DOI:
10.1039/C6RA13291J
(Paper)
RSC Adv., 2016,
6, 72213-72223
pH-Assisted surface functionalization of selenium nanoparticles with curcumin to achieve enhanced cancer chemopreventive activity†
Received
23rd May 2016
, Accepted 22nd July 2016
First published on 25th July 2016
Abstract
A facile pH-assisted method for synthesizing curcumin-functionalized selenium nanoparticles (SeNPs@Cur) with enhanced cancer chemopreventive activity is proposed in the present work. It is intriguing to observe that by controlling the pH value of the synthetic reaction system, water-insoluble Cur is successfully decorated on the surfaces of SeNPs@Cur through physical adsorption and the possible formation of Se–O bonds. Moreover, the as-prepared nanocomposites possess high drug conjugation efficiency (5.5%), good chemical stability and more uniform sizes and morphologies in comparison with bare SeNPs. Meanwhile, enhanced chemopreventive activity of SeNPs@Cur is demonstrated through the improvements of their free radical scavenging activities and the increases of their cytotoxicities against cancer cells. Cur surface decoration also increases cellular uptake of elemental Se and apoptosis in HepG2 cells. Studies on underlying mechanisms suggest that SeNPs@Cur-induced apoptosis is dependent on the activation of caspases and the overproduction of intracellular ROS. In conclusion, data herein indicate that the strategy to assemble antioxidant ligands on the surface of SeNPs is a potential way to design novel chemopreventive agents with better safety, tolerability and efficacy profiles.
Introduction
Cancer chemoprevention, which is defined as the use of specific natural or synthetic chemical agents to reverse, prevent, or delay carcinogenesis to invasive cancer, has been widely considered to be an appealing, promising and cost-effective approach to reduce cancer morbidity and mortality in well-defined high-risk groups and in the general population.1–4 Over the past few decades, around 2000 agents have exhibited significant preventive activities against cancers in experimental systems, and more than 40 promising agents and agent combinations have been evaluated in clinical.5–7 For example, randomized trials revealed that tamoxifen apparently reduced the incidence of invasive and non-invasive breast cancer in women and celecoxib showed tremendous activity in prevention of colorectal adenomas.8–10 But long-term use of these drugs is severely hindered by an increased risk of the occurrence of serious side effects and drug resistance.11–13 Accordingly, natural products are supposed to be more attractive alternatives to synthetic drugs.14 Extensive preclinical data indicated that phytochemicals could protect against cancers effectively, however, these results failed to translate as successful outcomes in clinical settings.15–17 Recently, it is recognized that the unsatisfactory consequences of natural compounds are mostly attributed to their poor bioavailability, which results in sub-therapeutic concentrations at target sites.18 For example, curcumin (Cur) which is extracted from the rhizome of Curcuma longa (turmeric) has been proved to exhibit diverse pharmacologic effects in cell-culture studies, such as anti-oxidant, anti-inflammatory, anti-carcinogenic, anti-proliferative and anti-angiogenic activities.19–22 Whereas, its introduction into clinical utility is seriously hampered by its low solubility in weakly acidic or neutral aqueous solution, and consequently poor absorption and bioavailability, followed by rapid metabolism and systemic elimination.23 To circumvent this problem, drug delivery systems of Cur such as liposomes, microemulsions and polymeric implantable devices have been explored diffusely, while obvious disadvantages including complex processes, poor stability, expensive cost and organic solvent residues, have shown up in these processes.24,25 Interestingly, it has been revealed previously that the aqueous solubility of Cur can be increased by up-regulating the pH value of the solution to the alkaline pH range.26 This is mainly due to the acidic phenol group in Cur could donate its hydrogen at alkaline pH, forming the phenolate ion that enables Cur into dissolution in water.27
Selenium (Se), an essential trace element for humans and animals, has been well documented as one of the most valuable and efficient cancer chemopreventive agents.28–31 Numerous epidemiological, preclinical and clinical studies suggested that Se supplementation could reduce the incidence of a series of cancers, such as prostate, lung, mammary, colon and liver cancers.32–42 Nevertheless, both inorganic and organic selenocompounds display a very narrow margin between beneficial and toxic effects which greatly limit their applications.43–45 Thanks to the emergence of nanotechnology, selenium nanoparticles (SeNPs) have attracted increasing considerations in the last years because of its excellent biological activities, low toxicities and important therapeutic properties.46–48 It has also been reported that SeNPs perform greater potentials than other Se species for local delivery of high dosage of elemental Se into cancer cells because of their higher density. Meanwhile, SeNPs with various sizes and shapes can be synthesized and purified simply from redox reaction systems.49–51 However, pure SeNPs will aggregate seriously in aqueous, especially under physiological conditions, resulting in poor anticancer activity.52 Thus, pure SeNPs should be decorated with other molecules to enhance their solubility and stability.
In order to combine the advantages of Cur and SeNPs and overcome their shortcomings in cancer chemoprevention, a facile, organic solvent-free and pH-assisted strategy for fabricating curcumin-functionalized selenium nanoparticles (SeNPs@Cur) is proposed in the present work, in which Cur is directly conjugated to the surface of SeNPs through physical adsorption and the possible formation of Se–O bonds. The water solubility of Cur increases by about 4.5 times and SeNPs@Cur show better stability in comparison with bare SeNPs. More importantly, SeNPs@Cur well combine the advantages of SeNPs with Cur in cancer chemoprevention, which is supported not only by the improvements of their free radical scavenging activities, but also by the increase of cellular uptake of elemental Se and caspase- and ROS-mediated apoptosis in cancer cells (Scheme 1). Thus, SeNPs@Cur can be regarded as an extremely potent agent for cancer chemoprevention and chemotherapy.
