Rotation-induced recovery and bleaching in magnetic resonance
Received
9th December 2014
, Accepted 26th January 2015
First published on 6th February 2015
Abstract
Thurber and Tycko recently described a ‘bleaching effect’ that occurs in magnetic resonance when solid samples that are doped with paramagnetic agents are subjected to rotation by magic angle spinning (MAS) in a static magnetic field with a rotation period comparable to the longitudinal relaxation time T1e of the electron spins. The bleaching effect has been investigated by Thurber and Tycko in samples spinning at temperatures near 20 K in a field of 9.4 T and by Corzilius et al. near 80 K in a field of 4.9 T. In our experience, the bleaching effect is not very severe at temperatures near 100 K in a field of 9.4 T at spinning frequencies up to 12 kHz. Bleaching can partly cancel DNP enhancements that are normally evaluated by comparing signal intensities with and without microwave irradiation. The signal attenuation due to doping and sample rotation is usually not taken into consideration when defining enhancement factors. In this paper, we describe a novel observation that the rotation of glassy samples doped with lanthanides spinning at frequencies as low as 0.1 kHz can lead to a significant reduction of the spin–lattice relaxation times T1(1H) of protons. This effect, which bears similarities with the so-called spin refrigerators, may contribute to the success of ‘brute force polarization’ at sample temperatures in the mK range. The acceleration of longitudinal proton relaxation also allows one to improve the signal-to-noise ratio per unit time.
Introduction
Dynamic nuclear polarization (DNP) in solid samples relies on a transfer of polarization from electron spins to nuclear spins induced by irradiation of electron paramagnetic resonance (EPR) transitions. This allows one to boost the sensitivity of nuclear magnetic resonance (NMR). The effect was predicted by Overhauser1 and confirmed by Carver and Slichter in 1953.2 Depending on the number of electron and nuclear spins involved in this process, different DNP mechanisms may be effective. In a system comprising a single electron spin and a single nuclear spin, the mechanism is known as the ‘solid effect’.3 In the presence of two electron spins and a single nuclear spin, a ‘cross effect’ may occur.4–6 For larger numbers of spins, a ‘thermal mixing’ process should be considered.3,7–9 Other methods can also produce large enhancements of nuclear polarizations, like optical pumping and proton spin refrigeration.10 DNP experienced a renaissance in the 90's11,12 with the advent of gyrotrons combined with magic angle spinning (MAS), which can multiply the sensitivity by several orders of magnitude in glassy samples doped with paramagnetic agents. Renewed interest in low temperature DNP was triggered by the invention of dissolution DNP.13 This method also generated interest for ‘brute force polarization’ where the sample is cooled to mK temperatures to enhance the Zeeman polarization, without resorting to any microwave irradiation.14–16
Recently, a decrease of nuclear polarization due to the rotation of glassy samples doped with paramagnetic impurities has been observed17 and evaluated for various radicals at 4.9 T and 80 K (ref. 18) and at 9.4 T and 18 K.19 This decrease tends to cancel some of the benefits of DNP. The enhancement factors have to be carefully measured to evaluate the balance between the positive and negative contributions to the nuclear polarization that can arise from doping with different paramagnetic agents such as nitroxides and lanthanides, microwave irradiation, sample spinning, low temperatures, and high magnetic fields.
We shall briefly discuss mechanisms that can drive the nuclear polarization away from thermal equilibrium through mechanical rotation of samples doped with paramagnetic agents. The use of the lanthanide Dy3+ as a doping agent in either static or rotating glassy samples leads to a welcome reduction of the longitudinal relaxation times T1(1H) in solids.14,19 An NMR study of samples doped with Dy3+ spinning at low temperature should consider the possibility of a proton spin refrigerator scenario.20–25 We shall explore some properties of Dy3+ in spinning glassy samples that may have implications for DNP-MAS and possibly for ‘brute force’ polarization at very low sample temperatures.
Proton spin refrigerator
A crystal of yttrium ethyl sulfate doped with ytterbium, Yb:Y(C2H5SO4)3·9H2O, also known as Yb:YES was made to rotate around an axis perpendicular to a static magnetic field B0 = 1 T at a temperature near 1 K with a spinning frequency of a few tens of Hz.20–22 Rotation frequencies up to a few kHz were reported later.23 Upon spinning the sample at 60 Hz, the proton polarization could be boosted to P(1H) = 19%.20 This effect could be rationalized by the interplay between the anisotropy of the electron g-factor, the electron relaxation time T1e and the proton spin relaxation time T1(1H). The lowest pair of energy levels in the Yb3+ EPR manifold can be described in terms of a fictitious spin I = 1/2 with an anisotropic g-factor given by: |  | (1) |
where g∥2 is parallel to the c axis of the crystal while g⊥2 is perpendicular to this axis, and θ is the angle subtended between the c axis and the magnetic field.
