DOI:
10.1039/D1RA04434F
(Review Article)
RSC Adv., 2021,
11, 28838-28850
A review of shape memory polymers based on the intrinsic structures of their responsive switches
Received
8th June 2021
, Accepted 23rd August 2021
First published on 26th August 2021
Abstract
Shape memory polymers (SMPs), as stimuli-responsive materials, have attracted worldwide attention. Based on the history and development of SMPs, a variety of reports about SMPs in recent years are summarized in this paper. The responsive switches are analyzed and divided into two kinds according to their intrinsic structures: physical switch and chemical one. Then, detailed classification and comprehensive discussion of SMPs are further elaborated, based on the intrinsic structures of responsive switches and stimulation types. Finally, the development and prospect of SMPs are objectively predicted and forecasted.
1. Introduction
Animals, plants and other organisms in nature have the ability to adapt themselves to different environments by altering their own characteristics. Inspired by nature, scientists have designed and manufactured some intelligent materials that can perceive environmental stimuli, analyze and process information, make judgments and take corresponding measures. Shape memory materials, including shape memory alloys,1 shape memory ceramics,2 shape memory polymers (SMPs),3 are a class of intelligent materials. SMPs are one kind of the most important shape memory materials due to their superior properties (e.g., strong designability of molecular structure, excellent flexibility, good processability, and light weight) and broad application prospects, which have been greatly developed over the past few decades.4
In recent years, with the rapid development of artificial intelligence, there has been a worldwide upsurge of research interest in SMPs. SMPs, being of the functions of self-monitoring, self-repairing and self-adaptation, are the important basic materials for intelligent autonomous system. However, much higher and more comprehensive requirements for SMPs are put forward due to the demands of higher intelligence, more complex environments and wider applications. Thus, SMPs will not only face new opportunities but also be confronted with greater challenges. Now, great efforts have been done on shape memory materials and researchers have also published review articles about SMPs from different perspectives (e.g., matrix resins, driving types, nanocomposites and applications).4,5 The responsive switches are basic to the designs, manufactures and applications of SMPs. Based on summary and analysis of the development history and research progress for SMPs, the nature of the responsive switches is disclosed in this review, who is the reversible interaction of physics or chemistry among polymer molecular chains or chain segments. And detailed classification and comprehensive discussion of SMPs are further elaborated according to the intrinsic structures of responsive switches and stimulation types (see Fig. 1), which is not only conducive for a better understanding of SMPs, but also helpful in designing or developing novel SMPs.6
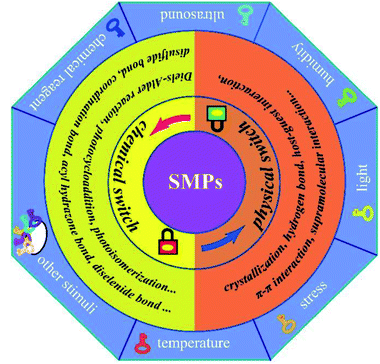 |
| Fig. 1 Schematic diagram of the classification of SMPs based on the intrinsic structures of their responsive switches. | |
2. History of SMPs
As far as we know, it has been nearly 100 years since the first discovery of shape memory materials, a kind of gold–cadmium alloys observed by Olander Sweden in 1932. Once heated to a threshold temperature, the deformed alloys could automatically revert to its original shape. Then, the concept of “elastic memory” was firstly proposed by Vernon in 1940s. In 1950s, Charles accidentally found the shape memory behaviours (shape fixation and shape recovery) of a chemical cross-linked polythene, opening the door for further study and applications of SMPs. It was an important milestone that the first commercial product of SMPs, thermal-shrinkable polyethylene pipe, came out in 1960. In 1970, National Aeronautics and Space Administration launched the application of SMPs in aerospace industry, and then restarted the research program for SMPs. In 1990, many kinds of SMPs were developed, such as polyolefin, copolymer, thermoplastic elastomer and polyester-alloy.7,8
In the last 30 years, SMPs have been paid great attention to by academic and industry scientists. There are many representative research findings, and a large number of SMPs have been developed for the requirements of applications.9,10 The triggers of responsive switches in SMPs have been well studied, based on different external stimuli (e.g., temperature, pH, chemical reagent, light, electricity, magnetism, pressure, humidity, ultrasonic or combinations thereof). Shape memory nanocomposites have been developed greatly in especial for their shape memory behaviours can be remotely controlled via light-thermal conversion, or magnetic-thermal conversion, or electro-thermal conversion, or acoustic-thermal conversion of nanomaterials. With the advent of artificial intelligence, more and more attention will be paid to SMPs.11,12 There are, of course, plenty of scientific issues about SMPs that need to be answered.
3. The critical components of SMPs and the intrinsic structures of their responsive switches
Since the first SMP came into being, scientists have taken a lot of efforts to understand the mechanisms of shape memory behaviours.13–17 Although many mechanisms of SMPs based on responsive switches, or external stimuli, or phase transformation types are proposed, the changes in nature—the changes in physical or chemical structures of SMPs during shape memory processes—should be disclosed. It is generally believed that SMPs are composed of a stable phase, memorizing the original shape and preventing the polymer from flowing, and a reversible phase, hardening as the disappearance of stimuli (e.g., temperature) and softening under stimuli. The stable phase, containing a stable network structure, is also a major contributor to the mechanical strength of a SMP. It has been shown that the entropic energy can be stored in the stable phase as a SMP is deformed from the original shape to a temporary one. When the stimulus on a temporarily deformed SMP is enough to trigger its responsive switch, the driving force generated from the stored energy prompts the SMP specimens to quickly revert to its original shape. The reversible phase is usually the linear chain structure with weak interchain interaction or the weakly cross-linked structure that is easily deformed (see Fig. 2). When the strength of a stimulus exceeds the switch threshold, the intermolecular interactions in the reversible phase are dropped to a very low level, and large deformation will occur under small external forces or even the driving force generated by the stored energy. The responsive switch is often included in reversible phase. Thus, as to complete a shape memory process, a SMP must have three critical components. The first is a stable phase (3D network structure), fixing the permanent shape of a SMP.4,18 The second is a reversible phase (reversible structure) that can be easily deformed in response to external stimuli.4,19 The third is a responsive switch that can open or close the door of shape deformations. Of course, an external stimulus is also the necessary factor.
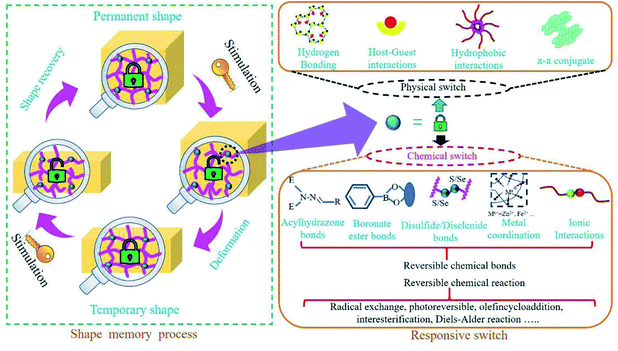 |
| Fig. 2 Schematic diagram of shape memory process and responsive switches of SMPs. | |
At molecular level, the responsive switches just correspond to the physical or chemical weak interactions (compared with the strong interactions of covalent bonds) among the molecular chains or chain segments of the reversible phase, which are the key factors for design and preparation of SMPs. One should note that the commonly called temperature switches of SMPs are generally referred to the temperature thresholds of shape memory recovery (see Fig. 2). Therefore, the intrinsic changes of SMPs during shape memory process involve the interconversion of dissociation and reconstruction for these weak interactions, the limited movements of the molecular chains or segments in reversible phase, and the variation of entropic energy stored in the network structure. Hu et al.4 have explained the responsive switches from phase level and molecular level. At phase level, glass transition temperature (Tg), melting temperature (Tm) and isotropic temperature (Ti), all can be used as switch thresholds. At molecular level, these intermolecular reversible factors such as non-chemical bond interactions, aggregation effects and chemical bonds can also be used as shape memory switches, that is, the shape memory switches can be physical, or chemical, or both (see Fig. 2).
Physical switches are usually resulted from some physical interactions20 (e.g., hydrogen bonding, supramolecular interaction, π–π interaction) and chemical switches from some reversible chemical bonds21–26 (e.g., ionic bonds, metal coordination, disulfide bonds, acylhydrazone bonds, diselenide bonds, boronate ester bonds, oxime bonds, hydrozone bonds, imine bonds, thioester bonds) or reversible chemical reaction27–30 (e.g., radical exchange reaction, photoreversible olefin cycloaddition, interesterification, Diels–Alder (DA) reaction). Thus, a variety of reports about SMPs in recent years are classified and discussed in the following text, based on the intrinsic structures of responsive switches—the intermolecular interactions of physics or chemistry.