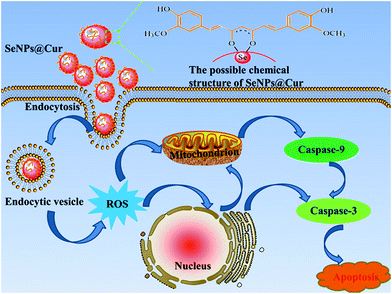 |
| Scheme 1 Schematic illustration of the proposed pathways for the apoptosis induced by SeNPs@Cur in HepG2 cells. | |
Results and discussion
Synthesis and characterization of SeNPs@Cur
It has been mentioned that the aqueous solubility of Cur can be improved by adjusting the pH value of the solution to an alkaline range. However, in fact, this approach leads to another main challenge: in alkali medium, Cur lacks stability and undergoes rapid hydrolytic degradation followed by molecular fragmentation.26,53 Interestingly, Tønnesen and Karlsen have pointed out that the degradation rate constant shows a minimum at pH 8.5, where Cur is postulated to be in an equilibrium between its three forms: H3Cur, H2Cur− and HCur2− (Fig. S1†).25 Accordingly, before formal experiments, the solubility and stability of Cur in an aqueous solution at pH 8.5 was measured under the synthetic conditions without the addition of H2SeO3 and ascorbic acid solutions. As shown in Fig. S2,† the solution of free Cur becomes cloudy and yellow precipitates are formed when an appropriate volume of Cur stock solution is added to it directly. Conversely, when the pH value of water is adjusted to 8.5, the solution keeps clear and Cur dissolves completely though its colour changes to red, showing that the solubility of Cur increases with the increase of the solution's pH value. Meanwhile, the absorption spectra acquired at fixed time intervals are presented in Fig. S2B.† Although the intensity of the maximum absorption at 465 nm decreases in a time-dependent manner and appears to be 5 nm blue shifted, more than 90% of the Cur is left in the solution after 3 h. Therefore, the pH value of the reaction system in this study was determined to be 8.5. And subsequently, a facile, fast and green method was suggested to synthesize Cur-functionalized SeNPs. As shown in Fig. S3C and D,† the fluorescence and UV-vis spectra of Cur extracted from SeNPs@Cur are in agreement with those of free Cur dissolved in DMSO, suggesting that no changes have occurred in the structure of Cur after being decorated on the surface of SeNPs. The amount of Cur on the surface of SeNPs@Cur was detected with a UV-vis spectrophotometer after it was completely extracted with dichloromethane and re-dissolved in DMSO (Fig. S3A and B†). Calculated according to the calibration curve (Fig. S3E†), the drug conjugation capability (DCC) and drug conjugation efficacy (DCE) of SeNPs@Cur is 4.2% and 5.5%, respectively.
The shapes and sizes of SeNPs@Cur and SeNPs were characterized by different microscopic methods. Fig. 1 shows the TEM images, SEM images and particle size distribution histograms of SeNPs with the presence (A, B and C) or absence (D, E and F) of Cur. It is clear that SeNPs@Cur present monodisperse and homogeneous spherical structure with an average diameter of 125 nm, whereas pure SeNPs are badly aggregated with an average diameter of about 1 μm. These results are similar to those of Feng et al. and Liu et al., in which SeNPs are functionalized with amino acids and 5-fluorouracil, suggesting that Cur plays an important role in regulating and controlling the size and stability of SeNPs.56,57 To characterize the chemical composition and structure of SeNPs@Cur, optical properties of SeNPs before and after loading with Cur are exhibited in Fig. 2. Based on the UV-vis spectra, it is found that the maximum absorbance of SeNPs@Cur is around 357 nm while SeNPs show the strongest absorbance at 660 nm (Fig. 2A). Although the characteristic peaks of Cur aqueous solution (at 345 and 419 nm) don't show up in the spectrum of SeNPs@Cur, a significant blue shift of the spectral position of SeNPs plasmon band absorption is found, describing the interaction of Cur and SeNPs.54 In addition, Cur is known to possess robust fluorescence emission peaked at 545 nm when excited at 420 nm.55 However, after being loaded onto SeNPs, its fluorescence is dramatically quenched (Fig. 2B), indicating the possible presence of fluorescence resonance energy transfer (FRET) between SeNPs and Cur. Similar results have also been reported by Kundu et al. and Singh et al.58,59 To further prove the adsorption of Cur molecules on the surface of SeNPs, SeNPs@Cur, SeNPs and Cur were also characterized by FTIR. As shown in Fig. 2C, the FTIR spectrum of SeNPs@Cur strongly resembles those of Cur and SeNPs, giving clear evidence that Cur and SeNPs form parts of the nanocomposite. In the spectrum of Cur standard powder, the peak at 1653 cm−1 corresponds to a contemporaneous contribution of C
C and C
O stretching mode, whereas the peak at 1600 cm−1 is assigned to the C
C stretching vibration of the aromatic ring. The peak at 1508 cm−1 comes from the superimposition of ν(C
C ring) and ν(C
O) vibrations. The range of 1500–1350 cm−1, characterized by the peaks at 1454, 1431 and 1384 cm−1, is the region of the in-plane bending vibrations of C–H, skeletal CCC and aromatic CCC and CCH modes. A strong single at 1286 cm−1 can be attributed to the ν(C–O) of the enol function. Another C–O–C stretching and the out of plane bending of CH3 together with the in plane bending of aromatic CCH bands are apparent in the range of 1250–900 cm−1.60,61 The appearance of the peaks at 1653, 1508, 1454 and 1286 cm−1 in the spectrum of SeNPs@Cur demonstrate the presence of Cur on the surface of SeNPs. Furthermore, the obvious transmittance weakness of the peaks at 1508 and 1286 cm−1 suggest the involvement of ketone group and enol structure in the interaction between Cur and SeNPs, which is similar to the results of Kundu et al. and Magro et al.58,62 Last but not least, XPS spectra were measured to confirm the formation of chemical bonds between Cur and Se. As shown in Fig. 2D, the XPS spectrum of SeNPs@Cur is similar to that of SeNPs because their elemental compositions are the same. However, the peaks of Se 3d5/2 and 3d3/2 increase from 55.17 and 55.77 eV (SeNPs) to 55.28 and 56.09 eV (SeNPs@Cur), respectively (Fig. 2E), indicating that Se 3d orbit participates in the bonding in the formation of SeNPs@Cur. More notably, the peaks of O 1s split into two in the fitting spectra of SeNPs@Cur and Cur, whereas there is only one peak in the fitting spectrum of SeNPs (Fig. 2F). And the shift of the peak at 532.4 eV to 532.67 eV in SeNPs@Cur may be related to the formation of Se–O bonds.
