87% at 3 mg mL−1, respectively, while 16 and hetisinone (16) showed only a low-class II repellent effect, with a repellency value of 31.25% and 37.50% at 3 mg mL−1, respectively.
Seven natural hetisine-type DAs (16, 42, 43, 84, 87–88, and 150), along with three hydrolysis products, 13-oxo-cardiopetamine (42a), 13-acetyl-15-oxo-cardiopetamine (42b), 15β-hydroxy-hetisinone (42c), were screened for their insect antifeedant activity against the Colorado potato beetle (CPB, Leptinotarsa decemlineata) and Spodoptera littoralis, as well as their toxicity to insect-derived Sf9 cells (derived from Spodoptera frugiperda pupal ovarian tissue) and mammalian Chinese hamster ovary (CHO) cells (Table 3).100,101 Among the tested compounds, cardiodine (87), with an EC50 value of 2.2 μg cm−2, was found to be the most active CPB antifeedant, followed by glandulosine (84), with an EC50 value of 4.0 μg cm−2, which implied that acetylation at both C-3 and C-11 might enhance their CPB antifeedant effects. Hetisinone (16) and cardiopetamine (42) also showed certain activity against L. decemlineata, with EC50 values of 13.1 and 22.5 μg cm−2, respectively. The most active antifeedants against S. littoralis were cardiodine (87, EC50, 4.4 μg cm−2) and cardiopetamine (42, EC50, 5.5 μg cm−2). In addition, 15β-hydroxy-hetisinone (42c) showed an EC50 value of 23.7 μg cm−2, indicating that OH groups at both C-13 and C-15 are favored for antifeedant effects against S. littoralis. In addition, only 42a with a ketone group at C-13 showed cytotoxicity to Sf9 and CHO cells with LD50 values of 5.3 and 12.5 mg mL−1, respectively, indicating that its insecticidal effects could be the result of neurotoxicity and/or cytotoxicity. It was observed that the of hetisine-type DAs. In general, although hetisine-type C20-DAs show relatively lower antifeedant activities than C19-DAs,102 they still have a certain advantage in serving as lead compounds in insecticide development because of their low toxicity to humans.
Table 3 Antifeedant effects of hetisine-type DAs on L. decemlineata and S. littoralis (EC50, μg cm−2) and cytotoxicity on Sf9 and CHO cells (LD50, mg mL−1)
Alkaloids |
L. decemlineata |
S. littoralis |
Sf9 cells |
CHO |
16 |
13.1 |
>50 |
>100 |
>100 |
42 |
22.5 |
5.5 |
— |
— |
43 |
12.9 |
>100 |
— |
— |
42a |
— |
>100 |
5.3 |
12.5 |
42b |
27.2 |
>100 |
>100 |
>100 |
42c |
100 |
23.7 |
>100 |
>100 |
87 |
2.2 |
4.4 |
>100 |
>100 |
84 |
4.0 |
>50 |
>100 |
>100 |
88 |
>50 |
>50 |
— |
— |
151 |
>50 |
>50 |
— |
— |
4.5. Antimicrobial and antiviral activities
Plants with abundant DA components have been used for the treatment of itch and other skin eruptions in folk medicine, which indicates that these plants may possess constituents with antifungal activity.98 The bioactivity-guided separation of the chloroform fraction with antimicrobial activities from P. aviculare led to the isolation of the hetisine-type DA panicudine (106), which was evaluated for its antimicrobial activity against both Gram-negative bacteria (Escherichia coli, Proteus mirabilis, Pseudomonas aeruginosa, Salmonella typhi, Salmonella paratyphi and Shigella flexneri), Gram-positive bacteria (Staphylococcus aureus, Bacillus subtilis, and Streptococcus pyogenes) and fungi by determining the diameter of the inhibition zone using the paper disk diffusion method.20 Consequently, panicudine (106) displayed considerable activity against all tested Gram-negative and Gram-positive organisms (diameter of zone of inhibition ranging from 5–23 mm at 0.4–400 mg mL−1), with the exception of Candida albicans. Hetisine-type DAs have also shown certain antiviral activity. Tanguticulines A (68) and E (125) from A. tanguticum exhibited obvious inhibitory effects on the cytopathic changes induced by the influenza A virus (H1N1), with IC50 values of 2.9 μg mL−1.46
4.6. Toxicity
To date, only a few hetisine-type DAs have been assessed for their acute toxicity in mice. The LD50 values (ip) for alkaloids 49, 59, 52, 13, and 4 were 185.5 mg kg−1, 12.8 mg kg−1, 34.1 mg kg−1, 26.2 mg kg−1, and 68.0 mg kg−1, respectively.17,103 From the data available, the toxicity of hetisine-type DAs is much lower than that of diester-type C19-DAs, such as aconitine (ip, LD50 = 0.30–0.38 mg kg−1), hapaconitine, or yunaconitine, which is beneficial for the applications of hetisine-type DAs in the pharmaceutical industry.