Static samples
A strong intensity variation of the EPR response as a function of the angle θ was observed near 1.5 K.24 At θ = 45°, T1e reaches a minimum, thus allowing the electron polarization to reach the Boltzmann equilibrium in a few milliseconds. By changing the angle θ by 45°, T1e changes by more than two orders of magnitude and can be as long as 1 s. At the same time, the electron splitting matches the nuclear Zeeman splitting, thus allowing cross relaxation and polarization transfer from the electron spins to the nuclear spins.20
Spinning samples
Continuous rotation leads to a periodical repetition of this process, leading to a large nuclear polarization without any need for microwave irradiation, in contrast to DNP experiments. This process is presented in Fig. 1, and can occur in powdered samples at angles 0° < φ < 90° where φ is the angle between the magnetic field and the rotation axis.20 The refrigerator requires paramagnetic agents such as Yb3+, Ce3+ or Dy3+. The nuclear polarization is transferred by spin diffusion from protons that are close to the paramagnetic center to more distant protons.20,25 When spinning about the magic angle, it has been demonstrated by simulations that proton spin diffusion is efficient at spinning frequencies of a few kHz, but becomes less efficient at higher speeds.26
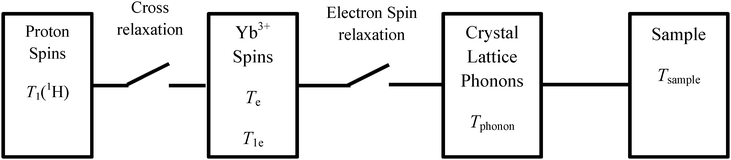 |
| Fig. 1 Scheme showing switches involved in spin refrigerators.20 At a given orientation, the EPR splitting is maximum while the electron relaxation time T1e is minimum. The electron spin bath has a temperature Te and relaxes towards thermal equilibrium while releasing heat to the phonon bath which has a temperature Tphonon, ultimately transferring the heat to the helium bath that has a temperature Tsample. After tilting the sample through an angle of 45°, the electron splitting becomes comparable to the proton Zeeman splitting, thus favoring cross relaxation. In this step the electron bath is warmed up by the proton spin bath that is therefore cooled down. Continuous sample spinning leads to a periodic repetition of the process. | |
Variants of the concept of proton spin refrigerators were suggested based on variations of temperature, light or pressure instead of the angle θ. The proton spin refrigerator can also work upon changing the magnetic field, or by inducing level crossings.20 Different conditions on the splittings were discussed.25 Recent simulations by Thurber and Tycko demonstrated that similar effects can occur when level crossings involve three spins19 in glassy samples.
Nuclear proton polarizations as large as P(1H) = 35% at 1.3 K and 2 T could be achieved with Yb3+, with enhancements of ε ≈ 220 with respect to thermal equilibrium under static conditions.23 Other doped crystals like Dy:YES were also shown to transfer a significant fraction of electron polarization to proton polarizations at 0.5 K and 0.12 T with a pulsed field of 0.04 T to polarize the electrons.25 Here, a relationship between the refrigerator cycle period and the proton polarization build up time is shown.
Right-Angle Spinning Electron Paramagnetic Resonance (RAS-EPR) involves spinning samples around an axis perpendicular with respect to the static magnetic field.27–29 This method allows one to shorten EPR acquisition times and resolve anisotropic interactions.27,29
Static bleaching
It is well known that local magnetic fields produced by paramagnetic agents may shift the nuclear resonance frequencies of nearby nuclear spins.3,30–32 This translates into line broadening and hence sensitivity losses in doped glassy static (i.e., non-spinning) samples.19 In this work, we shall refer to this effect as “static bleaching”. We may define a bleaching factor εstatbleach(1H) ≤ 1 as the ratio between the areas of proton spectra of doped and un-doped static samples, assuming that the proton polarization has reached thermal equilibrium in both cases.
Spinning-induced bleaching
Recent observations by Thurber and Tycko in DNP-MAS studies demonstrated a significant decrease of the proton polarization P(1H) with respect to thermal equilibrium in rotating glassy samples doped with paramagnetic agents as are commonly used for DNP at low temperatures.17,19 We shall refer to this effect as “spinning-induced bleaching” to distinguish it from “static bleaching”. We introduce here the bleaching factor εspinbleach(1H) as the ratio between the area of a spectrum measured with a spinning sample and the area measured with a static sample. An extensive experimental evaluation18 at 4.9 T and temperatures around 80 K demonstrated significant losses of proton polarization P(1H) in samples spinning at νrot = 4.975 kHz with respect to non-doped samples measured indirectly with cross polarization, with εspinbleach = 0.4 when using Ox063 (Trityl) and εspinbleach = 0.5 for TOTAPOL.18
Simulations involving a system of two electron spins and one nuclear spin during MAS in the absence of microwave irradiation demonstrate the importance of the cross effect for spinning-induced bleaching.19,33 Experiments have shown that the effect decreases the area of a proton spectrum of a static sample doped with TOTAPOL or DOTOPA recorded at thermal equilibrium at 20 K by 5 to 6 times when spinning at 6 kHz at the same temperature.19 The model used to explain the bleaching effect also predicted an increase of nuclear polarization P(1H) under certain conditions, in particular when the nuclear Larmor frequencies are smaller than the anisotropy of the electron g-factor.19
Increase of sample temperature due to sample rotation
It is well known that the friction between the rotors and the flowing nitrogen gas increases the sample temperature, as can be measured using suitable NMR thermometers.34–37 To determine the DNP enhancement as a function of the spinning speed, care must be taken to monitor and control the temperature.38
Another factor that may be responsible for increasing sample temperatures has been reported in rotating samples that are doped with paramagnetic agents, especially when these paramagnetic agents exhibit large g-factor anisotropies like those used for spin refrigerators. This heating effect was explained by the evacuation of phonons from the proton spin bath into the sample via the electron spin bath. Non-resonant heating by relaxation depends on the concentration of paramagnetic agents and the sample temperature, among other factors. It was measured to be between 1 and 1000 mW g−1 in Yb:YES crystals when the sample was spinning about an axis perpendicular to the magnetic field.20,39
Because these effects alter the DNP enhancements, their evaluation is necessary. In this paper we shall focus on the effects of spinning of samples doped with nitroxides, lanthanides and mixtures of nitroxides and lanthanides on the longitudinal proton relaxation times T1(1H) and proton polarization P(1H).