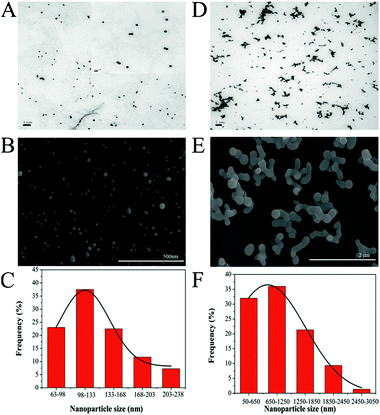 |
| Fig. 1 . TEM images, SEM images and particle size distribution histograms of SeNPs@Cur (A, B and C) and SeNPs (D, E and F). The inset is a 10 times magnification of micrograph (A). | |
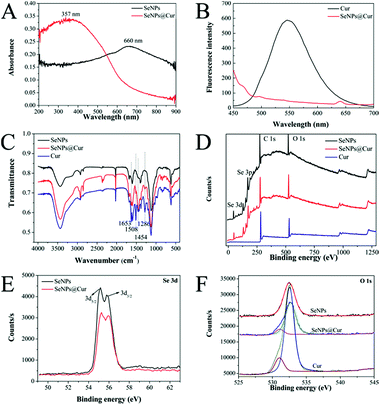 |
| Fig. 2 Chemical composition and structure characterizations of SeNPs@Cur. (A) UV-vis spectra of SeNPs@Cur and SeNPs. (B) Fluorescence spectra of SeNPs@Cur and Cur. (C) FTIR spectra of SeNPs@Cur, SeNPs and Cur. (D) XPS spectra of SeNPs@Cur, SeNPs and Cur. (E) Se 3d spectra of SeNPs@Cur and SeNPs. (F) O 1s spectra of SeNPs@Cur and Cur. | |
Taken together, besides typically unspecific adsorption of Cur on the surface of SeNPs resulting from nanoparticles' high surface energy, the possible formation of Se–O bonds also plays an important role in the construction of SeNPs@Cur which are supported by the changes in UV-vis, fluorescence, FTIR and XPS spectra. Similarly, Singh et al. have confirmed the binding of an O atom of the curcumin molecule to the AuNPs surfaces.59 And Magro and co-workers have proposed that Cur may be firmly bound on the surface of superparamagnetic nanoparticles through Fe–O bonds during the synthesis reaction.62
Stability is an important issue for the future applications of nanoparticles.46 In order to further verify the positive effect of Cur on the stability of SeNPs, changes in the intensity of the maximum absorption of SeNPs and SeNPs@Cur in aqueous solution, PBS buffer and serum-free medium were monitored within 50 h. As displayed in Fig. 3A and B, the absorbance of SeNPs@Cur in aqueous solution, PBS buffer and serum-free medium at 357 nm can hold steady until 12 h and drops slightly after that, however, the absorbance of SeNPs at 660 nm decreases dramatically after 4 hours' standing, explaining the higher stability of SeNPs@Cur than SeNPs. On the other hand, the protective effect of SeNPs on Cur was also measured by examining the stability of Cur in buffer solution or in serum-free medium. The results in Fig. 3C and D reveal that the amount of Cur in SeNPs@Cur could remain about the same for 36 h at 37 °C in both PBS buffer solution and RPMI 1640 medium. In contrast, free Cur freshly prepared in absolute ethanol undergoes rapid degradation immediately when it is diluted in PBS buffer solution or RPMI 1640 medium and exposed to the surroundings. Similarly, Wang et al. reported previously that Cur degraded rapidly not only in 0.1 M phosphate buffer but also in serum-free medium after 1 h-incubation at 37 °C.53 These results indicate that the conjugation of SeNPs and Cur could enhance the stability of each other under physiological conditions. Moreover, the high stability of SeNPs@Cur powerfully supports their future chemopreventive applications.
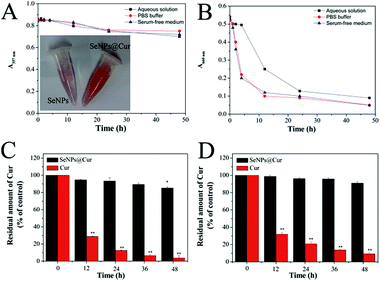 |
| Fig. 3 In vitro stability of SeNPs@Cur. (A) Storage stability of SeNPs@Cur in aqueous solution, PBS buffer and serum-free medium. (B) Storage stability of SeNPs in aqueous solution, PBS buffer and serum-free medium. (C) Degradation profiles of free Cur and Cur loaded on the surface of SeNPs@Cur in PBS buffer. (D) Degradation profiles of free Cur and Cur loaded on the surface of SeNPs@Cur in serum-free medium. The Inset is the photograph of the solutions of SeNPs and SeNPs@Cur. | |
Cancer chemopreventive activity of SeNPs@Cur
Carcinogenesis is viewed as a process that involves accelerated and abnormal cellular changes, in which the genes controlling proliferation, differentiation and apoptosis are transformed under selective environmental pressures.2,3 Thus, a practical chemopreventive agent should intervene in the multistage process of carcinogenesis to inhibit the transformation of normal cells to premalignant cells or suppress the development of premalignant cells to malignant cells.
Free radical scavenging effects of SeNPs@Cur
It is well known that excessive free radicals can alter the cellular antioxidant defense system, cause DNA damage and result in many diseases.35,45 Moreover, these highly reactive compounds are found to participate in both initiation and promotion phases of carcinogenesis.63 Hence, free radical scavengers which can protect cells against oxidative damages and prevent the initiation and/or promotion of carcinogenesis are shown to be anticarcinogens. Thus, the free radical scavenging effects of SeNPs@Cur, SeNPs and Cur were examined and shown in Fig. 4 and S4.† The scavenging activities of SeNPs@Cur against DPPH and hydroxyl free radicals increase significantly in a dose-dependent manner and are much higher than those of SeNPs (Fig. 4A). The scavenging effect of Cur on DPPH free radicals also increases rapidly. However, its effect on hydroxyl free radicals increases very slowly within the prescribed concentration range, which may partly result from its different solubility in the two reaction systems (Fig. 4B). These results demonstrate the distinct enhancement of free radical scavenging activities of SeNPs by Cur surface decoration. Furthermore, the synergistic interactions between SeNPs and Cur occurred in the free radical scavenging processes were analyzed by isobologram method.52 To DPPH free radicals, the IC50 values for SeNPs@Cur, SeNPs and Cur are found at 18.2, 560.0 and 1.1 μg mL−1, respectively (Fig. 4C). And to hydroxyl free radicals, the IC50 values for SeNPs@Cur, SeNPs and Cur are separately found at 70.7, 888.9 and 27.3 μg mL−1 (Fig. 4D). The results of the isobologram analysis suggest that the free radical scavenging effects between SeNPs and Cur in the SeNPs@Cur system are strongly synergistic, as evidenced by the location of the data point in the isobologram being far below the line defining an additive effect. Moreover, the actual IC50 values of the SeNPs@Cur (18.2 and 70.7 μg mL−1, respectively) are much lower than the theoretical values (209 and 700 μg mL−1, respectively). And the combination indexes (CI) of the IC50 values of SeNPs@Cur are separately found around 0.087 (<1) and 0.088 (<1), further confirming the synergism between SeNPs and Cur. These results indicate that the strategy to assemble antioxidant ligands on the surface of SeNPs may be a highly efficient way to enhance their cancer chemopreventive activities.