5. Conclusions
To date, a total of 157 hetisine-type DAs have been discovered, which are the most abundant subtype of C20-DAs. The reported hetisine-type DAs have been found in plants from seven genera in three families. The genera Aconitum and Delphinium of Ranunculaceae could be regarded as the richest resources of hetisine-type DAs. In terms of subtypes, the amine subtype with 94 members is the most common hetisine-type compounds, as they account for the largest proportion of reported compounds (60%), followed by the N,O-mixed acetal subtype with 56 members (35%). The other subtypes account for only a small portion of compounds (5%). The variety, quantity, position, and orientation of the oxygenated substituents make the large chemical diversity of hetisine-type DAs, which could serve as a vast resource for drug discovery.
Natural hetisine-type DAs have been reported to have various biological activities, including antiarrhythmic, antitumor, insecticidal, antifungal, and antiviral activities, as well as effect on peripheral vasculature. Besides, SAR studies revealed that more potent biological activities could be acquired after chemical modifications at certain positions. These findings are not only conducive to illuminating the pharmacodynamic material basis of plants with abundant hetisine-type DA components but also indicate their potential in drug discovery. In particular, the antiarrhythmic effects of hetisine-type DAs are more prominent than that of other DAs, and their lower toxicity highlights the potential of hetisine-type DAs in antiarrhythmic drug discovery.
Although chemical and biological studies on hetisine-type DAs have attracted considerable interest, some deficiencies and research potential remain. First, some of the studies ignored the probable structural transformations of several types of C20-DAs during the extraction processes. Thus, some of the reported hetisine-type quaternary ammonium hydroxides or salts are indeed artifacts of hetidine-type or vakognavine-type DAs. More detailed chemical studies on the structural identification of these alkaloids are required. Second, most of the biological activities of hetisine-type DAs have been investigated by using in vitro chemical and cellular models, and little clinical or in vivo research is currently available. These pharmacological studies are insufficient to validate the effects of hetisine-type DAs and their derived compounds, which hinders their application and promotion. It is necessary to evaluate the biological activities of the constituents using both in vitro and in vivo pharmacological models to facilitate further research and exploitation of these types of compounds.
Author contributions
Tianpeng Yin – resources, supervision, visualization, writing – original draft, writing – review and editing. Huixia Zhang –writing – review and editing. Wei Zhang – supervision, writing – review and editing, funding acquisition. Zhihong Zhang – supervision, writing – review and editing, funding acquisition.
Conflicts of interest
The authors declare no conflict of interest.
Acknowledgements
This research was funded by the Science and Technology Development Fund, Macau SAR (file no. 0077/2019/A2 and 0023/2019/AKP, and 0002/2019/APD).
References
- F. P. Wang, Q. H. Chen and X. Y. Liu, Nat. Prod. Rep., 2010, 27, 529–570 RSC.
- T. P. Yin, L. Cai and Z. T. Ding, RSC Adv., 2020, 10, 13669–13686 RSC.
- T. P. Yin, L. Cai and Z. T. Ding, RSC Adv., 2020, 10, 35072–35089 RSC.
- Y. Z. Wu, S. Shao, Q. L. Guo, C. B. Xu, H. Xia, T. T. Zhang and J. G. Shi, Org. Lett., 2019, 21, 6850–6854 CrossRef CAS PubMed.
- X. Zong, X. Yan, J. L. Wu, Z. Liu, H. Zhou, N. Li and L. Liu, J. Nat. Prod., 2019, 82, 980–989 CrossRef CAS PubMed.
- S. Huang, J. F. Zhang, L. Chen, F. Gao and X. L. Zhou, Phytochemistry, 2020, 178, 112459 CrossRef CAS PubMed.
- G. Zhou, L. Tang, X. Zhou, T. Wang, Z. Kou and Z. Wang, J. Ethnopharmacol., 2015, 160, 173–193 CrossRef CAS PubMed.