Experimental
The experiments were carried out using a Bruker 9.4 T spectrometer with 3.2 mm diameter rotors spinning at frequencies up to 12 kHz at nominal temperatures near 100 K. The triple-resonance probe was coupled to a gyrotron that can provide 5 W microwave irradiation at 263 GHz. The rotors were made of either thin-walled zirconia or sapphire. Proton saturation recovery experiments conducted in static or spinning samples used saturation by trains of 100 pulses with 90° angles spaced by 1 ms followed by recovery intervals between 1 and 10 s. To determine the proton build-up times τDNP(1H), 10° pulses were used. The measurements without microwave irradiation, using either un-doped samples or samples doped with lanthanides, employed 45° or 90° pulses. The build-up and recovery curves were fitted to mono-exponential functions to determine τDNP(1H) and T1(1H). Cross polarization from 1H to 13C was performed with or without microwave irradiation. DyCl3 and HoCl3 salts were bought from Sigma Aldrich. Fresh samples were prepared less than a day before the measurements.
Three kinds of experimental data were observed in un-doped samples and in samples doped with nitroxides (TEMPOL and TOTAPOL), lanthanides (DyCl3 and HoCl3) or mixtures of TEMPOL and DyCl3. The relaxation time T1(1H) was measured in static and spinning samples. The thermal equilibrium proton polarization P(1H) was measured in static and spinning samples with a single pulse to evaluate the extent of spinning-induced bleaching. The nuclear DNP enhancement εDNP was measured in glycerol
:
H2O 1
:
1 (v
:
v) samples doped with 50 mM TEMPOL with or without addition of 10 mM DyCl3. Mixtures of different paramagnetic agents have been used for DNP at low temperatures.40–43 To evaluate the sensitivity per unit time it is necessary to account for the overall time required for the experiments. The following ratio κspin can be defined for a doped sample:
κspin = T1(1H)static/T1(1H)spinning |
To evaluate the overall enhancement it is necessary to account for the build-up time by defining the ratio
κDNP:
κ
DNP = Tun-doped1(1H)/τDNP(1H) |
T
un-doped1(
1H) being the longitudinal relaxation time of the un-doped sample, in analogy to previous studies.
18,44 Note that our factor
κDNP is related to the factor
κ0 defined by Corzilius
et al.18 since
κ0 = (
κDNP)
1/2. A factor
εTot can be defined as
εTot= εDNP ×
εspinbleach ×
εstatbleach × (
κDNP)
1/2. Thus the overall effect is assumed to be given by the product of static and spinning-induced bleaching factors.
Evaluation of T1(1H) in samples doped with paramagnetic agents
Longitudinal proton relaxation times T1(1H) were measured in static and spinning samples of glycerol:H2O doped with either DyCl3 or HoCl3. These paramagnetic agents have been used as relaxation agents in static14,15,19 and spinning19 glassy samples. Solvent mixtures with glycerol
:
H2O 60
:
40 (v
:
v) or glycerol
:
H2O 50
:
50 (v
:
v) were used without deuteration (see Fig. 2). One does not expect a pronounced orientation dependence of T1(1H) in glassy static samples, unlike what has been observed in crystals.20 A strong anisotropic g-factor has been reported in a sample of 20 mM Dy(III)DOTA in glycerol
:
H2O 1
:
1 (v
:
v).45 In samples of glycerol
:
H2O 60
:
40 (v
:
v) doped with different concentrations of DyCl3, slow spinning about the magic angle at 10 < νrot < 100 Hz leads to a shortening of T1(1H) by nearly a factor of two compared to static samples (Fig. 2). A decrease of T1(1H) with an increase in the lanthanide concentration can also be observed in static samples in Fig. 2a.14 A less dramatic shortening of T1(1H) was observed with an increase in the spinning frequency νrot in an un-doped sample of glycerol
:
H2O 1
:
1 (v
:
v) (Fig. 2b), showing a minimum near νrot = 2 kHz. The appearance of such a minimum was common to all glassy samples studied in this work, as shown in Fig. 2. However, the reduction of T1(1H) was not as pronounced as for lanthanides. Fig. 2c shows a comparison of T1(1H) measured in static and spinning samples of glycerol
:
H2O 1
:
1 (v
:
v) doped with 20 mM DyCl3 and 20 mM HoCl3. In Fig. 2(a) and (c), T1(1H) first drops sharply near νrot = 100 Hz and then continues to decrease slowly to a minimum near νrot = 2 kHz. The shortening of T1(1H) with an increase in νrot in the absence of paramagnetic agents, shown in Fig. 2b, can be ascribed to the acceleration of spin diffusion with an increase of νrot.26 Small amounts of paramagnetic molecular oxygen may also play a role. For spinning frequencies νrot > 2 kHz, T1(1H) tends to increase slightly, as in Fig. 2c. Indeed, high spinning frequencies νrot lead to inefficient spin diffusion.26
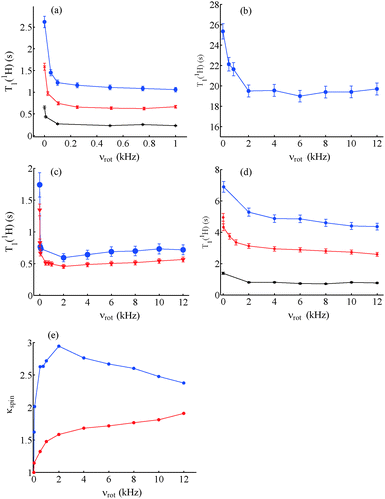 |
| Fig. 2 (a) Relaxation times T1(1H) in samples of glycerol : H2O 60 : 40 (v : v) doped with 10 mM DyCl3 (blue), 20 mM DyCl3 (red) and 40 mM DyCl3 (black) as a function of slow spinning frequencies 0 < νrot < 1 kHz. (b) T1(1H) relaxation times in samples of un-doped glycerol : H2O 1 : 1 (v : v) at spinning frequencies 0 < νrot < 12 kHz. (c) Relaxation times T1(1H) in samples of glycerol : H2O 1 : 1 (v : v) doped with 20 mM DyCl3 (red) and 20 mM HoCl3 (blue) at spinning frequencies 0 < νrot < 12 kHz. (d) Relaxation times T1(1H) in samples of glycerol : H2O 1 : 1 (v : v) doped with 25 mM TOTAPOL (blue), 50 mM TEMPOL (red) and a mixture of 50 mM TEMPOL and 10 mM DyCl3 (black) at spinning frequencies 0 < νrot < 12 kHz. (e) Acceleration of the proton spin–lattice relaxation described by the factor κspin = T1(1H)static/T1(1H)spinning for samples of glycerol : H2O 1 : 1 (v : v) doped with 20 mM DyCl3 (blue) and 50 mM TEMPOL (red). All data were recorded at 9.4 T at a nominal temperature of 100 K. The areas of the proton spectra were integrated after saturation recovery at thermal equilibrium for 5 samples without microwave irradiation. | |
Fig. 2d shows T1(1H) as a function of the spinning frequency νrot for samples of glycerol
:
H2O 1
:
1 (v
:
v) doped with 50 mM TEMPOL, 25 mM TOTAPOL, 50 mM TEMPOL and 10 mM DyCl3. Although there is an initial decrease in T1(1H) for samples doped only with nitroxides, this decrease amounts to merely 10% of T1(1H) at νrot = 100 Hz, similar to un-doped samples.
Fig. 2e shows κspin for samples of glycerol
:
H2O 1
:
1 (v
:
v) doped either with 50 mM TEMPOL or 20 mM DyCl3. The trend in samples doped with Dy3+ is for κspin to increase sharply until νrot = 2 kHz and then to decrease, whereas for samples doped with TEMPOL there is little effect until νrot = 2 kHz followed by an increase at higher spinning frequencies.
Bleaching in static samples was evaluated by proton saturation recovery experiments at 9.4 T and 100 K for glycerol
:
H2O 1
:
1 (v
:
v) doped with 50 mM TEMPOL with or without 10 mM DyCl3. The static bleaching factors were εstatbleach = 0.83 and 0.89 for samples doped with TEMPOL with and without DyCl3 respectively. In the un-doped sample, εstatbleach = 1 (Fig. 3a). Considering a model of effective bleached spheres, where each paramagnetic agent influences a spherical volume with a radius r, we determined that r < 10 Å for TEMPOL and r < 13 Å for DyCl3. Our estimates did not take line broadening into account, in agreement with ref. 33. Measurements on samples that are un-doped or doped with DyCl3 only showed smaller bleached spheres, with an effective r = 9 Å (data not shown). An effective bleached sphere for Yb3+ with r = 7 Å was suggested in Yb:YES.20 In any case the existence of paramagnetic impurities in glassy matrices leads to small sensitivity losses.18,46 On the other hand the presence of paramagnetic agents leads to a shortening of T1(1H), which may boost the sensitivity per unit time.