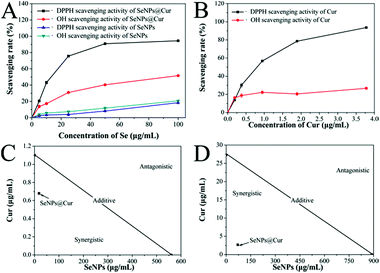 |
| Fig. 4 Free radical scavenging activity of SeNPs@Cur. (A) Scavenging effects of SeNPs@Cur and SeNPs on DPPH and hydroxyl radicals. (B) Scavenging effects of Cur on DPPH and hydroxyl radicals. (C and D) Isobologram analysis of the scavenging activities of SeNPs and Cur against DPPH radicals. (D) Isobologram analysis of the scavenging activities of SeNPs and Cur against hydroxyl radicals. | |
Growth inhibition of cancer cells by SeNPs@Cur
It is well known that HepG2 (hepatocellular carcinoma cell line) and MCF-7 cells (human breast adenocarcinoma cell line) have widespread usage in pharmacology and toxicology studies and have been employed to estimate the cancer chemopreventive effects of a number of chemicals.2,4 On the other hand, HBMECs (Human Brain Microvascular Endothelial Cells) and BRL-3A cells (rat normal hepatocyte cell line) were selected as normal in vitro models to assess the cytotoxicity of the nanocomposites.64,76 Thus, the cell viability of MCF-7, HepG2, HBMECs and BRL-3A cells was evaluated through MTT assay after they were treated with SeNPs@Cur and SeNPs. As shown in Fig. 5A and B, SeNPs@Cur significantly inhibit the growth of MCF-7 and HepG2 cells in a dose- and time-dependent manner with IC50 values of 11.1 and 8.1 μg mL−1, respectively. However, they exhibit no effect on the viability of HBMECs and BRL-3A cells within the concentration range of 0–100 μg mL−1, suggesting that SeNPs@Cur have great selectivity between cancer and normal cells. According to the results of chemical composition characterization of SeNPs@Cur, the cytotoxicities of SeNPs and Cur toward HBMECs, BRL-3A, MCF-7 and HepG2 cells at corresponding concentrations were also evaluated to determine their own effects on the nanocomposites. As shown in Fig. 5C and D, the inhibitory effects of SeNPs against MCF-7 and HepG2 cells are facilitated in a dose- and time-dependent mean as well. But their IC50 values (20.2 and 20.0 μg mL−1) are much higher than those of SeNPs@Cur. Moreover, SeNPs display an obvious cytotoxicity against BRL-3A cells with the increase of their concentrations. For instance, by contrast with the control group, the viability of BRL-3A cells decreases to 86% and 66% at 50 and 100 μg mL−1 of SeNPs, respectively. Otherwise, the cytotoxicities of Cur toward the four cell lines are reported in Fig. 5E. It is found that Cur shows distinct cytotoxicities against MCF-7 and HepG2 cells at the concentrations of 1.89 and 3.78 μg mL−1, but no cytotoxicity against HBMECs and BRL-3A cells within the concentration range of 0–3.78 μg mL−1, which are similar to the results of Syng-ai et al.65 Therefore, the synergistic interaction between SeNPs and Cur in the SeNPs@Cur system will show up under high concentrations (50 and 100 μg mL−1). Furthermore, the surface functionalization of SeNPs with Cur not only enhances their anticancer activities but also significantly improves their safety toward normal cells.
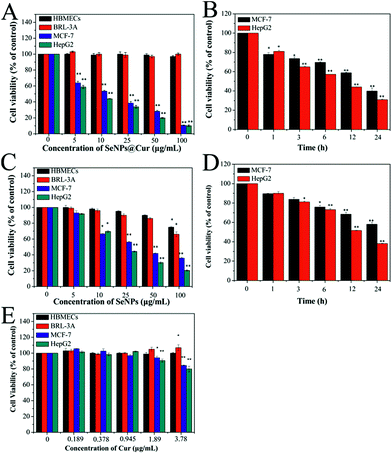 |
| Fig. 5 Cytotoxicity of SeNPs@Cur measured by MTT assay. (A) Cell viability of BRL-3A, MCF-7 and HepG2 cells treated with SeNPs@Cur at different concentrations for 24 h. (B) Cell viability of MCF-7 and HepG2 cells incubated with 25 μg mL−1 of SeNPs@Cur for various time periods. (C) Cell viability of BRL-3A, MCF-7 and HepG2 cells treated with SeNPs at different concentrations for 24 h. (D) Cell viability of MCF-7 and HepG2 cells treated with25 μg mL−1 of SeNPs for various time periods. (E) Cell viability of BRL-3A, MCF-7 and HepG2 cells treated with Cur at different concentrations for 24 h. | |
Additionally, the optical microscopic images of BRL-3A, MCF-7 and HepG2 cells following exposure to 25 μg mL−1 SeNPs or SeNPs@Cur and staining with 0.04% trypan blue solution are displayed in Fig. 6. Expectably, BRL-3A, MCF-7 and HepG2 cells in control groups are intact and remain spindle-shaped or spherical. Despite the fact that numerous red particles are attached to the surface of the cells, no significant changes in cell morphology, color and quantity are seen in BRL-3A cells when they are incubated with 25 μg mL−1 SeNPs or SeNPs@Cur. Nevertheless, exposure MCF-7 and HepG2 cells to 25 μg mL−1 SeNPs or SeNPs@Cur results in extensive cell mortality. And more cancer cells are stained blue, shrink into spheres and lost adhesion to the bottom of tissue culture dish as evidenced by significant reduction in cell numbers when they are treated with SeNPs@Cur. These results indicate that SeNPs and SeNPs@Cu could cause the changes in morphology of cancer cells, and consequently inhibit the growth of cancer cells. In agreement with the results of MTT assay, under the same concentration, SeNPs@Cu with negligible toxicities towards normal cells exhibit greater cytotoxicities against cancer cells in comparison with SeNPs. Biocompatibility is an important index for the employment of nanoparticles in cancer chemoprevention. Consequently, SeNPs@Cu may be more suitable to be employed in future clinical chemoprevention studies.