4. Classifications of SMPs
For better understanding the recent research of SMPs, the SMPs are divided into two kinds: one based on physical switches and the other on chemical switches (see Table 1). Now that shape memory behaviours can be triggered by responsive switches, each type of the SMPs is further subdivided and discussed according to external stimuli (e.g., temperature, stress, light, ultrasound, humidity, chemical reagent) that can turn on or turn off the switches.
Table 1 Overview of selected examples of responsive switches and corresponding stimulation types
|
Types of the switches |
Stimulation types |
Reference |
Physical switches |
Melting transition |
Temperature, light, microwave, electricity |
34, 37, 41, 59–63 and 67 |
Glass transition |
Temperature, magnetism, ultrasound, humidity |
35, 36, 38–40, 42, 43, 62, 64, 68–70 and 75 |
Hydrogen bonds |
Temperature, stress, light, humidity, carbon dioxide, pH, non-aqueous solvents |
44, 46–52, 54–58, 65, 66 and 71–76 |
Host–guest interactions |
pH |
20 |
Hydrophobic interactions |
pH, dimethyl sulfoxide |
80 and 84 |
π–π interaction |
Temperature |
45 and 53 |
Chemical switches |
Disulfide bonds |
Temperature, hydrogen peroxide |
86 and 121 |
Diels–alder linkage |
Temperature, ultrasound, light |
87, 98 and 106 |
Metal coordination |
Light, ultrasound, complexing agent, electricity |
88–91, 107, 108, 110–114 and 123 |
Reversible photodimerization linkage |
Light |
99 |
Photocycloaddition linkage |
Light |
100 |
cis–trans isomerization |
Light |
101 |
Borate ester bonds |
pH, acetic acid solutions |
115, 116, 118 and 119 |
Acylhydrazone bonds |
pH |
117 |
Donor–acceptor interactions |
Hydrogen peroxide |
120 |
4.1. SMPs based on physical switches
In general speaking, SMPs based on physical switches are those, whose responsive switches are associated with reversible physical interactions and may be triggered by external stimuli such as temperature, stress, light, ultrasound, humidity, chemical reagent, or combinations thereof.
4.1.1. Temperature response. Temperature is the most achievable and effective stimulus to trigger the responsive switches of SMPs.31 Temperature rise of materials can be obtained through direct or indirect heating. Direct heating means heating materials by heat conduction or heat radiation. Indirect heating is realized by energy conversion (e.g., light-thermal conversion, magneto-thermal conversion, electro-thermal conversion, and acoustic-thermal conversion) of the fillers integrated in polymer matrices. Nanomaterials are one kind of the most commonly used fillers for energy conversion. High temperature can induce phase transitions and dissociations of reversible physical interactions.
4.1.1.1 Temperature induced phase transition. It has been shown that phase transition is a commonly used responsive switch in SMPs. The phase transition temperature (Ttrans) as a switch threshold for reversible deformations might be glass transition temperature (Tg), or melting temperature (Tm), or isotropic temperature (Ti).32,33 Below the Ttrans, the movements of polymer molecular chains or chain segments are restricted and the temporary shape is fixed. As the stimulation temperature rises above the Ttrans, the movement of molecular chains or chain segments is intensified, and then the deformed SMP should restore to its initial shape.For example, polycaprolactone (PCL) has been extensively discussed for its crystalline regions can play the role of network nodes and be used as the responsive switch. However, the Tm of PCL is above the human body temperature, limiting its application in biomaterials. An effective method was applied to decrease the Tm of a cross-linked PCL to around 33 °C via controlling the branching degree and molecular weight.34 Beside the entropic energy stored in polymer network, the conformation energy stored in polymer molecular chains can also be used as the driving force of shape recovery. A SMP, whose main chain was polyacetylene in helical state like a spring and side chains were octadecyl groups like “comb teeth”, was synthesized (see Fig. 3a).35 The energy stored in stretched “springs” served as driving energy of shape recovery and the phase transition of “comb teeth” was regarded as the responsive switch. To meet the need of application at high temperature, a SMP of SPEEK (sulfonated polyether ether ketone) ionomer was prepared.36 In this strategy, the Tg of this SMP was improved by the Coulomb force between metal ions and sulfonate anions, endowing a switch temperature from 224 to 288 °C. The higher the valence state of the metal ions is, the better shape memory behaviours and higher modulus this SMP has.
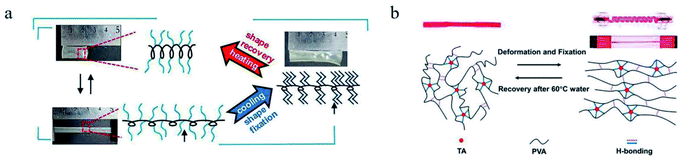 |
| Fig. 3 (a) The shape memory process and mechanism of a SMP with spring-like main chains and comb teeth-like side chains.35 Copyright 2019. Reproduced with permission from American Chemical Society. (b) Shape memory mechanism of the PVA–TA Hydrogel.46 Copyright 2016. Reproduced with permission from American Chemical Society. | |
In general, it is hard for pure SMPs to satisfy the comprehensive requirements of different industries. Composite technology is the most effective mean in the functional modification of SMPs.37 A SMP composite excited by microwave (MV) was prepared by introducing zero-dimension silicon carbide (SiC) into polyvinyl alcohol (PVA) matrix.38 The rapid heating of this SMP composite was achieved via the MV-thermal conversion of SiC nanofiller, and thus the rapid shape recovery was also realized. The nonuniform dispersion of nanofillers in polymer matrices is a serious problem for the preparation of SMP composites with superior properties. By using zinc dimethacrylate (ZDMA) as a compatibilizer as well as in situ reinforcer, a SMP composite of ferroferric oxide (Fe3O4)/carboxylic styrene butadiene rubber (XSBR) with uniform dispersed Fe3O4 particles and magnetic-thermal conversion was achieved.39 The mechanical properties and Tg of this SMP composite were enhanced for the additional ionic cross-linked points generated by the bridging role of ZDMA between Fe3O4 particles and XSBR matrix. The doping technology of SMPs by using conductive polymers is a feasible method to realize electro-thermal response of shape memory behaviours. A shape memory polylactic acid (PLA) microfiber membrane coated with a layer of conductive polypyrrole was synthesized via combining electrostatic spinning with chemical vapor polymerization technologies, whose shape memory behaviour was based on the rapid electro-thermal response.40 In addition to the visible shape memory behaviours, those on microscale can be also applied in surface topography of polymer.41 In resent research, some SMPs with tunable wettability have been developed via controlling their surface microstructure through shape memory functions.42,43
4.1.1.2 Temperature induced dissociation of non-chemical bonds. The responsive switches based on non-chemical bonds have been applied in SMPs.44,45 These SMPs have at least two types of cross-linking networks, physically network and chemically one. The former acts as the reversible phase, while the latter serves as the stable phase. The tannic acid (TA) can form intramolecular or intermolecular hydrogen bonds in some hydrogels. This hydrogen bond networks can be dissociated or recombined. A SMP hydrogel was synthesized via blending of PVA and TA. The stronger hydrogen bonds between PVA and TA acted as the cross-linkage structure for memorizing the permanent shape, while the weaker hydrogen bonds among PVA chains served as the responsive switch (see Fig. 3b).46–49 Analogously, a shape memory polybenzoxazine film consisting of arylamine mannich-type linkage was synthesized, whose chemical structure is different from that of the conventional polybenzoxazines.50 Mannich polybenzoxazines possessed more weak hydrogen bonds of –OH⋯π that only existed at relatively lower temperatures and served as the responsive switch. Moreover, a SMP was prepared via crosslinking of polydimethylsiloxane and poly(ethylene glycol) diglycidyl ether.51 When the temperature rose above 37 °C, the weak hydrogen bonds among molecular chains or chain segments were disengaged, which led to the improvement for mobility of molecular chains or chain segments, as well as the shape recovery. Another SMP hydrogel was developed by the polymerization the acrylamide in an elastin-like polypeptide (ELP) solution.52 This SMP hydrogel, holding dual networks of a physically cross-linking ELP and a chemically cross-linking polyacrylamide (PAM), exhibited excellent shape memory function, wherein the hydrogen bonds among the ELP molecular chains acted as the responsive switch. From the discussion above, the design of SMPs based on hydrogen bonds mostly relies on three groups: pyridine, ureidopyrimidine, and carboxylic acids.Besides hydrogen bonds, π–π interactions, weakly non-chemical bonds, can play important roles in shape memory properties by acting as the physical cross-linked points. A series of polyimides (PIs) with different chain structures were synthesized by a two-step method, showing a shape recovery ratio (Rr) of above 93%.53
4.1.2. Stress response. The stress-responsive SMPs are those, whose responsive switches are triggered by stress. A SMP of poly-2-ureido-4[1H]-pyrimidone (UPy) was prepared,54–56 whose temporary shape can be fixed through dimerization of unfolded UPy units, and shape recovery can be realized via dissociation of hydrogen bonds under stress. Fang et al.57 synthesized a kind of stress-responsive photonic crystals basing on photocured copolymers of ethoxylated trimethylolpropane triacrylate (ETPTA) and polyethylene glycol diacrylate (PEGDA) oligomer (see Fig. 4). A disordered temporary shape can be achieved from a 3D ordered permanent shape at ambient conditions by evaporating water from the templated macropores. And the shape recovery accompanied by reconfiguration of photonic crystals can also be realized by a tiny contact pressure or even the surface tension gradient generated during solvent evaporating. They further studied a dynamic direct writing technology of ethoxylated trimethylolpropane triacrylate-co-polyethylene glycol diacrylate (ETPTA-co-PEGDA) copolymer by integrating the well-established templating nanofabrication and shape memory function.58 The deformation and recovery process of this SMPs are attributed to the mutual transformation of the external energy provide by stress and the internal one stored in SMP system.