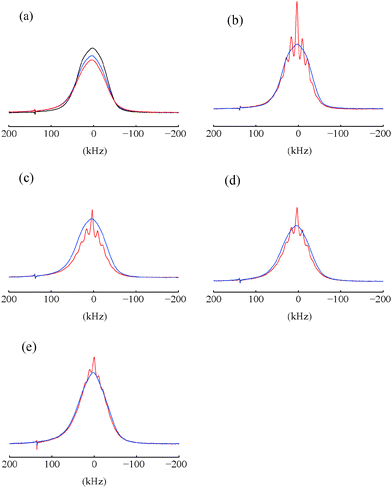 |
| Fig. 3 (a) Proton spectra at thermal equilibrium of a static un-doped sample of glycerol : H2O 1 : 1 (v : v) (black), of a similar sample doped with 50 mM TEMPOL (blue) and of a similar sample doped with 50 mM TEMPOL and 10 mM DyCl3 (red). (b) Proton spectra of a static un-doped sample of glycerol : H2O 1 : 1 (v : v) (blue) and of the same sample spinning at 12 kHz (red). (c) Proton spectra of a static sample of glycerol : H2O doped with 50 mM TEMPOL (blue) and of the same sample spinning at 12 kHz (red). (d) Proton spectra of a static sample of glycerol : H2O doped with 50 mM TEMPOL and 10 mM DyCl3 (blue) and of the same sample spinning at 12 kHz (red). (e) Proton spectra of a static sample of glycerol : H2O doped with 10 mM DyCl3 (blue) and of the same sample spinning at 12 kHz (red). All data were taken at 9.4 T at a nominal temperature of 100 K. | |
Bleaching in spinning samples was determined by comparing the areas of the proton spectra of static and spinning samples at thermal equilibrium. A thin-walled zirconia rotor was used for these measurements. The spectra of static samples and samples spinning at νrot = 12 kHz recorded using un-doped samples of glycerol
:
H2O 1
:
1 (v
:
v) and samples doped with either 50 mM TEMPOL alone or with 50 mM TEMPOL plus 10 mM DyCl3 are shown in Fig. 3. A 20% decrease of the signal area occurs when spinning the sample doped only with 50 mM TEMPOL at νrot = 12 kHz (Fig. 3c) compared to the static sample. Although significant, this decrease is not as important as was found at lower temperatures.18,19 Adding 10 mM DyCl3 enhances static bleaching as shown in Fig. 3a, but reduces spinning-induced bleaching in comparison with samples doped with TEMPOL only. In the case of un-doped samples (Fig. 3b), the areas of the proton spectra show a small decrease at νrot = 12 kHz compared to the static spectra. In this sample the spinning-induced bleaching is not as significant as in samples doped only with nitroxides (Fig. 3c). Another example in Fig. 3e shows two proton spectra of glycerol
:
H2O 1
:
1 (v
:
v) doped with 20 mM DyCl3 at thermal equilibrium and νrot = 0 or 10 kHz. The lack of spinning-induced bleaching is clearly seen. Samples doped with 20 mM HoCl3 did not show any significant bleaching.
The behavior of bleaching at different spinning frequencies can be seen in Fig. 4a. In samples doped with nitroxides, spinning-induced bleaching reduces the area of the proton spectra when the spinning speed is increased. For a sample of glycerol
:
H2O 1
:
1 (v
:
v) with 50 mM TEMPOL, the bleaching factor is εspinbleach = 0.8 for νrot = 12 kHz (see also Fig. 3c). By adding 10 mM DyCl3, εspinbleach increases to 0.9 at νrot = 12 kHz (Fig. 3d). It is known that the presence of DyCl3 combined with TEMPOL may shorten the latter's longitudinal electron relaxation time T1e.40–42,45 In samples doped with both agents, spinning-induced bleaching is reduced. The factor is εstatbleach ≈ 1 for 1
:
1 glycerol
:
H2O (v
:
v) doped with 20 mM DyCl3. If we disregard the measurement at 12 kHz, it is also the same for the un-doped sample.
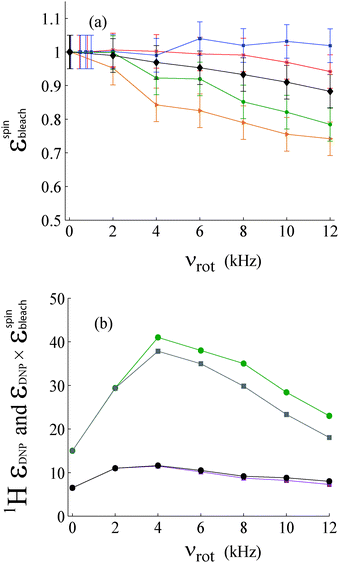 |
| Fig. 4 (a) Bleaching factor εspinbleach due to spinning measured for five samples of glycerol : H2O 1 : 1 (v : v) doped with 20 mM DyCl3 (blue), 50 mM TEMPOL (green), 25 mM TOTAPOL (orange), a mixture of 50 mM TEMPOL and 10 mM DyCl3 (black) and without any doping (red). (b) DNP proton polarization enhancements measured in two samples of glycerol : H2O 1 : 1 (v : v) doped with 50 mM TEMPOL (green), after correction for bleaching due to spinning (grey), for a mixture of 50 mM TEMPOL and 10 mM DyCl3 (black) and after correction for bleaching due to spinning (purple). All data were taken at 9.4 T and a nominal temperature of 100 K. The lines are drawn to guide the eye. | |
A sample of 1
:
1 glycerol
:
H2O (v
:
v) doped with 25 mM TOTAPOL showed a more pronounced spinning-induced bleaching, since εspinbleach = 0.74 at 12 kHz. This is consistent with the more effective cross effect in biradicals, as shown by simulations.19
Samples doped with nitroxides tend to show a decrease in the signal intensity when the spinning speed is increased. The intensity decreases almost linearly with an increase in the spinning frequency at 100 K and 9.4 T. It is shown in Fig. 3 and 4 that this effect is not as pronounced as that observed near 20 K, with εspinbleach = 0.1 to 0.2.19
Dynamic nuclear polarization enhancements were measured in a sample of glycerol
:
H2O 1
:
1 (v
:
v) doped with 50 mM TEMPOL. T1(1H) and build-up times τDNP(1H) were measured by saturation recovery. Enhancements were measured by cross polarization from 1H to 13C, using rf fields of 125 kHz for 1H during the 90° pulse and 78 kHz during the contact time. Spinal-64 proton decoupling was used with an rf field of 78 kHz. Proton DNP build-up times were measured to be τDNP(1H) = 2.5 ± 0.2 s for the sample with 50 mM TEMPOL and τDNP(1H) = 0.55 ± 0.2 s for a sample with 50 mM TEMPOL plus 10 mM DyCl3 for 2 < νrot < 12 kHz. An increased enhancement was observed with an increase in the spinning speed until a maximum around νrot = 4 kHz. Beyond this point the enhancement decreases as shown experimentally and explained theoretically.48,49