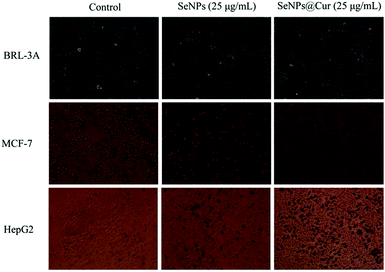 |
| Fig. 6 Phase contrast microscopy light micrographs of BRL-3A, MCF-7 and HepG2 cells treated with 25 μg mL−1 SeNPs or SeNPs@Cur and stained with 0.04% trypan blue solution (magnification 10×). | |
Intracellular uptake of SeNPs@Cur
It has been reported that cellular uptake could contribute to nanoparticle toxicity.66 In order to understand the nanoparticle cytotoxicities in more detail, qualitative and quantitative studies on the cellular uptake of SeNPs and SeNPs@Cur by the most susceptible HepG2 cells were conducted with TEM and ICP-AES. TEM images of HepG2 cells with or without treatment are shown in Fig. 7A, B and D. After 12 h of incubation with HepG2 cells, SeNPs and SeNPs@Cur are separately found in vesicles in the cytoplasm, confirming the successful cellular uptake of SeNPs and SeNPs@Cur through endocytosis.63 As shown in magnifying images (Fig. S5†), the particle sizes of SeNPs and SeNPs@Cur within the vesicles are in keeping with the results shown in Fig. 1, though SeNPs@Cur are apparent to be clustered. For a single cell, more vesicles containing nanoparticles are readily observed when it is treated with SeNPs@Cur (labeled by red arrows). In addition, the contents of elemental Se in 1 × 106 cells after treatment with different concentrations of nanoparticles are shown in Fig. 7C. Not surprisingly, the amount of SeNPs or SeNPs@Cur penetrated into HepG2 cells increases in a dose-dependent manner. But apparently, the amount of SeNPs@Cur uptake is much higher than that of SeNPs at each concentration, suggesting the enhancement of cellular uptake of SeNPs after functionalization with Cur. These results are in accordance with other studies, in which the sizes and surface characteristics of SeNPs are indicated to play a crucial role in cellular uptakes.23
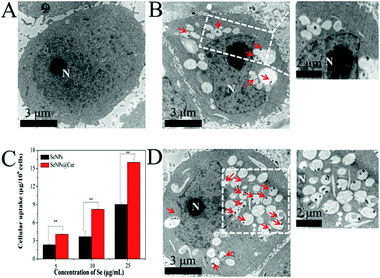 |
| Fig. 7 Intracellular uptake of SeNPs@Cur by HepG2 cells. (A, B and D) TEM images of HepG2 cells without or with the treatment of SeNPs or SeNPs@Cur (N: nucleus, red arrows: nanoparticles in endocytic vesicles, scale bar indicate 1 μm). (C) Cellular amount of Se in HepG2 cells after 12 h incubation with SeNPs or SeNPs@Cur. | |
Induction of apoptosis in HepG2 cells by SeNPs@Cur
It is worth noting that an increasing number of studies have shown that chemopreventive agents could stimulate apoptosis in premalignant and malignant cells in vitro or in vivo.67 For example, Cur was reported separately by Rao et al. and Liu et al. to have the ability to induce apoptosis in colon and breast cancer cells.19,22 And the potential mechanism for cancer chemoprevention of Se was postulated to be apoptosis.15,39 Apoptosis induction is arguably the most potent defense against cancer.68 In view of the above, the possibility of apoptosis involved in the death of SeNPs or SeNPs@Cur treated HepG2 cells was investigated by annexin V-FITC/PI flow cytometric analysis. In Fig. 8A, it is shown that the percentage of the cells undergoing apoptosis increases from 6.0% to 11.9% with an increase in the dose of SeNPs (5 μg mL−1 to 10 μg mL−1), as reflected by the combination of Q2 and Q3 populations. For SeNPs@Cur treated cells, the percentage of the apoptotic cells increases from 7.1% to 17.9% at the concentration of 5 to 10 μg mL−1, which is obviously higher than that encouraged by SeNPs. This result confirms that exposure of HepG2 cells to different concentrations of SeNPs@Cur for 24 h results in a more marked dose-dependent increase in the proportion of apoptotic cells by contrast to SeNPs.