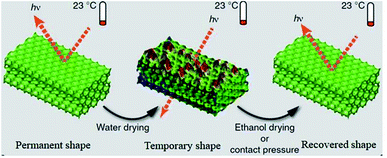 |
| Fig. 4 A schematic illustration of the shape memory process of a SMP accompanied by reconfiguration of photonic crystals.57 Copyright 2015. Reproduced with permission from Springer Nature. | |
4.1.3. Light response. Light-responsive SMPs have attracted wide attention due to their unique advantages such as contactless and remote controlling, local application, and the ability free of surrounding intervention. They have an important application prospect in biomedical devices, soft drive machinery or wearable electronic devices. Most photoinduced shape memory behaviours are triggered by temperature thresholds, containing phase transition or dissociation of non-chemical bonds. Because the temperature variations of these SMPs are local and slight during shape memory progress, they are defined as light-responsive SMPs here.
4.1.3.1 Light-induced phase transition. The doping technology of SMPs by using light-thermal conversion fillers is a feasible method to realize light response of shape memory behaviours.59 By using the reinforcing and light-thermal conversion functions of dopamine particles, Yang et al.60 synthesized a SMP hydrogel with ultrafast near infrared ray (NIR) response. Plasmonic silver nanoparticles (AgNPs) as a class of light-thermal conversion nanofillers have also been applied in SMPs (see Fig. 5a).61 In this system, the “nanorod-like” structure of cellulose nanocrystalline (CNC)–g-AgNPs is beneficial to the generation of plasmonic coupling between two individual NPs. Rare earth organic complexe (REOC) with unique optical, electrical, magnetic and thermal functions, was blended with polymer to prepare a light-responsive REOC/SMP composite.62 In this work, the existence of hydrogen bonds between nitrate groups of Sm (TTA) Phen (NO3)3(STPN) and carboxyl groups of cross-linked poly(methyl methacrylate-co-itaconic acid) (P(MMA-co-IA)) significantly improved the dispersion of STPN in polymer matrix. A SMP was developed by blending carbon dots and PVA.63 The shape memory behaviours of this SMP could be quickly implemented by the melting process of PVA crystalline regions under 650 nm laser irradiation. In the above discussed SMPs, the light-thermal conversion fillers also offered additional physical cross-linked points, which improved the shape memory performance.
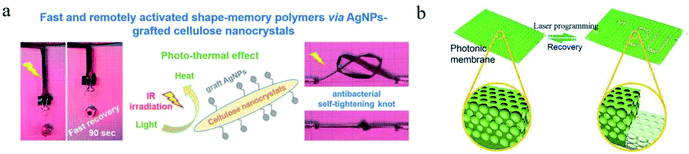 |
| Fig. 5 (a) Digital images of SMP films actuated upon IR irradiation.61 Copyright 2018. Reproduced with permission from American Chemical Society. (b) Schematic of shape memory process of photonic patterns.65 Copyright 2019. Reproduced with permission from Royal Society of Chemistry. | |
However, there are some drawbacks in doping technology of SMPs by using light-thermal conversion fillers due to the poor compatibility of light-thermal fillers in polymers. Recently, a kind of benzoxazine/epoxy (V-fa/ECO) copolymer was prepared from bio-based raw materials of vanillin, furfurylamine, paraformaldehyde, and epoxidized castor oil. Shape memory behaviours of this copolymer could be triggered by NIR without using any light-thermal fillers.64 The mechanism was that light-thermal effect of π–π interactions among polymer chains provided the energy for shape recovery and glass transition of the copolymer.
4.1.3.2 Light-induced dissociation of the non-chemical bonds. A photonic membrane with shape memory properties was prepared through integrating the copolymer of PEGDA-tetrafunctional aliphatic polyurethane acrylate (PUA) and Cu2S nanoparticles.65,66 The internal stress stored in this SMP could be released through light-thermal effect of Cu2S nanoparticles, which improved the motion of the molecular segments in PEGDA-PUA copolymer and induced shape recovery (see Fig. 5b).
4.1.4. Ultrasound response. Ultrasound can penetrate much more deeply in the materials, triggering shape memory behaviours in a remote and controlled way. Basing on an ultrasound-responsive polyurethane (PU), Han et al.67 studied a controlled drug release technology. Under high intensity focused ultrasound (HIFU), the shape recovery of the blend system of PU and drug brought about the enlargements of drug-to-body fluid contact and the drug diffusion coefficient. By using copolymer of methyl methacrylate and butyl acrylate, a similar research work has been done (see Fig. 6).68 The shape memory mechanism was that ultrasonic effect rose the temperature of the SMPs above their Tg,69 leading to the trigger of their responsive switches.70
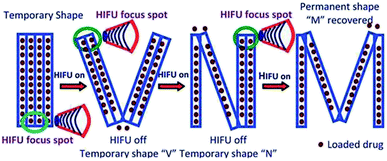 |
| Fig. 6 Schematic illustration of the HIFU-enabled shape memory process and drug-controlled release.68 Copyright 2012. Reproduced with permission from Royal Society of Chemistry. | |
4.1.5. Humidity response. Thermal-responsive SMPs have always been the focus of research, but there are disadvantages for biomedical field. For example, if the responsive temperature of SMPs is higher than the body temperature, it will damage cells and tissues. Humidity is regarded as a mild stimulus, which will be conducive to avoid the negative effects of direct heating.71,72 A humidity-responsive SMP hydrogel based on polyacrylamide-chitosan (PAM-CS) was synthesized through free radical polymerization.73 The temporary shape of this SMP hydrogel was fixed due to the formation of the physical microcrystalline crosslinking or chain entanglement as soaked in alkaline or saline solutions. These physical cross-linked networks could be dissociated by H2O or HCl solution. Gu et al.74 developed a humidity-responsive SMP hydrogel basing on PVA/silk hybrids (see Fig. 7). In this SMP hydrogel, the β-sheet crystals were regarded as network nodes to memory the permanent shape, while the hydrogen bonds within the amorphous regions of silks served as a switch for the reversible deformations. Some other humidity-responsive SMP composites were synthesized by introducing nanoparticles into the polymer matrices.75,76 The nanoparticles were regarded as the additional physical cross-linked points to improve the shape memory properties of composite. The humidity-responsive shape memory effects (SMEs) are attributed to the plasticizing effect and the competitive hydrogen bonds. That is to say, the absorbed water in the composites can reduce the entanglement and the interaction of molecular chains, which will improve the movability of molecular chains and result in the shape recovery.
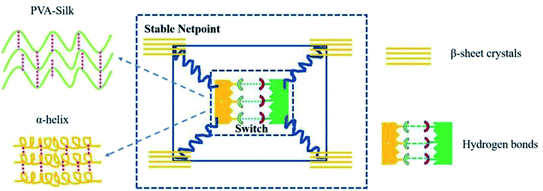 |
| Fig. 7 Structure model of the shape memory PVA/silk hybrid.74 Copyright 2019. Reproduced with permission from Elsevier. | |
4.1.6. Chemical reagent response. Chemical reagent can also act as external stimuli to trigger the physically responsive switches of SMPs.77 Chemical reagent responsive SMPs have been concerned due to their unique advantages such as excellent controllability and wide applied fields.
4.1.6.1 Carbon dioxide (CO2) response. Xu et al.78 synthesized a CO2-responsive hydrogel via copolymerization of 2-vinyl-4,6-diamino-1,3,5-triazine and N,N-dimethylacrylamide with polyethylene glycol diacrylates (see Fig. 8a). The physical cross-linkage generated from hydrogen bonds among diaminotriazine (DAT) groups served as a switch for the reversible deformation. The dissolved CO2 induced the protonation of DAT segments, leading to the dissociation of hydrogen bonds.