- T. P. Yin, H. Zhou, L. Cai and Z. T. Ding, RSC Adv., 2019, 9, 10184–10194 RSC.
- J. Yu, T. P. Yin, J. P. Wang, R. F. Mei, L. Cai and Z. T. Ding, Nat. Prod. Res., 2017, 31, 228–232 CrossRef CAS PubMed.
- E. Nyirimigabo, Y. Xu, Y. Li, Y. Wang, K. Agyemang and Y. Zhang, J. Pharm. Pharmacol., 2015, 67, 1–19 CrossRef CAS PubMed.
- F. P. Wang, Prog. Chem., 2009, 21, 63–65 CAS.
- C. P. Commission, Chinese Pharmacopoeia: Section I, China Medical Science and Technology Press, Beijing, 2020 Search PubMed.
- F. P. Wang and X. T. Liang, C20-diterpenoid alkaloids, 2002, pp. 1–280 Search PubMed.
- Y. Shen, W. J. Liang, Y. N. Shi, E. J. Kennelly and D. K. Zhao, Nat. Prod. Rep., 2020, 37, 763–796 RSC.
- M. Przybylska, Acta Crystallogr., 1963, 16, 871–876 CrossRef CAS.
- D. H. Hou, L. Y. Wu, W. R. Xu and J. H. Liu, J. China Pharm. Univ., 1987, 18, 268–272 CAS.
- B. T. Salimov, Z. K. Kuzibaeva and F. N. Dihakhangirov, Chem. Nat. Compd., 1996, 32, 366–368 CrossRef.
- C. Z. Lin, Z. X. Zhao, S. M. Xie, J. H. Mao, C. C. Zhu, X. H. Li, B. Zeren-dawa, K. Suolang-qimei, D. Zhu, T. Q. Xiong and A. Z. Wu, Phytochemistry, 2014, 97, 88–95 CrossRef CAS PubMed.
- K. Wada, M. Hazawa, K. Takahashi, T. Mori, N. Kawahara and I. Kashiwakura, J. Nat. Med., 2010, 65, 43–49 CrossRef PubMed.
- H. M. Salama and N. Marraiki, Saudi J. Biol. Sci., 2010, 17, 57–63 CrossRef CAS PubMed.
- A. Ulubelen, A. H. Mericli, F. Mericli, N. Kilincer, A. G. Ferizli, M. Emekci and S. W. Pelletier, Phytother Res., 2001, 15, 170–171 CrossRef CAS PubMed.
- K. Wada, S. Ishizuki, T. Mori, H. Bando, M. Murayama and N. Kawahara, Biol. Pharm. Bull., 1997, 20, 978–982 CrossRef CAS PubMed.
- M. S. Yunusov, Nat. Prod. Rep., 1991, 8, 499–526 RSC.
- G. E. Brunner, Schweiz. Apoth.-Ztg., 1922, 60, 357–358 CAS.
- W. A. Jacobs and L. C. Craig, J. Biol. Chem., 1942, 143, 605–609 CrossRef CAS.
- H. Suginome and H. Simamouti, Justus Liebigs Ann. Chem., 1940, 545, 220–228 CrossRef CAS.
- P. G. Xiao, F. P. Wang, F. Gao, L. P. Yan, D. L. Chen and Y. Liu, J. Soc. Evol. Syst., 2006, 44, 1–46 Search PubMed.
- T. P. Yin, Y. F. Yan, X. Li, G. W. Gong and W. J. Wang, Phytochem. Lett., 2021, 41, 142–146 CrossRef CAS.
- L. H. Shan, J. F. Zhang, F. Gao, S. Huang and X. L. Zhou, Sci. Rep., 2017, 7, 6063 CrossRef PubMed.
- N. H. Chen, Y. B. Zhang, W. Li, P. Li, L. F. Chen, Y. L. Li, G. Q. Li and G. C. Wang, RSC Adv., 2017, 7, 24129–24132 RSC.
- Q. P. Jiang and S. W. Pelletier, Tetrahedron Lett., 1988, 29, 1875–1878 CrossRef CAS.
- Y. Li and S. Liang, China J. Chin. Mater. Med., 2009, 34, 220–1222 Search PubMed.
- J. B. Xu, Y. Y. Luo, S. Huang, F. Gao and X. L. Zhou, Chem. Biodiversity, 2019, 17, e1900600 Search PubMed.