A straightforward correction of the proton enhancement is shown in Fig. 4b for both samples by multiplying the enhancement by their respective values of εspinbleach. In the case of the sample doped with TEMPOL only, there is an overall loss of about 20% when spinning at 12 kHz. The reduction of the enhancement with an increase in the spinning frequency is more pronounced than for the case of the doubly-doped sample containing both TEMPOL and DyCl3 where the enhancement was less than in the sample containing only TEMPOL as the doping agent, presumably because of a leakage of polarization.3,47
According to data in Fig. 2–4 for samples doped with TEMPOL with or without DyCl3 at νrot = 4 kHz, the factors are εTOT = 55 and 92, respectively. The factors εTOT are 37 and 45 at νrot = 12 kHz for samples with or without DyCl3, respectively. This suggests that DyCl3 could be used to enhance DNP at higher spinning speeds, albeit at the expense of slightly greater linewidths.
Discussion
The marked reduction of T1(1H) in samples doped with lanthanides such as Dy3+ or Ho3+ at spinning frequencies as low as νrot = 0.1 kHz and in the absence of spinning-induced bleaching is consistent with the principles of the proton spin refrigerator discussed above for lanthanides such as Yb3+, Ce3+
(ref. 20) or Dy3+
(ref. 25) and also at low sample spinning frequencies. These principles are also valid for powdered samples that are spinning about angles that are not orthogonal to the static field.20,23 Simulations by Thurber and Tycko confirm the possibility of effects similar to those of spin refrigerators in MAS-NMR of glassy samples,19 but other lanthanides like Dy3+ were not specifically addressed.
A reduction of T1(1H) was observed in all samples, with a minimum near νrot = 2 kHz. This feature is consistent with the common observation that proton spin diffusion is facilitated at low spinning frequencies, as confirmed by simulations.26 However, the reduction of T1(1H) at low spinning frequencies is not as pronounced when using TEMPOL or TOTAPOL only rather than lanthanides, which lead to a minimum at frequencies as low as νrot = 0.1 kHz.
Despite the sharp reduction of T1(1H) when the sample is spinning at a few tens of Hz, the experimental conditions for the proton spin refrigerator to increase the proton polarization are not completely fulfilled, since the rotor period is not short in comparison with T1e. For Dy3+ at 100 K, T1e is expected to be much shorter than the rotor period τrot = 83.3 μs when spinning at νrot = 12 kHz. A discussion about different Zeeman splittings, along with a relationship between T1(1H) and the rotor period is provided.25 Data for samples of water and glycerol 1
:
1 (v
:
v) doped with 20 mM DyCl3 showed a clear absence of bleaching due to spinning at νrot = 10 or 12 kHz, whereas for un-doped samples a small signal reduction was observed. No bleaching due to spinning was expected for either of these samples.
The reduction of T1(1H) observed in glassy samples doped with lanthanides when spinning at low frequencies may provide an alternative strategy for nuclear polarization relaxation experiments at much lower temperatures, as used for “brute force polarization” in glassy samples doped with paramagnetic agents. This could be exploited by either spinning of the sample or the magnetic field.20,23
The fact that the NMR signal area decreases in samples doped with nitroxides like TEMPOL or TOTAPOL has been explained qualitatively. Simulations show that the interactions between two electron spins and one nuclear spin that can lead to the so-called ‘cross effect’ can be responsible not only for DNP enhancements but also for signal losses.19,48–50 However, the decrease of the NMR response may involve mechanisms other than the cross effect, since a homogeneous saturation of the EPR response (as occurs when increasing the temperature) may be equivalent to irradiation near the center of gravity of the EPR response, thus producing simultaneous zero- and double-quantum transitions, leading to a nuclear polarization that is smaller than that at thermal equilibrium. RAS-EPR could play an important role in determining contributions of different mechanisms to the perturbation of the nuclear magnetic polarization in samples doped with paramagnetic agents that are subject to rotation. In this work we used high concentrations of paramagnetic nitroxides (either TOTAPOL or TEMPOL) to observe spinning-induced bleaching. The use of cross-polarization to evaluate the bleaching effect provides an indirect way to determine the losses of proton polarization.18,19 However, the efficiency of cross polarization may vary from sample to sample and may cause further losses that are not necessarily due to bleaching induced by spinning.