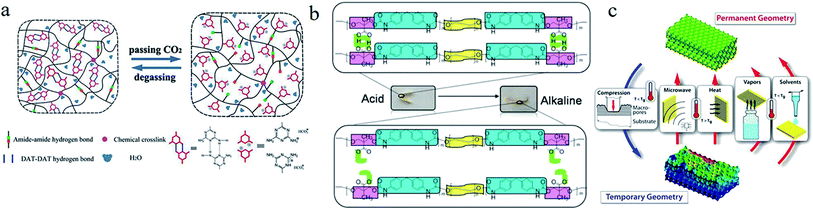 |
| Fig. 8 (a) The CO2-triggered shape memory mechanism of a hydrogel with double hydrogen bonds.78 Copyright 2015. Reproduced with permission from John Wiley & Sons Ltd. (b) Schematic route of the pH sensitive shape memory behavior of PEG-30%-MDI-DMPA.81 Copyright 2016. Reproduced with permission from Royal Society of Chemistry. (c) A schematic diagram of the microstructural transitions between the 3D ordered permanent shape and disordered temporary shape of PU-based SMPs with solvent response.85 Copyright 2017. Reproduced with permission from American Chemical Society. | |
4.1.6.2 pH response. pH induced SMBs are fast and controllable. Li et al.79 developed a pH-responsive SMP composite by blending of poly(ethylene glycol)-poly(ε-caprolactone)-polyurethane (PECU) and functionalized CNC with pyridine groups or carboxyl groups. The hydrogen bonds between pyridine groups and hydroxyl groups were just the switch of this SMP composite, who were formed in alkali condition and weaken in acidic condition due to the protonation of pyridine group. A SMP hydrogel with both pH-response and temperature-response was developed by grafting dansyl groups (Dns) into the networks of polyacrylamide (PAAM).80 In this work, cross-linked PAAM served as stable networks, while the hydrophobic association of Dns groups that could be modulated by pH or temperature was designed as the responsive switch. By using carboxylic dimerization as a switch, Song et al.81 synthesized a pH-responsive SMP of polyethylene glycol-4,4′-diphenylmethane diisocyanate-dimethylol propionic acid (PEG-MDI-DMPA) (see Fig. 8b). The carboxylic dimers in polymer chains were dissociated under alkaline condition (pH 9) and rebuilt under acidic condition (pH 2). A SMP was prepared by cross-linking of β-cyclodextrin-modified alginate (β-CD-Alg) and diethylenetriamine (DETA)-modified alginate (DETA-Alg),82 in which inclusion complexes (ICs) of β-CD and DETA served as a reversible phase, and the cross-linked alginate was regarded as a stable phase. It was shown that this SMP could be programmed into temporary shape at pH 11.5 and recover to its original shape at pH 7.
4.1.6.3 Solvent response. A hydrogel with solvent-responsive SMBs was synthesized by copolymerization of dopamine methacrylamide, N,N′-dimethylacrylamide, and N,N′-methylenebisacrylamide cross-linker.83 In this hydrogel, hydrophobic association was used as responsive switch, who can be dissociated in dimethyl sulfoxide (DMSO) and rebuilt in H2O.However, the catechol group was easy to be oxidized in aqueous solution, resulting in unstable mechanical properties of this hydrogel. By copolymerization of hydrophobic monomers, butyl methacrylate and methacrylic acid, in a mixed solvent of DMSO and H2O. Liao et al.84 synthesized a series of solvent-responsive gels with stable mechanical properties. Fang and his cooperators85 reported a solvent-responsive SMP with reconfigurable/rewritable macroporous photonic crystals (see Fig. 8c). Its shape recovery accompanied by reconfiguration of ordered photonic crystals could be triggered by multiple stimuli (e.g., vapors, nonaqueous solvents, temperature, and MV radiation). Tg of this SMP were decreased for the plasticizing effect of the solvent molecules permeating in polymer matrix.
4.2. SMPs based on chemical switches
SMPs based on chemical switches mainly refer the ones, whose responsive switches are based on reversible chemical reactions or reversible chemical bonds.
4.2.1. Temperature response. A polymer adhesive with dynamic disulfide bonds exhibited two levels of SMEs.86 One was attributed to the reversible phase of the crystalline domains. The other was associated with the dynamic disulfide bonds. When heated to about 150 °C, the viscosity of the adhesive declined significantly for the temporary breaking of the dynamic disulfide bonds, which was benefit to the wetting of the adhesive on substrate. After cooled to room temperature, new disulfide bonds in the adhesive were built and a strong adhesion was achieved. Zhang et al.87 synthesized a SMP that contained network nodes of Diels–Alder (DA) adducts. This SMP could undergo shape memory process in solid state at intermediate temperatures via breaking and recombining part of the DA adducts.
4.2.2. Light response. Coordination bonds provide a reversibility which can be used for the design of SMPs. Burnworth et al.88 synthesized a metallosupramolecular SMPs that could be healed by light irradiation. Under UV irradiation, the dissociation of the multidentate ligand (Zn-Ns) leaded to a sharp drop in the viscosity of polymer, resulting in the selective healing of cracks.88,89 Feng et al.90 synthesized an organic cross-linked poly(acrylamide-co-N-vinylimidazole) hydrogel by using photoacid generator (PAG) as a trigger (see Fig. 9a). The temporary shape of this hydrogel could be fixed by the coordination bonds between the imidazole groups and metal ions. Under UV irradiation, PAG as proton donor could reduce the pH of this hydrogel below the acid dissociation constant (pKa) of imidazole groups, resulting in the disassociation of these coordination bonds, that is the reason of shape recovery. Bai et al.91 synthesized a series of shape memory ionomers by blending PVA, polyacrylic acid (PAA) and bridge metal ions (e.g., Fe3+, Cu2+, Co2+), in which the responsive switches were the coordination bonds between metal ions and carboxyl groups. These coordination bonds could be temporarily decomposed by light-thermal conversion of metal ions themselves and rebuilt after the disappearance of NIR light.
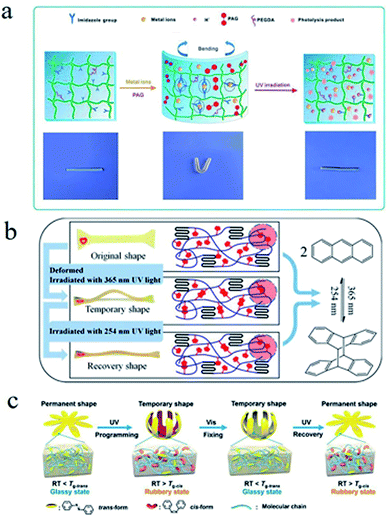 |
| Fig. 9 (a) The UV light-triggered shape memory mechanism of an organic cross-linked poly(acrylamide-co-N-vinylimidazole) hydrogel.90 Copyright 2015. Reproduced with permission from Royal Society of Chemistry. (b) The light-triggered shape memory process and mechanism of a PLA-PEG-A.100 Copyright 2016. Reproduced with permission from American Chemical Society. (c) The shape memory process and mechanism of an SMP with azobenzene molecule.103 Copyright 2018. Reproduced with permission from American Chemical Society. | |
In addition, photoinduced reversible chemical reactions can also be used in SMPs.92–97 A dynamically cross-linked elastomer hybrid with self-healing and shape memory properties was synthesized by Shi et al.98 In this hybrid, a reversible network was formed via DA reaction between macromolecular diene and carbon nanotubes (CNTs), and the reverse DA (rDA) reaction could be triggered by laser irradiation due to the light-thermal effect of CNTs. The network nodes between CNTs and furan-modified poly(styrene-block-butadieneblock-styrene) (SBS) could be rearranged for the liberation of SBS chains by rDA reaction, endowing the hybrids with self-healing ability. The combinations of DA reaction and rDA reaction could be used to reprogram the shape memory behaviours under laser irradiation or thermal treatment.