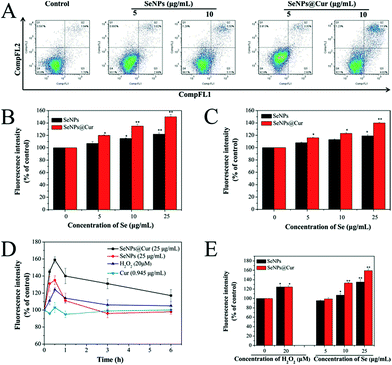 |
| Fig. 8 Induction of apoptosis in HepG2 cells by SeNPs@Cur. (A) Flow cytometric analysis of HepG2 cells exposed to SeNPs or SeNPs@Cur for 24 h based on an annexin V-FITC/PI double-staining. (B) The activation of caspase-3 induced by SeNPs or SeNPs@Cur. (C) The activation of caspase-9 induced by SeNPs or SeNPs@Cur. (D) Time courses of the production of intracellular ROS induced by SeNPs@Cur (25 μg mL−1), SeNPs (25 μg mL−1), H2O2 (20 μM), and Cur (0.945 μg mL−1). (E) The levels of ROS in HepG2 cells treated with SeNPs or SeNPs@Cur for 30 min. | |
Caspases, a family of aspartate-specific cysteine proteases, play prominent roles in the initiation and execution of apoptosis.69 Caspase-3 is regarded as the central regulator of apoptosis, while caspase-9 acts as initiator of mitochondria-mediated apoptotic pathways. To determine whether the caspase family was activated in HepG2 cells exposed to SeNPs and SeNPs@Cur, the enzymatic activities of caspase-3 and -9 were examined by fluorometric assays with specific substrates, respectively. As shown in Fig. 8B and C, the treatments with 5, 10 and 25 μg mL−1 of Se@Cur significantly promote the activation of caspase-3 and -9 in a concentration-dependent manner. The activities of caspase-3 increase from 1.2 to 1.5 folds and those of caspase-9 increase from 1.1 to1.4 folds in response to the SeNPs@Cur treatments. Meanwhile, SeNPs evoked activations of caspase-3 and -9 are clearly lower than those induced by SeNPs@Cur and distinct increase only apparent at 25 μg mL−1. And Cur shows no obvious effect on the activities of caspase-3 and -9 (Fig. S6A†). These results suggest that Se@Cur can cause more apoptosis of HepG2 cells than SeNPs and Cur through the encouragements of the activations of caspase-3 and -9. On the other hand, it has been reported that the overproduction of intracellular reactive oxygen species (ROS) may be correlated with the induction of apoptosis.70 Moreover, ROS is considered as an important regulator of mitochondrial function because mitochondrial respiratory chain is a potential source of ROS.71 Consequently, in this work, the intracellular ROS generation in HepG2 cells were measured by detecting the DCF fluorescence intensity. The time course of ROS generation induced by 25 μg mL−1 of SeNPs@Cur, 25 μg mL−1 of SeNPs, 20 μM H2O2 or 0.945 μg mL−1 of Cur is shown in Fig. 8D. It is noteworthy that the level of intracellular ROS with a peak at 30 min is significantly elevated after treatment for 15 min but followed by a gradually fall down to 360 min. In compared with the untreated groups, exposure of HepG2 cells to H2O2, SeNPs or SeNPs@Cur for 30 min visibly elevates the intracellular ROS level in a dose-dependent way as described in Fig. 9. Otherwise, under the same concentrations of 10 and 25 μg mL−1, Fig. 8E shows that SeNPs@Cur increased ROS generations (1.3 and 1.6 folds) are significantly higher than those upgraded by SeNPs (1.1 and 1.3 folds). At the same time, Fig. S6B† reveals that there is no increase in the intracellular ROS level of HepG2 cells when incubated with various concentrations of Cur. These results indicate that the combination of SeNPs and Cur synergistically elevates the intracellular ROS level and dramatically results in the induction of mitochondrial dysfunction and subsequent enhancement in apoptotic efficacy. In summary, data in this study suggest that SeNPs@Cur mediated apoptosis, as evidenced by the activations of caspases and the up-regulation of ROS stress, is a crucial mechanistic paradigm to explain their cancer chemopreventive activities (Scheme 1).
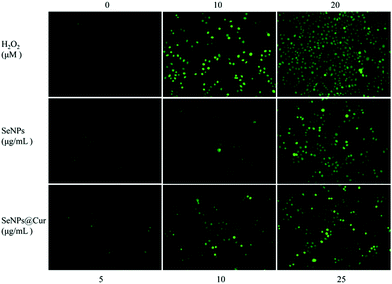 |
| Fig. 9 Phase contrast microscopy fluorescence micrographs of HepG2 cells with or without the treatments of different concentrations of H2O2, SeNPs or SeNPs@Cur (magnification 10×). | |
Experimental
Materials
All chemicals were of reagent grade and used without further purification. Selenium dioxide (SeO2, SP, 99%), ascorbic acid, curcumin (Cur) (>98% pure) were purchased from Sinopharm Group Chemical Reagent Co., Ltd. (Shanghai, China). BCA protein assay kit and Annexin V-FITC/PI apoptosis assay kit were purchased from Jiancheng Bioengineering Institute (Nanjing, China). Thiazolyl blue tetrazolium bromide (MTT), 1,1-diphenyl-2-picrylhydrazyl (DPPH) and 20,70-dichlorofluorescein diacetate (DCF-DA) were purchased from MP Biomedical Co. (CA, USA). Caspase-3 substrate (Ac-DEVD-AMC) and caspase-9 substrate (Ac-LEHD-AMC) were purchased from AAT Bioquest, Inc. (USA). RPMI 1640 medium, DMEM medium (high glucose) and fetal bovine serum (FBS) were purchased from Gibco BRL (Gaithersburg, MD). Ultrapure (UP) water (18.5 MΩ cm−1) was used in all experimental processes.
Synthesis of SeNPs@Cur
A stock solution of 5 mM H2SeO3 was prepared by dissolving 5.55 mg of SeO2 powder in 10 mL of deionized water. A 3.75 mL aliquot of 50 mM ascorbic acid aqueous solution prepared freshly was dropwise added into 7.5 mL H2SeO3 stock solution under magnetic stirring, and the mixture was reconstituted to a final volume of 75 mL with deionized water. Then the mixed solution was stirred for about half an hour at room temperature. When the colour of the solution completely became red, the pH was adjusted to 8.5 with 0.1 M NaOH and 3 mg of Cur powder dissolved in 0.5 mL absolute ethyl alcohol was added dropwise into the reaction system. The mixture was kept away from light immediately and stirred for 3 h under N2 at room temperature. After that, the nanoparticles were centrifuged at 3000 rpm for 15 min and washed with deionized water for several times. Finally, the nanoparticles were re-suspended in deionized water and the SeNPs@Cur solution was stored at 4 °C in dark until use. Se concentration of the stock solution was determined by inductively coupled plasma atomic emission spectroscopy (ICP-AES) analysis (Shimadzu).