- I. A. Bessonova, L. N. Samusenko, M. S. Yunosov, M. R. Yagidaev and V. G. Kondratev, Chem. Nat. Compd., 1991, 27, 79–81 CrossRef.
- B. N. Xing, S. S. Jin, H. Wang, Q. F. Tang, J. H. Liu, R. Y. Li, J. Y. Liang, Y. Q. Tang and C. H. Yang, Fitoterapia, 2014, 94, 120–126 CrossRef CAS PubMed.
- Q. F. Tang, W. C. Ye, J. H. Liu and C. H. Yang, Phytochem. Lett., 2012, 5, 397–400 CrossRef CAS.
- Z. M. Vaisov and M. S. Yunusov, Chem. Nat. Compd., 1987, 23, 337–339 CrossRef.
- C. H. Yang, X. C. Wang, Q. F. Tang, W. Y. Liu and J. H. Liu, Helv. Chim. Acta, 2008, 91, 759–765 CrossRef CAS.
- F. Sun and D. Q. Yu, Acta Pharmacol. Sin., 1985, 20, 913–917 CAS.
- A. Ulubelen, H. K. Desai, S. K. Srivastava, B. P. Hart, J. C. Park, B. S. Joshi and R. Ilarslan, J. Nat. Prod., 1996, 59, 360–366 CrossRef CAS PubMed.
- S. A. Ross, B. S. Joshi, H. K. Desai, S. W. Pelletier, M. G. Newton, X. Zhang and J. K. Snyder, Tetrahedron, 1991, 47, 9585–9598 CrossRef CAS.
- L. V. Beshitaishvili and M. N. Sultankhodzhaev, Chem. Nat. Compd., 1992, 28, 206–208 CrossRef.
- Y. H. Ge, S. Z. Mu, S. Y. Yang, Y. Lu, J. X. Zhang, Y. Wang and X. J. Hao, Helv. Chim. Acta, 2009, 92, 1860–1865 CrossRef CAS.
- T. M. Gabbasov, E. M. Tsyrlina, D. M. Anatov, L. V. Spirikhin and M. S. Yunusov, Chem. Nat. Compd., 2017, 53, 105–108 CrossRef CAS.
- H. Bando, K. Wada, T. Amiya, K. Kobayashi, Y. Fujimoto and T. Sakurai, Heterocycles, 1987, 26, 2623–2637 CrossRef CAS.
- X. Fan, L. Yang, Z. Liu, L. Lin, C. Li, S. Guo, Z. Wang, Z. Wang and F. Sui, Phytochemistry, 2019, 160, 71–77 CrossRef CAS PubMed.
- X. Y. Liu, L. Song, Q. H. Chen and F. P. Wang, Nat. Prod. Commun., 2010, 5, 1005–1008 CAS.
- M. Reina, R. Mancha, A. Gonzalez-Coloma, M. Bailen, M. L. Rodriguez and R. A. Martinez-Diaz, Nat. Prod. Res., 2007, 21, 1048–1055 CrossRef CAS PubMed.
- F. N. Dzhakhangirov and I. A. Bessonova, Chem. Nat. Compd., 2002, 38, 74–77 CrossRef CAS.
- I. A. Bessonova, L. N. Samusenko, M. S. Yunusov and V. G. Kondrat'ev, Chem. Nat. Compd., 1990, 26, 318–320 CrossRef.
- I. M. Yusipova, B. Tashkhodzhaev, I. A. Bessonova, M. S. Yunusov, M. R. Yagudaev, V. G. Kondrat'ev and A. I. Shreter, Chem. Nat. Compd., 1990, 26, 314–318 CrossRef.
- X. T. Liang, Chin. J. Org. Chem., 1986, 5, 335–340 Search PubMed.
- Q. Jiang and S. W. Pelletier, J. Nat. Prod., 1991, 54, 525–531 CrossRef CAS.
- T. X. Tang, Q. F. Chen, X. Y. Liu, X. X. Jian and F. P. Wang, J. Asian Nat. Prod. Res., 2016, 18, 315–327 CrossRef CAS PubMed.
- P. Wangchuk, J. B. Bremner and S. Samosorn, J. Nat. Prod., 2007, 70, 1808–1811 CrossRef CAS PubMed.
- B. Jiang, S. Lin, C. Zhu, S. Wang, Y. Wang, M. Chen, J. Zhang, J. Hu, N. Chen, Y. Yang and J. Shi, J. Nat. Prod., 2012, 75, 1145–1159 CrossRef CAS PubMed.