Banerjee et al.99 synthesized a light responsive self-healing polymer by using low molecular weight tri-arm polyisobutylene (PIB) with terminal groups of coumarin. The results showed that the reversible photodimerization of two adjacent coumarins could occur under UV irradiation of 365 nm, endowing this system with a stable macromolecular network. While under UV irradiation of 254 nm, the cross-linked PIB networks would be dissociated for the disaggregation of photodimers. Then the low viscosity PIB were flowing and filled the cut. Xie et al.100 designed a SMP of poly(L-lactide)-poly(ethylene glycol)-(anthracene group) (PLA-PEG-A) copolymer (see Fig. 9b). It showed that the shape fixation of samples was realized by the photo-crosslinking, the photocycloaddition reaction of anthracene groups under UV light of 365 nm. Irradiated by UV light of 254 nm, part of the cyclized structures could be disassociated, leading to the decrease of the cross-linked density and the shape recovery. A special SMP has been synthesized by functionalizing the terminal groups of star-shaped PCL with 7-hydroxypropoxy-4-methylcoumarin.101 This functionalized PCL could be cross-linked by the dimerization of coumarin groups under UV irradiation of 365 nm, and the cross-linked network could also be destroyed under UV irradiation of 256 nm, which was the basic reason for the light-responsive shape memory behaviour of this PCL. The cis–trans isomerization can be used as the switch of these SMPs. A swimming micro robot was designed by using the reversible process of light-responsive shape memory behaviours.102 In this work, the cis isomers of azobenzene (azo) chromophores in the polymer films of paddles could be transformed into trans ones under UV irradiation and the opposite will be carried out under visible irradiation, which brought about the alternant bends of the paddles in two opposite directions and pushed forward the micro robot in liquid. Moreover, the moving direction of the micro robot could be guided by light source. Tg of polymer with azo groups could be altered by photoisomerization, which has also been applied in SMP (see Fig. 9c).103 Under UV light irradiation (365 nm), Tg of this kind of SMPs is below room temperature for most of the azo groups are cis structure. While under visible light irradiation (530 nm), Tg is above room temperature for most of the azo groups are trans structure. Host–guest interactions between α-cyclodextrin and azo groups could be reversible broken and recombination by cis–trans isomerization of azo. A shape memory film composed of azo-grafted poly(acrylate acid) (azo-PAA), α-cyclodextrin modified alginate (α-CD-Alg) and calcium chloride was synthesized.104 In this SMP, the cross-linked network formed through network nodes—coordinate bonds of Ca2+-carboxyl groups—served as the stable phase and host–guest interactions between α-cyclodextrin and azo as the reversible switch. The SMP film could be programmed into temporary shapes as needed and recover to its permanent shape upon the stimuli of temperature.
4.2.3. Ultrasound response. Some chemical bonds, such as ester bond, disulfide bond, DA linkage, and coordinate bond,105 can be temporally destroyed by ultrasound, which is an ideal strategy for SMPs. A kind of PU containing functional groups of DA adducts was synthesized, being of the functions of shape memory and self-healing under HIFU (see Fig. 10).106 HIFU triggered rDA reactions and DA reshuffling reactions were the main reasons for the effective healing. Sijbesma et al.107,108 synthesized a coordination polymer composed of low-molecular-weight diphenylphosphine telechelic poly(tetrahydrofuran) and palladium(II) dichloride. The palladium(II)–phosphine ligand could be destroyed under ultrasonic and reconstituted as the disappearance of ultrasonic, who can be used as a responsive switch of this SMP.
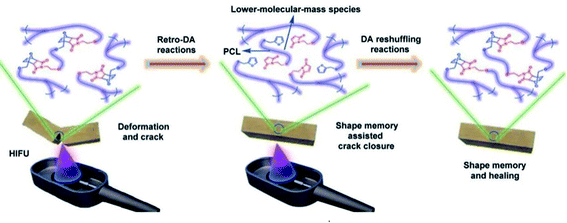 |
| Fig. 10 A schematic diagram of the healing of a PCL-based PU with DA linkage assisted by HIFU-triggered shape memory.106 Copyright 2014. Reproduced with permission from Royal Society of Chemistry. | |
4.2.4. Chemical reagent response.
4.2.4.1 Complexing agent response. Coordination bonds of metal ions have been used as the switches in SMPs to generate additional cross-linkages for fixation of temporary shapes.109 Some complexing agents can disrupt coordination bonds of polymer system by extracting metal ions, and these coordination bonds can be rebuilt when the metal ions are reincorporated.110 For example, a copolymer hydrogel was prepared by one-step photopolymerization of vinylimidazole (VI) and acrylonitrile (AN) in the presence of a polyethylene glycol-based cross-linker, named as PVI–AN hydrogel (see Fig. 11a).111 The spiral shape of PVI–AN specimen could be fixed by the cross-linked network formed via network nodes of Zn2+-imidazole coordination bonds. Once the hydrogel was transferred into EDTA solution, its cross-linked network would be disrupted for Zn2+ was captured by EDTA, inducing the realization of shape recovery. An interpenetrating network polymer of PAM and alginate was prepared by copolymerization of acrylamide (Am), host–guest cross-linker of azobenzene and α-CD in an aqueous solution of alginate.112 The temporary shape of this polymer could be fixed by Ca2+ and recover to its original shape via depriving Ca2+ by EDTA, due to the reversible coordination bonds of alginate–Ca2+. Zhang et al.113 synthesized a similar SMP with rhodium–phosphine ligand. The rhodium–phosphine coordination bonds could be destroyed by triphenylphosphine solution and rebuilt in [RhCl(1,5-cyclooctadiene)]2 solution. A SMP hydrogel with an interpenetrating network of physically cross-linked CMC and chemically cross-linked PAM could be fixed by chelation of CMC and Fe3+, and the original shape could be recovered by treatment of EDTA, oxalate or H2SO4 solution for the chelation of CMC and Fe3+ was destroyed.114
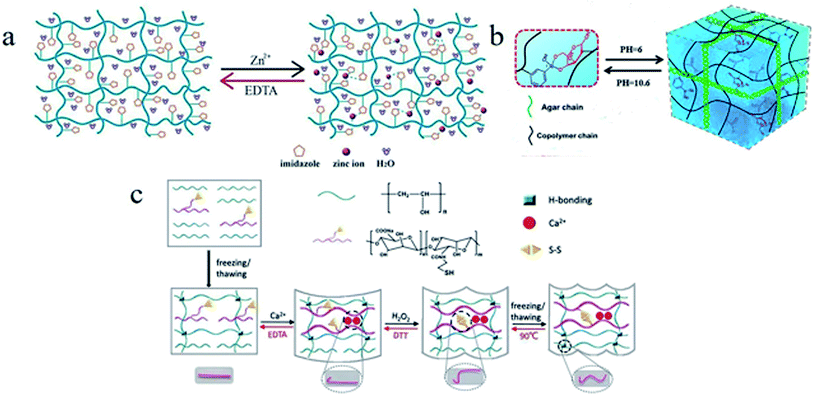 |
| Fig. 11 (a) A schematic diagram of the EDTA-triggered shape memory mechanism of a PVI–AN hydrogel.111 Copyright 2013. Reproduced with permission from Royal Society of Chemistry. (b) Mechanism of programmed shape memory process based on PBA–diol ester bonds.116 Copyright 2017. Reproduced with permission from American Chemical Society. (c) A schematic diagram of the shape memory process and mechanism of a SMP hydrogel with three different types of reversible interactions, including Ca2+ ligand, a thiol–disulfide, and the hydrogen bonding.121 Copyright 2018. Reproduced with permission from Royal Society of Chemistry. | |
4.2.4.2 pH response. A SMP hydrogel with a responsive switch of reversible phenylboronic acid (PBA)–diol ester bonds was reported.115 The temporary shape of this SMP hydrogel could be fixed in alkaline condition, and the shape recover was performed in acidic condition due to dissociation of the PBA–diol ester bonds. Moreover, Le et al.116 fabricated a SMP hydrogel with triple responses that corresponded to stimuli of EDTA, pH, and temperature, respectively. In this work, shape fixation and shape restoration were associated with PBA–diol ester bonds, acrylic acid (AAc)–Fe3+ coordination, and a physically cross-linked agar network (see Fig. 11b). A kind of hyaluronic acid-based injectable hydrogels with dual dynamic covalent bonds of acylhydrazone and disulfide linkages were synthesized.117 These hydrogels displayed pH-responsive shape memory behaviours due to the reversible acylhydrazone bonds and the osmotic driving for the concentration gradient between the network and surroundings. A hydrogel of the copolymer of acrylamide, acrylic acid, agar, and PVA exhibited multiple shape memory behaviours based on the coordination bonds of carboxy–Fe3+, borate ester bonds, and hydrogen bonds. pH response of this hydrogel was associated with dynamic borate ester bonds.118 A hydrogel was prepared by copolymerization of acrylamide, oxidized sodium alginate (OSA), and PVA, whose multiple-shape memory behaviours were based on imine, coordination, and borate bonds.119 A temporary shape could be fixed by a treatment of borax solution for borate bonds were formed, and original shape could be recovered by soaking in acetic acid solutions for the borate ester bonds were destroyed.