Quantification of Cur decorated on surfaces of SeNPs@Cur
To analyze the Cur content loaded on surfaces of SeNPs@Cur, an aliquot of SeNPs@Cur solution was extracted completely with dichloromethane and stocked in DMSO finally. In brief, 1 mL of SeNPs@Cur solution (400 μg mL−1, Se concentration) was mixed with 2 mL dichloromethane and shaken vigorously for 10 min to extract Cur from SeNPs@Cur in water by solvent extraction. Then the mixture stood for 5 min at room temperature to completely separate the inorganic and organic phases. The organic extraction solvent was sucked out carefully and collected in a 25 mL beaker. These procedures were repeated several times until the solvent extraction phase didn't show the yellow color of Cur. Finally, the dichloromethane solutions of Cur were dried under N2 at room temperature. The obtained Cur powder was dissolved in an appropriate volume of dimethyl sulfoxide (DMSO) and the solution was measured for absorbance at 465 nm (Abs465) using a UV-vis spectrophotometer (UV-2550, Shimadzu). The Abs465 was used to determine the Cur concentration based on a calibration curve previously established using standard solutions with different amounts of free Cur dissolved in DMSO. Three independent replicates were measured and the whole process should pay attention to avoid light as far as possible. The DCC and DCE of SeNPs@Cur were measured to be 4.2% and 5.5%, respectively, according to the below equations:
DCC (%) = (amount of conjugated drug)/(amount of drug loaded NPs) × 100% |
DCE (%) = (amount of loaded drug)/(total amount of feeding drug) × 100% |
Characterization of SeNPs@Cur
The as-prepared product was characterized by various microscopic and spectroscopic measurements including transmission electron microscope (TEM), scanning electron microscope (SEM), UV-vis spectroscopy, fluorescence spectroscopy and Fourier transform infrared spectroscopy (FTIR). TEM samples were prepared by directly drying a drop of the SeNPs@Cur suspension onto a carbon film coated copper grid. The micrographs were obtained at an accelerating voltage of 80 kV under TEM (HT7700, Hitachi High-Tech). A thin film of the sample was prepared on a silicon chip and subjected to SEM (S-4800, Hitachi High-Tech) to obtain SEM images. To determine the chemical composition of nanoparticles, FTIR (TENSOR27, Bruker) and UV-vis studies were conducted in the range of 400–4000 cm−1, 200–900 nm, respectively. And the fluorescent emission spectrum was recorded by a fluorescence spectrophotometer with excitation at 425 nm (F-5301, Shimadzu).
XPS measurements of SeNPs@Cur
X-ray photoemission spectroscopy (XPS) measurement was carried out on an ESCALab 250Xi spectrometer (Thermo Scientific) with the monochromatic Al KR X-ray radiation (energy 1486.6 eV, 500 μm spot-size). A low energy electron gun was used for the static charge compensation. The pass energy and step size were set at 100 and 1.0 eV for the survey scans and 15 and 0.1 eV for the narrow scans, respectively.
In vitro stability of Cur in SeNPs@Cur system
The in vitro stability of Cur in SeNPs@Cur was examined using the method described by Wichitnithad et al. with some modifications.27 Briefly, stock solutions of Cur (15 μg mL−1) dissolved in absolute ethanol and SeNPs@Cur (400 μg mL−1) diluted in water were prepared separately to have an equal concentration of Cur. The two stock solutions were diluted with 0.1 M phosphate buffer saline (PBS, pH 7.4) or commercial RPMI 1640 medium to a final Cur concentration of 1 μg mL−1 and left to stand at 37 °C for 48 h. The residual contents of Cur in the solutions were determined at appropriate time intervals using a UV-vis spectrophotometer. And the residual percentages were calculated to express the stability of Cur in buffer solution or in serum-free medium.
Cell lines and cell culture
Cancer cells including MCF-7 and HepG2 and normal cells including HBMECs and BRL-3A were purchased from Kunming Institute of Zoology, Chinese Academy of Science (Kunming, China) and used in this study. All cell lines were maintained in RPMI-1640 or DMEM (high glucose) media supplemented with penicillin (100 units per mL), streptomycin (50 units per mL) and fetal bovine serum (10%) at 37 °C in a humidified incubator (5% CO2, 95% air).
Cytotoxicity of SeNPs@Cur
Cell viability was determined using MTT assay based on the reduction of MTT to a purple formazan dye by mitochondrial dehydrogenase in live cells.72 Stock solutions of SeNPs@Cur (400 μg mL−1) and SeNPs (400 μg mL−1) dissolved in deionized water and stock solution of Cur (378 μg mL−1) dissolved in absolute ethanol were diluted with RPMI-1640 or DMEM (high glucose) media to obtain defined concentration gradients. Obviously, the highest concentration of ethanol in Cur treatment groups was less than 1% (v/v) and its cytotoxicity against MCF-7, HepG2 and BRL-3A cells was really negligible. On the other hand, cells were harvested in the exponential phase of growth, seeded at a density of 3 × 105 cells per mL in 96-well polystyrene culture plates and allowed to adhere for 24 h. Then cells were incubated with SeNPs@Cur, SeNPs or Cur at different concentrations for different periods of time. After treatment, the medium was removed and 20 μL per well of MTT solution (5 mg mL−1 in PBS) was added to the wells and incubated for another 4 h. The formazan crystals formed by live cells were solubilized with 100 μL DMSO and absorbance was measured at 595 nm with a microplate reader (Bio-Rad Laboratories Ltd, China).
In addition, altered cell morphology was observed with a phase contrast microscope (Olympus Optical Co., Ltd, Tokyo, Japan). Cells were seeded and treated as described above. However, after 24 h of incubation, the cells were washed three times with PBS and stained with 0.04% trypan blue solution for 10 min. Finally, cells were washed three times with PBS again and cell morphological images were scanned under the light microscope (100×).
Cellular uptake of SeNPs@Cur in HepG2 cells
The intracellular contents of SeNPs and SeNPs@Cur in HepG2 cells were quantified by measuring the amount of elemental Se in the cells.73 Twenty four hours after seeding in 6-well culture plates, the cells were treated with SeNPs or SeNPs@Cur solutions at concentrations of 5, 10 and 25 μg mL−1 for another 12 h. To completely remove the unbound nanoparticles, the cells were washed five times with PBS. Then, they were harvested, centrifuged to precipitate and digested with 3 mL concentrated nitric acid and 1 mL H2O2 in a digestive stove at 180 °C for 3 h to dissolve all the components including nanoparticles. Ionized samples were diluted with deionized water to a proper concentration and Se contents were detected using ICP-AES.
Moreover, to observe the cellular uptakes of SeNPs and SeNPs@Cur, ultrathin sections of HepG2 cells were prepared and analyzed by TEM.74 Briefly, the cells treated with 10 μg mL−1 SeNPs or SeNPs@Cur were washed five times with PBS to get rid of excess unbound NPs. Cells were trypsinized and washed 3 times in PBS and fixed in Karnovsky's fixative (5% glutaraldehyde + 4% formaldehyde in 0.1 M cacodylate buffer + 50 mg CaCl2/100 mL) for 2 h. Fixed cells were washed 3 times with PBS. Post-fixation staining was done using 1% osmium tetroxide for 1 h at room temperature. The samples were dehydrated in alcohol (70, 80, 90, 95, and 100% ethanol) and treated twice with propylene oxide for 30 min, followed by treatment with propylene oxide and Spurr's low viscosity resin for 16 h. Samples were further treated with pure resin for 24 h and embedded in molds containing pure resin. Resin blocks were hardened at 70 °C for 2 days. Ultrathin sections (70 nm) were cut using Reichert Jung Ultracut (Reichert-Jung, Wien, Austria). The sections were stained with 1% lead citrate and 0.5% uranyl acetate and analyzed under TEM.