- Z. T. Zhang, L. Wang, Q. F. Chen, Q. H. Chen, D. L. Chen, X. Y. Liu and F. P. Wang, Tetrahedron, 2013, 69, 5859–5866 CrossRef CAS.
- F. P. Wang, D. L. Chen, H. Y. Deng, Q. H. Chen, X. Y. Liu and X. X. Jian, Tetrahedron, 2014, 70, 2582–2590 CrossRef CAS.
- M. Grandez, A. Madinaveitia, J. A. Gavín, A. Alva and G. de la Fuente, J. Nat. Prod., 2002, 65, 513–516 CrossRef CAS PubMed.
- F. Mericli, A. H. Mericli, N. Tan, H. Oezcelik and A. Ulubelen, Sci. Pharm., 1999, 67, 313–318 CAS.
- S. Suzgec, L. Bitis, U. Sozer, H. Ozcelik, J. Zapp, A. K. Kiemer and A. H. Mericli, Chem. Nat. Compd., 2009, 45, 287–289 CrossRef CAS.
- F. Mericli, A. H. Mericli, H. K. Desai, A. Ulubelen and S. W. Pelletier, Sci. Pharm., 2001, 69, 63–67 CrossRef CAS PubMed.
- Y. C. Wu, T. S. Wu, M. Niwa, S. T. Lu and Y. Hirata, Phytochemistry, 1988, 27, 3949–3953 CrossRef CAS.
- F. Sun, X. T. Liang and D. Q. Yu, J. Nat. Prod., 1987, 50, 923–926 CrossRef CAS.
- Y. C. Wu, T. S. Wu, M. Niwa, S. T. Lu and Y. Hirata, Heterocycles, 1987, 26, 943–946 CrossRef CAS.
- B. G. Wang, X. Hong, G. Y. Zuo and X. J. Hao, J. Asian Nat. Prod. Res., 2000, 2, 271–281 CrossRef CAS PubMed.
- G. Goto, K. Sasaki, N. Sakabe and Y. Hirata, Tetrahedron Lett., 1968, 9, 1369–1373 CrossRef.
- F. Sun, X. T. Liang and D. Q. Yu, J. Nat. Prod., 1988, 51, 50–53 CrossRef CAS.
- L. M. Fan, H. P. He, Y. M. Shen and X. J. Hao, J. Integr. Plant Biol., 2005, 47, 120–123 CrossRef CAS.
- G. Goto, K. Sasaki, N. Sakabe and Y. Hirata, Tetrahedron Lett., 1968, 1369–1373 CrossRef CAS.
- L. M. Fan, H. P. He, Y. M. Shen and X. J. Hao, J. Integr. Plant Biol., 2005, 47, 120–123 CrossRef CAS.
- X. J. Hao, Prog. Chem., 2009, 21, 84–99 CAS.
- X. J. Hao, J. L. Nie, H. Sun and Z. J. Gu, Acta Bot. Yunnanica, 1997, 19, 297–303 CAS.
- H. M. H. Salama, Egypt. J. Bot., 1997, 37, 85–92 CAS.
- D. H. Hou, L. Li, Q. J. Wang and J. H. Liu, J. China Pharm. Univ., 1981, 17, 68–74 Search PubMed.
- X. Fan, C. Wang, N. Wang, X. Ou, H. Liu, Y. Yang, X. Dang, X. Zeng and L. Cai, J. Pharmacol. Sci., 2016, 132, 235–243 CrossRef CAS PubMed.
- D. A. Pei, G. S. Li, X. J. Jiang, J. L. Xu and H. W. Liu, Chin. J. Card. Pacing. Electrophysiol., 1995, 9, 207–209 Search PubMed.
- Y. P. Wang, W. Z. Chen, X. L. Wang and Z. Hua, Acta Pharmacol. Sin., 1996, 31, 581 CAS.
- X. Huang, Y. Yang, J. Zhu, D. Xu, J. Peng and J. Liu, J. Cardiovasc. Pharmacol., 2012, 59, 77–83 CrossRef CAS PubMed.
- X. Fan, Y. Chen, J. Xing, P. Wu, H. Chen, J. Yang, J. Zhang, X. Wang and C. Huang, J. Ethnopharmacol., 2012, 139, 42–51 CrossRef CAS PubMed.
- Y. Q. Wei, H. Yang, W. J. Liu and H. Chao, Chin. J. Clin. Pharmacol. Ther., 2011, 16, 784–785 Search PubMed.