Conclusions
We have evaluated proton NMR signal intensities and longitudinal proton relaxation times T1(1H) of glassy samples doped with paramagnetic agents spinning at the magic angle near 100 K at 9.4 T. The bleaching of the proton polarization due to spinning at 12 kHz leads to a loss of 25% in samples doped with 25 mM TOTAPOL and a loss of 20% in samples doped with 50 mM TEMPOL. These polarization losses are less severe than those measured by Corzilius et al.,18 in agreement with predictions of Thurber and Tycko19 that the bleaching effect should be less severe at higher temperatures and lower concentrations of paramagnetic agents.18 By adding DyCl3 to samples doped with nitroxide radicals it was possible to reduce spinning-induced bleaching, at the expense of some static bleaching and some line broadening. This strategy could be useful at higher spinning speeds and lower temperatures. At relatively high concentrations of nitroxides, we observe a significant decrease of the proton polarization at spinning speeds near 12 kHz, with an almost linear dependence on the spinning frequency. Compared to static samples, a significant reduction of T1(1H) has been observed in glassy samples doped with Dy3+ and Ho3+ spinning at frequencies as low as 0.1 kHz. The reduction of T1(1H) in these glassy samples, which is reminiscent of proton spin refrigerators that use similar paramagnetic agents in crystalline samples,25 allows one to decrease the recovery delays between subsequent experiments and hence obtain a better signal-to-noise ratio per unit time.
Acknowledgements
AJPL would like to thank Professor T. Wenckebach for constructive conversations. This work was supported by the Swiss National Science Foundation (SNSF), the Ecole Polytechnique Fédérale de Lausanne (EPFL), the Swiss Commission for Technology and Innovation (CTI), Bruker Biospin, the French CNRS, and the European Research Council (ERC Advanced Grant 339754 “Dilute Para-Water”).
References
- A. Overhauser, Phys. Rev., 1953, 92, 411 CrossRef CAS.
- T. R. Carver and C. P. Slichter, Phys. Rev., 1953, 92, 212 CrossRef CAS.
- A. Abragam and M. Goldman, Rep. Prog. Phys., 1978, 41, 395 CrossRef CAS.
- K. N. Hu, G. T. Debelouchina, A. A. Smith and R. Griffin, J. Chem. Phys., 2011, 134, 125105 CrossRef PubMed.
- T. Maly, G. T. Debelouchina, V. S. Bajaj, K. N. Hu, C. G. Joo, M. L. Mak-Jurkauskas, J. R. Sirigiri, P. C. A. van der Wel, J. Herzfeld, R. J. Temkin and R. G. Griffin, J. Chem. Phys., 2008, 128, 052211 CrossRef PubMed.
- C. Song, K. N. Hu, C.-G. Joo, T. Swager and R. G. Griffin, J. Am. Chem. Soc., 2006, 128, 11385 CrossRef CAS PubMed.
- W. Th. Wenckebach, T. J. B. Swanenburg and N. J. Poulis, Phys. Rep., 1974, 14, 181 CrossRef.
- B. N. Provotorov, Sov. Phys. JETP, 1962, 14, 1126 Search PubMed.
- V. A. Atsarkin, Usp. Fiz. Nauk, 1978, 126, 3 CrossRef CAS.
- C. D. Jeffries, Annu. Rev. Nucl. Sci., 1964, 14, 101 CrossRef CAS.
- T. Prisner and W. Kockenberger, Appl. Magn. Reson., 2008, 34, 213 CrossRef CAS.
- R. G. Griffin and T. F. Prisner, Phys. Chem. Chem. Phys., 2010, 12, 5737 RSC.
- J. H. Ardenkjaer-Larsen, B. Fridlund, A. Gram, G. Hansson, M. Lerche, R. Servin, M. Thaning and K. Golman, Proc. Natl. Acad. Sci. U. S. A., 2003, 100, 10158 CrossRef CAS PubMed.
- D. G. Gadian, K. S. Panesar, A. J. Perez Linde, A. J. Horserwill, W. Köckenberger and J. R. Owers-Bradley, Phys. Chem. Chem. Phys., 2012, 14, 5397 RSC.
- D. T. Peat, A. J. Horsewill, W. Köckenberger, A. J. Perez Linde, D. G. Gadian and J. R. Owers-Bradley, Phys. Chem. Chem. Phys., 2013, 15, 758 RSC.
- J. Owers Bradley, A. J. Horsewill, D. T. Peat, K. S. H. Goh and D. Gadian, Phys. Chem. Chem. Phys., 2013, 15, 10413 RSC.
- R. Tycko, 18th ISMAR Meeting, Rio de Janeiro, May 2013.
- B. Corzilius, L. B. Andreas, A. A. Smith and Q. Z. Ni. R. Griffin, J. Magn. Reson., 2014, 240, 113 CrossRef CAS PubMed.
- K. Thurber and R. Tycko, J. Phys. Chem., 2014, 140, 184201 CrossRef PubMed.
- K. H. Langley and C. D. Jeffries, Phys. Rev., 1966, 152, 358 CrossRef CAS.