4.2.4.3 Redox agent response. By integrating DNA/bipyridinium dithienylethene (DTE) and CMC modified with dopamine and self-complementary nucleic acid, Li et al.120 prepared a multiple-responsive hydrogel. Shape memory behaviours of this hydrogel could be triggered by crown ether (CE), or visible light, or redox agent. In this hydrogel, the donor–acceptor interactions of dopamine/DTE would be dissociated by oxidation of sodium persulfate, and recombined by reduction of vitamin C. Tang et al.121 synthesized another multiple-responsive SMP hydrogel that contained three types of reversible interactions (Ca2+ complexation, disulfide bond, hydrogen bond) by blending cysteamine-grafted alginate (SA-SH) and PVA (Fig. 11c). A temporary shape of hydrogel could be fixed by using a treatment of hydrogen peroxide (H2O2), and the permanent shape could be recovered by introducing dithiothreitol (DTT). Wang et al.122 reported a shape memory blend of poly(ethylene oxide) (PEO), poly(acrylicacid) (PAA), and TA. The temporary shape of this blend could be fixed via the ionic cross-linkage of Cu2+–carboxylate coordination bonds. And the shape recovery could be performed for the breaking of Cu2+–carboxylate coordination bonds induced by oxidation of sodium metabisulfite. Recently, some scholars have realized shape memory behaviours by means of electric redox. Rapid shape memory behaviours of a hydrogel device in air were realized by using electro-redox reaction of Fe3+ and Fe2+, where the reversible coordination of Fe3+ ions and amine groups served as a responsive switch (see Fig. 12).123
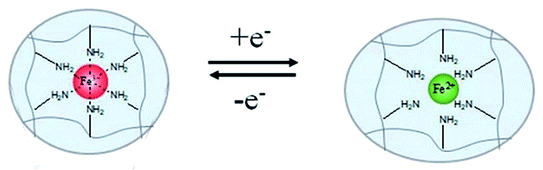 |
| Fig. 12 Mechanism of shape memory effect and rapid reversible actuation of hydrogels with electrochemically controlled local metal ion coordination and crosslinking.123 Copyright 2020. Reproduced with permission from Royal Society of Chemistry. | |
5. Conclusion and prospect
Based on deep analysis in the developments of SMPs, it is indicated that the nature of the responsive switch in SMPs is physical interaction or chemical one among polymer molecular chains or chain segments. For better understanding the mechanisms of their shape memory behaviours, the SMPs are divided into two kinds (one based on physical switches and the other on chemical switches) and in detail discussed in this paper, from of which it is shows that a lot of works have been conducted to improve the performance of SMPs and develop new types of SMPs. Although many shape memory mechanisms are deeply understood and a wide variety of SMPs have been developed, in order to satisfy the application requirements of intelligent materials, there is a long way to go. The SMPs with these properties such as high sensitivity, good responsive accuracy, multiple responsive switches, multifunctional responsive switches, and excellent mechanical characteristics, are needed in the future.
The design and development of new types of responsive switches may become an important part of research in SMPs in future. The development of responsive switches with high-precision, quantifiable and controllable responses is getting attention for the requirement of intelligent control in intelligent materials. Moreover, the multifunctional responsive switches that mean a switch can respond to different stimuli will be greatly developed. The organisms in nature can sensitively and accurately respond to the external stimuli for their precisely and artfully designed structures. Therefore, precise control of the composition and structure of materials in micro scale or even molecular level is another important part of research in SMPs. With the development of material design (e.g., material computation and design combined with computer techniques) and preparation technology (e.g., 4D printing technology), this goal will be achieved gradually.
Although SMPs cannot rival the response properties of organisms in nature, many characters of the former such as service behaviors in high or low temperature, vacuum, high pressure, and chemical environments are better than that of the latter. In the future, SMPs as a kind of intelligent materials will be greatly developed and widely applied.
Author contributions
Lide Yang: Investigation, Conceptualization, Methodology, Writing – Original draft preparation. Jiankun Lou: Investigation. Jianmin Yuan: Conceptualization, Methodology, Writing – Reviewing and Editing, Supervision, Validation. Jianru Deng: Methodology, Writing – Reviewing and Editing, Supervision, Validation.
Conflicts of interest
The authors declare that they have no known competing financial interests or personal relationships that could have appeared to influence the work reported in this paper.
Acknowledgements
We gratefully acknowledge the valuable cooperation of professor Shengpei Su and the members of his laboratory (Hunan Normal University, China).
Notes and references
- A. Cohadesa, N. Hostettlera, M. Paucharda, C. J. G. Plummer and V. Michaud, Compos. Sci. Technol., 2018, 161, 22–31 CrossRef.
- H. A. Rauch, Y. Chen, K. An and H. Z. Yu, Acta Mater., 2019, 168, 362–375 CrossRef CAS.
- W. Zhao, Z. Huang, L. Liu, W. Wang, J. Leng and Y. Liu, Compos. Sci. Technol., 2020, 203, 108563 CrossRef.
- J. Hu, Y. Zhu, H. Huang and L. Jing, Prog. Polym. Sci., 2012, 37, 1720–1763 CrossRef CAS.
- Y. Xia, Y. He, F. Zhang, Y. Liu and J. Leng, Adv. Mater., 2021, 33, 2000713 CrossRef CAS.
- F. G. Bonifacich, O. A. Lambri, V. Recarte, V. Sánchez-Alarcos and J. I. Pérez-Landazábal, Compos. Sci. Technol., 2021, 201, 108538 CrossRef CAS.
- D. Ratna and J. Karger-Kocsis, J. Mater. Sci., 2008, 43, 254–269 CrossRef CAS.
- T. Xie, Polymer, 2011, 52, 4985–5000 CrossRef CAS.
- S. Yang, Y. He, Y. Liu and J. Leng, J. Mater. Chem. C, 2020, 8, 303–309 RSC.
- Z. Cheng, D. Zhang, X. Luo, H. Lai, Y. Liu and L. Jiang, Adv. Mater., 2020, 33, 2001718 CrossRef PubMed.
- X. Kuang, D. J. Roach, J. Wu, C. M. Hamel, Z. Ding, T. Wang, M. L. Dunn and H. J. Qi, Adv. Funct. Mater., 2019, 29, 1805290.1–1805290.23 Search PubMed.
- C. Lin, J. X. Lv, Y. S. Li and F. H. Zhang, Adv. Funct. Mater., 2019, 29, 201906569 Search PubMed.
- M. Zare, M. P. Prabhakaran, N. Parvin and S. Ramakrishna, Chem. Eng. J., 2019, 374, 706–720 CrossRef CAS.
- J. Fan and G. Li, Nat. Commun., 2018, 9, 1–8 CrossRef PubMed.
- K. J. Wang, Y. G. Jia and X. X. Zhu, Macromolecules, 2017, 50, 8570–8579 CrossRef CAS.
- J. Hu and S. Chen, J. Mater. Chem., 2010, 20, 3346–3355 RSC.
- X. L. Wu, W. M. Huang, Y. Zhao and D. Zheng, Polymers, 2013, 5, 1169–1202 CrossRef.
- M. D. Hager, S. Bode, C. Weber and U. S. Schubert, Prog. Polym. Sci., 2015, 49, 3–33 CrossRef.
- E. Wang, Y. Wu, M. Z. Islam, Y. Dong, Y. Zhu, F. Liu, Y. Fu, Z. Xu and N. Hu, Mater. Lett., 2019, 238, 54–57 CrossRef CAS.
- Z. C. Jiang, Y. Y. Xiao, Y. Kang, J. Bang and S. Zhang, ACS Appl. Mater. Interfaces, 2017, 9, 20276–20293 CrossRef CAS PubMed.
- Y. Shi, M. Yoonessi and R. A. Weiss, Macromolecules, 2013, 46, 4160–4167 CrossRef CAS.
- S. B. Zhou, L. X. Lu, T. Tian and S. S. Wu, Polym. Chem., 2019, 10, 1920–1929 RSC.
- L. Ling, J. Li, G. Zhang, R. Sun and C. P. Wong, Macromol. Res., 2018, 26, 365–373 CrossRef CAS.
- S. Ji, W. Cao, Y. Yu and H. Xu, Adv. Mater., 2015, 27, 7740–7745 CrossRef CAS PubMed.
- W. N. Du, Y. Jin, S. Q. Lai, L. J. Shi, W. H. Fan and J. G. Pan, Polymer, 2018, 158, 120–129 CrossRef CAS.
- M. M. Perera and N. Ayres, Polym. Chem., 2020, 11, 1410–1423 RSC.
- H. Otsuka, K. Aotani, Y. Higaki and A. Takahara, Chem. Commun., 2002, 23, 2838–2839 RSC.
- M. Abdallh, C. Yoshikawa, M. Hearn and G. P. Simon, Macromolecules, 2019, 52, 2446–2455 CrossRef CAS.