- X. Gao, J. Zhu, Y. M. Yang, J. D. Li, Z. M. Yang and J. H. Liu, Chin. J. Cardiol., 2007, 35, 151–154 Search PubMed.
- J. Zhu, P. Li, Y. M. Yang, H. Q. Tan, T. H. Hu, X. Q. Yuan, G. G. Wang, L. X. He, L. M. Kang, J. D. Li and Y. C. Song, Chin. J. Cardiol., 2001, 29, 124 Search PubMed.
- R. B. Wang, S. X. Peng and W. Y. Hua, J. China Pharm. Univ., 1992, 23, 257 CAS.
- R. B. Wang, S. X. Peng and W. Y. Hua, Acta Pharmacol. Sin., 1993, 28, 583–593 CAS.
- B. Borcsa, L. Fodor, D. Csupor, P. Forgo, A. Molnár and J. Hohmann, Planta Med., 2014, 80, 231–236 CrossRef CAS PubMed.
- J. F. Zhang, Y. Li, F. Gao, L. H. Shan and X. L. Zhou, J. Asian Nat. Prod. Res., 2019, 21, 716–724 CrossRef CAS PubMed.
- M. Y. Ren, Q. T. Yu, C. Y. Shi and J. B. Luo, Molecules, 2017, 22, 267 CrossRef PubMed.
- D. K. Zhao, X. Q. Shi, L. M. Zhang, D. Q. Yang, H. C. Guo, Y. P. Chen and Y. Shen, Chin. Chem. Lett., 2017, 28, 358–361 CrossRef CAS.
- X. X. Liang, Y. Y. Gao and S. X. Luan, RSC Adv., 2018, 8, 23937–23946 RSC.
- K. Wada, E. Ohkoshi, Y. Zhao, M. Goto, S. L. Morris-Natschke and K. H. Lee, Bioorg. Med. Chem. Lett., 2015, 25, 1525–1531 CrossRef CAS PubMed.
- K. Wada, M. Hazawa, K. Takahashi, T. Mori, N. Kawahara and I. Kashiwakura, J. Nat. Prod., 2007, 70, 1854–1858 CrossRef CAS PubMed.
- K. Wada and H. Yamashita, Molecules, 2019, 24, 2317 CrossRef CAS PubMed.
- M. Hazawa, K. Takahashi, K. Wada, T. Mori, N. Kawahara and I. Kashiwakura, Invest. New Drugs, 2011, 29, 1–8 CrossRef CAS PubMed.
- K. Wada, S. Ishizuki, T. Mori, E. Fujihira and N. Kawahara, Biol. Pharm. Bull., 2000, 23, 607–615 CrossRef CAS PubMed.
- K. Wada, Yakugaku Zasshi, 2002, 122, 929 CrossRef CAS PubMed.
- K. Wada, S. Ishizuki, T. Mori, E. Fujihira and N. Kawahara, Biol. Pharm. Bull., 1998, 21, 140–146 CrossRef CAS PubMed.
- W. C. Wang and J. W. Michael, Flora of China, 2001 Search PubMed.
- J. F. Zhang, L. Chen, S. Huang, L. H. Shan, F. Gao and X. L. Zhou, J. Nat. Prod., 2017, 80, 3136–3142 CrossRef CAS PubMed.
- A. González-Coloma, M. Reina, A. Guadaño, R. Martínez-Díaz, J. G. Díaz, J. García-Rodriguez and M. Grandez, Chem. Biodiversity, 2004, 1, 1327–1335 CrossRef PubMed.
- A. González-Coloma, A. Guadano, C. Gutiérrez, R. Cabrera, E. de la Pena, G. de la Fuente and M. Reina, J. Agric. Food Chem., 1998, 46, 286–290 CrossRef PubMed.
- M. Reina and A. González-Coloma, Phytochem. Rev., 2007, 6, 81–95 CrossRef CAS.
- Y. Jiang, H. Q. Wu and J. H. Liu, Chin. Tradit. Herb. Drugs, 1987, 18, 23–26 Search PubMed.
- I. A. Bessonova and S. A. Saidkhodzhaeva, Chem. Nat. Compd., 2000, 36(5), 419–477 CrossRef CAS.
Footnote |
† Electronic supplementary information (ESI) available. See DOI: 10.1039/d1ra07173d |
|
This journal is © The Royal Society of Chemistry 2021 |