- C. D. Jeffries, Cryogenics, 1963, 3, 41 CrossRef.
- A. Abragam, Cryogenics, 1963, 3, 42 CrossRef.
- J. R. McColl and C. D. Jeffries, Phys. Rev. Lett., 1966, 16, 8 CrossRef.
- J. P. Wolfe and D. C Jeffries, Phys. Rev., 1971, 4, 3 Search PubMed.
- H. B. Brom and W. J. Huiskamp, Physica, 1972, 60, 163 CrossRef CAS 1973, 66, 43.
- M. E. Halse, J.-N. Dumez and L. Emsley, J. Chem. Phys., 2012, 136, 224511 CrossRef PubMed.
- G. A. Sierra and A. Schweiger, Rev. Sci. Instrum., 1997, 68(2), 1326 CrossRef PubMed.
- G. A. Sierra, M. A. Schuler and A. Schweiger, Chem. Phys. Lett., 1999, 303, 475 CrossRef CAS.
- D. Hessinger, C. Bauer, G. Jeschke and H. W. Spiess, Appl. Magn. Reson., 2001, 20, 17 CrossRef CAS.
- N. Bloembergen, Physica, 1949, 3, 4 Search PubMed.
- W. E. Blumberg, Phys. Rev., 1960, 119, 1 CrossRef.
- S. Lange, A. H. Linden, Ü. Akbey, W. T. Franks, N. Loening, B.-J. Van Rossum and H. Oschkinat, J. Magn. Reson., 2012, 216, 209 CrossRef CAS PubMed.
- F. Mentink-Vigier, COST Meeting near Southampton, 2014, private communication.
- R. N. Purusottam, G. Bodenhausen and P. Tekely, J. Magn. Reson., 2014, 246, 69 CrossRef CAS PubMed.
- K. R. Thurber and R. Tycko, J. Magn. Reson., 2009, 196, 84 CrossRef CAS PubMed.
- P. Miéville, V. Vitzthum, M. A. Caporini, S. Jannin, S. Gerber-Lemaire and G. Bodenhausen, Magn. Reson. Chem., 2011, 49, 689 CrossRef PubMed.
- M. Rosay, L. Tometich, S. Pawsey, R. Bader, R. Schauwecker, M. Blank, P. M. Borchard, S. R. Cauffman, K. L. Felch, R. T. Weber, R. J. Temkin, R. G. Griffin and W. E. Maas, Phys. Chem. Chem. Phys., 2010, 12, 5850 RSC.
- A. Zagdoun, G. Casano, O. Ouari, M. Schwarzwälder, A. J. Rossini, F. Aussenac, M. Yulikov, G. Jeschke, C. Copéret, P. Tordo and L. Emsley, J. Am. Chem. Soc., 2013, 135, 12790 CrossRef CAS PubMed.
- W. J. Potter and H. J. Stapleton, Phys. Rev. B: Condens. Matter Mater. Phys., 1972, 5, 1729 CrossRef.
- J. H. Ardenkjaer Larsen, S. Macholl and H. Johannesson, Appl. Magn. Reson., 2008, 34, 509 CrossRef CAS.
- J. W. Gordon, S. B. Fain and I. J. Rowland, Magn. Reson. Med., 2012, 68, 4949 CrossRef PubMed.
- H. Johannesson, S. Macholl and J. H. Ardenkjaer-Larsen, J. Magn. Reson., 2009, 197, 167 CrossRef CAS PubMed.
- V. K. Michaelis, A. A. Smith, B. Corzilius, O. Haze, T. M. Swager and R. G. Griffin, J. Am. Chem. Soc., 2013, 135, 2935 CrossRef CAS PubMed.
- T. Kobayashi, O. Lafon, A. S. Lilly Thankamony, I. I. Slowing, K. Kandel, D. Carnevale, V. Vitzthum, H. Vezin, J.-P. Amoureux, G. Bodenhausen and M. Pruski, Phys. Chem. Chem. Phys., 2013, 15, 5553 RSC.
- P. Lueders, S. Razzaghi, H. Jäger, R. Tschaggelar, M. A. Hemminga, M. Yulikov and G. Jeschke, Mol. Phys., 2013, 111, 2824 CrossRef CAS.
- A. J. Rossini, A. Zagdoun, M. Lelli, D. Gajan, F. Rascon, M. Rosay, W. E. Maas, C. Copéret, A. Lesage and L. Emsley, Chem. Sci., 2012, 3, 108 RSC.
- O. Lafon, A. S. L. Thankamony, M. Rosay, F. Aussenac, X. Lu, J. Trébosc, V. Bout-Roumazeilles and J. P. Amoureux, Chem. Commun., 2013, 49, 2864 RSC.
- K. Thurber and R. Tycko, J. Chem. Phys., 2012, 137, 084508 CrossRef PubMed.
- F. Mentink-Vigier, Ü. Akbey, Y. Hovav, S. Vega and H. Oschkinat, J. Magn. Reson., 2012, 224, 13 CrossRef CAS PubMed.
- A. N. Pravdivtsev, A. V. Yurkovskaya, R. Kaptein, K. Miesel, H. M. Vieth and K. L. Ivanov, Phys. Chem. Chem. Phys., 2013, 15, 14660 RSC.
|
This journal is © the Owner Societies 2015 |