- O. R. Cromwell, J. Chung and Z. Guan, J. Am. Chem. Soc., 2015, 137, 6492–6495 CrossRef CAS PubMed.
- M. J. Fan, J. L. Liu and X. Y. Li, Ind. Eng. Chem. Res., 2014, 53, 16156–16163 CrossRef CAS.
- J. R. Kumpfer and S. J. Rowan, J. Am. Chem. Soc., 2011, 133, 12866–12874 CrossRef CAS PubMed.
- M. Li, S. Fu, L. A. Lucia and Y. Wang, Compos. Sci. Technol., 2020, 199, 108371 CrossRef CAS.
- Z. J. Ding, L. Yuan, G. Z. Liang and A. J. Gu, J. Mater. Chem. A, 2019, 7, 9736–9747 RSC.
- M. Ebara, K. Uto, N. Idota, J. M. Hoffman and T. Aoyagi, Adv. Mater., 2012, 24, 273–278 CrossRef CAS PubMed.
- S. H. Kang, T. Aoki and G. Kwak, Macromolecules, 2019, 52, 7984–7993 CrossRef CAS.
- Y. Shi and R. A. Weiss, Macromolecules, 2014, 47, 1732–1740 CrossRef CAS.
- T. Y. Yang, M. Wang, F. Jia, X. Y. Ren and G. H. Gao, J. Mater. Chem. C, 2020, 8, 2326–2335 RSC.
- H. Du, Z. Song, J. Wang, Z. Liang, Y. Shen and F. You, Sens. Actuators, A, 2015, 228, 1–8 CrossRef CAS.
- C. Liu, J. Huang, D. Yuan and Y. Chen, Ind. Eng. Chem. Res., 2018, 57, 14527–14534 CrossRef CAS.
- F. H. Zhang, Y. L. Xia, L. L. Wang, L. W. Liu, Y. J. Liu and J. S. Leng, ACS Appl. Mater. Interfaces, 2018, 10, 35526–35532 CrossRef CAS PubMed.
- S. A. Turner, J. Zhou, S. S. Sheiko and V. S. Ashby, ACS Appl. Mater. Interfaces, 2014, 6, 8017–8021 CrossRef CAS.
- C. Li, Y. Jiao, X. Lv, S. Wu and J. Chu, ACS Appl. Mater. Interfaces, 2020, 12, 13464–13472 CrossRef PubMed.
- H. Y. Zhang, H. Lai, Z. J. Cheng, D. J. Zhang, P. C. Liu, Y. F. Li and Y. Y. Liu, Appl. Surf. Sci., 2020, 525, 146525 CrossRef CAS.
- F. Song, Z. Li, P. Jia, M. Zhang, C. Bo, G. Feng, L. Hu and Y. Zhou, J. Mater. Chem. A, 2019, 7, 13400–13410 RSC.
- D. Liu, W. C. Nie, Z. B. Wen, C. J. Fan, W. X. Xiao, L. Bei, X. J. Lin, K. K. Yang and Y. Z. Wang, ACS Macro Lett., 2018, 7, 705–710 CrossRef CAS.
- Y. N. Chen, L. Peng, T. Q. Liu, Y. X. Wang, S. J. Shi and H. L. Wang, ACS Appl. Mater. Interfaces, 2016, 8, 27199–27206 CrossRef CAS PubMed.
- L. Yang, Z. Wang, G. Fei and H. Xia, Macromol. Rapid Commun., 2017, 38, 1700421 CrossRef PubMed.
- W. Peng, G. Zhang, Q. Zhao and T. Xie, Adv. Mater., 2021, 2102473 CrossRef CAS.
- S. Yang, Y. Zhang, T. Wang, W. Sun and Z. Tong, ACS Appl. Mater. Interfaces, 2020, 12, 46701–46709 CrossRef CAS.
- S. Zhang, Q. Ran, Q. Fu and G. Yi, Macromolecules, 2018, 51, 6561–6570 CrossRef CAS.
- H. Y. Lai, H. Q. Wang, J. C. Lai and C. H. Li, Molecules, 2019, 24, 3224 CrossRef CAS PubMed.
- Y. Zhang, M. S. Desai, T. Wang and S. W. Lee, Biomacromolecules, 2020, 21, 1149–1156 CrossRef CAS PubMed.
- Q. Wu, Y. Bai, Y. Chen, J. Ju, F. Zhang and T. Wang, J. Mater. Chem. A, 2015, 3, 352–359 RSC.
- R. J. Wojtecki, M. A. Meador and S. J. Rowan, Nat. Mater., 2011, 10, 14 CrossRef CAS PubMed.
- A. M. Kushner, J. D. Vossler, G. A. Williams and Z. Guan, J. Am. Chem. Soc., 2009, 131, 8766–8768 CrossRef CAS PubMed.
- Z. V. Guan, J. T. Roland, J. Z. Bai, S. X. Ma, T. M. Mcintire and M. Nguyen, J. Am. Chem. Soc., 2004, 126, 2058–2065 CrossRef CAS PubMed.
- Y. Fang, Y. Ni, S. Y. Leo, C. Taylor, V. Basile and P. Jiang, Nat. Commun., 2015, 6, 7416 CrossRef CAS PubMed.
- Y. Fang, Y. Ni, S. Y. Leo, B. Wang, V. Basile, C. Taylor and P. Jiang, ACS Appl. Mater. Interfaces, 2015, 7, 23650–23659 CrossRef CAS.
- H. Koerner, G. Price, N. A. Pearce, M. Alexander and R. A. Vaia, Nat. Mater., 2004, 3, 115–120 CrossRef CAS PubMed.
- L. Yang, Z. Wang, G. Fei and H. Xia, Macromol. Rapid Commun., 2017, 38, 1700421 CrossRef PubMed.
- A. Toncheva, F. Khelifa, Y. Paint, V. Michel, P. Lambert, P. Dubois and J. M. Raquez, ACS Appl. Mater. Interfaces, 2018, 10, 29933–29942 CrossRef CAS PubMed.
- H. N. Wang, L. Fang, Z. Zhang, J. Epaarachchi, L. Li, X. Hu, C. Lu and Z. Xu, Composites, Part A, 2019, 125, 105525 CrossRef CAS.
- S. Wu, W. Li, Y. Q. Sun, X. L. Pang, X. J. Zhang, J. L. Zhuang, H. R. Zhang, C. F. Hu, B. F. Lei and Y. L. Liu, J. Mater. Chem. C, 2020, 8, 8935 RSC.
- L. Amornkitbamrung, S. Srisaard, C. Jubsilp, C. W. Bielawski and S. Rimdusit, Polymer, 2020, 209, 122986 CrossRef CAS.
- P. N. Chang, W. B. Niu, L. C. Qu and S. F. Zhang, J. Mater. Chem. C, 2019, 7, 1896–1903 RSC.
- M. Quan, B. Yang, J. Wang, H. Yu and X. Cao, ACS Appl. Mater. Interfaces, 2018, 10(4), 4243–4249 CrossRef CAS.
- J. Han, G. Fei, G. Li and H. Xia, Macromol. Chem. Phys., 2013, 214, 1195–1203 CrossRef CAS.
- G. Li, G. Fei, H. Xia, J. Han and Y. Zhao, J. Mater. Chem., 2012, 22, 7692–7696 RSC.
- B. Liu, H. Xia, G. Fei, L. Guo and W. Fan, Macromol. Chem. Phys., 2013, 214, 2519–2527 CrossRef CAS.
- M. Bao, Q. Zhou, W. Dong, X. Lou and Y. Zhang, Biomacromolecules, 2013, 14, 1971–1979 CrossRef CAS PubMed.
- Q. Yin, S. Tu, M. Chen and L. Wu, ACS Appl. Polym. Mater., 2020, 2, 2858–2866 CrossRef CAS.
- G. Li, T. Gao, G. Fan, Z. T. Liu and Y. Zhao, ACS Appl. Mater. Interfaces, 2020, 12, 6407–6418 CrossRef CAS PubMed.
- H. Xiao, C. X. Ma, X. X. Le and L. Wang, Polymers, 2017, 9, 138 CrossRef PubMed.
- L. Gu, Y. Z. Jiang and J. L. Hu, Mater. Today: Proc., 2019, 16, 1491–1496 CAS.
- X. Qi, X. Yao, S. Deng, T. N. Zhou and Q. Fu, J. Mater. Chem. A, 2014, 2, 2240–2249 RSC.
- G. H. Yang, X. J. Wan, Y. J. Liu, L. Rui, Y. K. Su, X. R. Zeng and J. N. Tang, ACS Appl. Mater. Interfaces, 2016, 8, 34744–34754 CrossRef CAS PubMed.
- S. S. Song, R. H. Dong, D. Wang and A. X. Song, Soft Matter, 2013, 9, 4209–4218 RSC.
- B. Xu, Y. Y. Zhang and W. G. Liu, Macromol. Rapid Commun., 2015, 36, 1585–1591 CrossRef CAS PubMed.
- Y. Li, H. M. Chen, D. Liu, W. X. Wang, Ye. Liu and S. B. Zhou, ACS Appl. Mater. Interfaces, 2015, 7, 12988–12999 CrossRef CAS.
- X. L. Gong, Y. Y. Xiao, M. Pan, K. Yang, B. J. Li and S. Zhang, ACS Appl. Mater. Interfaces, 2016, 8, 27432–27437 CrossRef CAS.
- Q. J. Song, H. M. Chen, S. B. Zhou, K. Q. Zhao, B. Q. Wang and P. Hu, Polym. Chem., 2016, 7, 1739–1746 RSC.
- X. J. Han, Z. Q. Dong, M. M. Fan, Y. Liu, J. H. Li, Y. F. Wang, Q. J. Yuan, B. J. Li and S. Zhang, Macromol. Rapid Commun., 2012, 33, 1055–1060 CrossRef CAS PubMed.
- J. Huang, J. Liao, T. Wang, W. Sun and Z. Tong, Soft Matter, 2018, 14, 2500–2507 RSC.
- J. X. Liao, J. H. Huang, S. R. Yang, X. L. Wang, T. Wang, W. X. Sun and T. Zhen, ACS Appl. Polym. Mater., 2019, 1, 2703–2712 CrossRef CAS.
- Y. Fang, S. Y. Leo, Y. L. Ni, J. Y. Wang and B. C. Wang, ACS Appl. Mater. Interfaces, 2017, 9, 5457–5467 CrossRef CAS PubMed.
- B. T. Michal, E. J. Spencer and S. J. Rowan, ACS Appl. Mater. Interfaces, 2016, 8, 11041–11049 CrossRef CAS.
- G. Zhang, Q. Zhao, L. Yang, W. Zou, X. Xi and T. Xie, ACS Macro Lett., 2016, 5, 805–808 CrossRef CAS.
- M. Burnworth, L. Tang, J. R. Kumpfer, A. J. Duncan, F. L. Beyer, G. L. Fiore, S. J. Rowan and C. Weder, Nature, 2011, 472, 334–337 CrossRef CAS PubMed.
- D. Habault, H. Zhang and Y. Zhao, Chem. Soc. Rev., 2013, 42, 7244–7256 RSC.
- W. Feng, W. F. Zhou, S. D. Zhang, Y. J. Fan, Y. Akram and H. Y. Yang, RSC Adv., 2015, 5, 81784–81789 RSC.
- Y. K. Bai, J. W. Zhang, D. D. Wen and B. Yuan, J. Mater. Chem. A, 2019, 7, 20723–20732 RSC.
- S. Kobatake, S. Takami, H. Muto, T. Ishikawa and M. Irie, Nature, 2007, 446, 778–781 CrossRef CAS PubMed.
- Y. Zheng, M. Micic, S. V. Mello, M. Mabrouki, F. M. Andreopoulos, V. Konka, S. M. Pham and R. M. Leblanc, Macromolecules, 2002, 35, 5228–5234 CrossRef CAS.
- L. Wang, X. F. Yang, H. M. Chen, T. Gong, W. B. Li, G. Yang and S. B. Zhou, ACS Appl. Mater. Interfaces, 2013, 5, 10520–10528 CrossRef CAS.
- H. N. Van and P. F. E. Du, Macromolecules, 2018, 51, 3405–3414 CrossRef.
- H. Y. Jiang, S. Kelch and A. Lendlein, Adv. Mater., 2006, 18, 1471–1475 CrossRef CAS.
- G. Stoychev, A. Kirillova and L. Ionov, Adv. Opt. Mater., 2019, 7, 1900067 CrossRef.
- J. Bai and Z. Shi, ACS Appl. Mater. Interfaces, 2017, 9, 27213–27222 CrossRef CAS.
- S. Banerjee, R. Tripathy, D. Cozzens, T. Nagy, S. Keki, M. Zsuga and R. Faust, ACS Appl. Mater. Interfaces, 2015, 7, 2064–2072 CrossRef CAS.
- H. Xie, M. J. He, X. Y. Deng, L. Du, C. J. Fan, K. K. Yang and Y. Z. Wang, ACS Appl. Mater. Interfaces, 2016, 8, 9431–9439 CrossRef CAS PubMed.
- T. Defize, J. M. Thomassin, H. Ottevaere, C. Malherbe, G. Eppe, R. Jellali, M. Alexandre, C. Jérôme and R. Raphaël, Macromolecules, 2018, 52, 444–456 CrossRef.
- C. Huang, J. Lv, X. Tian, Y. Wang, Y. Yu and L. Jie, Sci. Rep., 2015, 5, 17414 CrossRef CAS PubMed.
- X. Zhang, C. Y. Zhu, B. Xu, Q. Lang, L. Qin, J. Wei and Y. L. Yu, ACS Appl. Mater. Interfaces, 2019, 11, 46212–46218 CrossRef CAS.
- M. Pan, Q. Yuan, X. L. Gong, S. Zhang and B. J. Li, Macromol. Rapid Commun., 2016, 37, 433–438 CrossRef CAS PubMed.
- X. Q. Li, Z. H. Wang and H. S. Xia, Front. Chem., 2019, 7, 59 CrossRef CAS.
- X. Lu, G. Fei, H. Xia and Y. Zhao, J. Mater. Chem. A, 2014, 2, 16051–16060 RSC.
- J. M. J. Paulusse and R. P. Sijbesma, Angew. Chem., Int. Ed., 2004, 43, 4460–4462 CrossRef CAS.
- J. M. J. Paulusse, J. P. J. Huijbers and R. P. Sijbesma, Chem.–Eur. J., 2006, 12, 4928–4934 CrossRef CAS.
- H. Xiao, L. Wei, L. X. Xia, C. X. Ma, W. L. Zhao, J. Zheng, J. W. Zhang, Y. J. Huang and T. Chen, Chem. Commun., 2016, 52, 13292–13295 RSC.
- J. Xu, Y. He, Y. T. Li and Q. S. Ye, Mater. Lett., 2020, 260, 126968 CrossRef.
- W. Nan, W. Wang, H. Gao, W. Liu and W. Wang, Soft Matter, 2013, 9, 132–137 RSC.
- M. Xie, C. Wu, C. Chen, Y. Liu and C. Zhao, Polym. Chem., 2019, 10, 4852–4858 RSC.
- P. F. Zhang, M. Behl, X. Z. Peng, M. Balk and A. Lendlein, Chem. Mater., 2019, 31, 5402–5407 CrossRef CAS.
- N. Li, G. Chen, W. Chen, J. Huang, J. Tian, X. Wan, M. He and H. Zhang, Carbohydr. Polym., 2017, 178, 159–165 CrossRef CAS.
- H. Meng, J. Zheng, X. Wen, Z. Cai, J. Zhang and T. Chen, Macromol. Rapid Commun., 2015, 36, 533–537 CrossRef CAS PubMed.
- X. Le, W. Lu, H. Xiao, L. Wang, C. Ma, J. Zhang, Y. Huang and T. Chen, ACS Appl. Mater. Interfaces, 2017, 9, 9038–9044 CrossRef CAS PubMed.
- Y. Xu, G. G. Lu, M. Y. Chen, P. L. Wang, Z. L. Li, X. W. Han, J. Liang, Y. Sun, Y. J. Fan and X. D. Zhang, Carbohydr. Polym., 2020, 250, 116979 CrossRef CAS.
- M. Wang, J. P. Zhuge, C. Q. Li, L. B. Jiang and H. Yang, Iran. Polym. J., 2020, 29, 569–579 CrossRef.
- M. Wang, B. Mo, B. Chen, L. Jiang and H. Yang, Colloid Polym. Sci., 2020, 298, 285–291 CrossRef CAS.
- Z. Li, G. Davidson-Rozenfeld, M. Vázquez-González, M. Fadeev, M. Zhang, H. Tian and I. Willner, J. Am. Chem. Soc., 2018, 140, 17691–17701 CrossRef CAS PubMed.
- L. Y. Tang, L. F. Wen, S. P. Xu, P. H. Pi and X. F. Wen, Chem. Commun., 2018, 54, 8084–8087 RSC.
- S. Wang, G. Li, Z. W. Liu and Y. Zhao, ACS Appl. Mater. Interfaces, 2019, 11, 30308–30316 CrossRef CAS.
- Y. Cong, S. Liu, F. Wu, H. Zhang and J. Fu, J. Mater. Chem. B, 2020, 8, 9679–9685 RSC.
|
This journal is © The Royal Society of Chemistry 2021 |