Free radical scavenging effects of SeNPs@Cur
DPPH and hydroxyl free radical scavenging activities of Se@Cur were assessed as previously reported.72
Apoptosis assay of HepG2 cells induced by SeNPs@Cur
Normal, apoptotic, and necrotic cells can be successfully distinguished with annexin V-FITC/PI double-staining assay.75 Cell apoptosis in this study was detected by an annexin V-FITC/PI assay kit according to the manufacturer's instructions. In general, HepG2 cells were seeded in 6-well culture plates, and treated with 5 and 10 μg mL−1 of SeNPs or SeNPs@Cur for 24 h. After incubation, 1 × 106 cells were washed with PBS and resuspended in 400 μL of binding buffer. Then 5 μL of annexin V-FITC was added to the samples. The tubes were incubated for 15 min at 4 °C in the dark. Then 10 μL of propidium iodide (PI) was added and the tubes were incubated for 5 min at 4 °C in the dark. Finally, flow cytometry analysis of 2 × 104 cells was conducted within 45 min.
Activities of caspase-3 and -9
After indicated treatment, harvested HepG2 cell pellets were re-suspended in cell lysis buffer and incubated on ice for 1 h. After centrifugation at 11
000g for 30 min, the supernatants were collected and immediately measured for protein concentration by a BCA protein assay kit. To assay caspase activities, the supernatants of cell lysates were placed in 96-well plates and the specific caspase substrates (Ac-DEVD-AMC for caspase-3, and Ac-LEHD-AMC for caspase-9) were added. Then the plates were incubated at 37 °C for 1 h, and caspase activity was determined by fluorescence intensity with the excitation and emission wavelengths set at 380 and 440 nm, respectively.
Generation of intracellular ROS
Intracellular ROS accumulation was evaluated by DCF fluorescence assay. Briefly, after treatment with 0 to 25 μg mL−1 SeNPs or SeNPs@Cur, cells were harvested and suspended in PBS containing 10 mM of DCFH-DA (1 × 106 cells per ml). After incubation at 37 °C for different time intervals, the stained cells were collected, washed and re-suspended in PBS. Then, the ROS level was determined by measuring the fluorescence intensity using a microplate reader with the excitation and emission wavelengths set at 500 and 529 nm, respectively.
Statistical and synergy analysis
All experiments were carried out at least in triplicate, and data were expressed as mean ± standard deviation (S.D.). Statistical analysis was performed using SPSS statistical program version 13 (SPSS Inc., Chicago, IL). The difference between two groups was analyzed by two-tailed Student's t-test. Differences with P < 0.05(*) or P < 0.01(**) were considered statistically significant. The synergistic effect between SeNPs and Cur was evaluated by the isobologram method, in which a straight line was formed by plotting the IC50 values of SeNPs and Cur on the x- and y-axes, respectively. Data points in the isobologram correspond to the actual IC50 values of SeNPs@Cur. If a data point is on or near the line, this represents an additive treatment effect, whereas a data point that lies below or above the line indicates synergism or antagonism, respectively. Moreover, the combination index (CI) was also calculated to examine the interaction between SeNPs and Cur. A CI value of 1 indicates an additive effect between two compounds. Synergism is reflected by a combination index of <1, whereas antagonism is reflected by a combination index of >1.
Conclusions
In summary, a facile pH-assisted method has been developed in this work to synthesize Cur-functionalized SeNPs (SeNPs@Cur) with enhanced cancer chemopreventive activity. In our design, spherical SeNPs with a mean size of 125 nm are successfully capped with Cur through physical adsorption and the formation of Se–O bonds, which significantly elevate the stability of both SeNPs and Cur under physical conditions. Moreover, Cur surface decoration enhances the intracellular uptake of SeNPs through endocytosis and improves their selectivity between cancer and normal cells, which powerfully supports future in vivo applications of SeNPs@Cur. Meanwhile, SeNPs@Cur well combine the advantages of SeNPs with Cur in cancer chemoprevention, which is proved by their more excellent free radical scavenging effects and more serious cytotoxicity toward cancer cells, in contrast with SeNPs. Furthermore, studies on the underlying mechanisms reveal that as a result of the activation of caspase-3 and -9, and the overproduction of intracellular ROS, SeNPs@Cur induce obvious apoptosis in HepG2 cells, which is demonstrated to play an important role in cancer chemoprevention. Therefore, our results indicate that assembling antioxidant ligands on the surface of SeNPs is an efficient way to achieve enhanced cancer chemoprevention. SeNPs@Cur with great stability, safety and efficacy profiles may be a potential chemopreventive agent for further evaluations and long-term applications in clinical for human cancers.
Acknowledgements
This research was financed by Grants from the New Century Excellent Talents in University (NCET-13-0483), Open Fund of State Key Laboratory of Electroanalytical Chemistry (SKLEAC201301), the Shaanxi Provincial Research Fund (2014KJXX-42, 2014K02-13-03, 2014K13-10), the Yangling district research fund (2014NY-35) and Fundamental Research Funds for the Northwest A&F University of China (2014YB093, 2452015257).
Notes and references
- B. Dunn, Nature, 2012, 483, S2 CrossRef CAS PubMed.
- M. B. Sporn and N. Suh, Carcinogenesis, 2000, 21, 525 CrossRef CAS PubMed.
- J. C. Soria, E. S. Kim, J. Fayette, S. Lantuejoul, E. Deutsch and W. K. Hong, Lancet Oncol., 2003, 4, 659 CrossRef CAS PubMed.
- A. S. Tsao, E. S. Kim and W. K. Hong, Ca-Cancer J. Clin., 2004, 54, 150 CrossRef PubMed.
- S. M. Lippman, S. E. Benner and W. K. Hong, J. Clin. Oncol., 1994, 12, 851 CAS.
- R. G. Mehta, G. Murillo, R. Naithani and X. Peng, Pharm. Res., 2010, 27, 950 CrossRef CAS PubMed.
- V. M. Adhami, H. H. Bailey and H. Mukhtar, Carcinogenesis, 2014 DOI:10.1093/carcin/bgu141.
- B. Fisher, J. P. Costantino, D. L. Wickerham, C. K. Redmond, M. Kavanah, W. M. Cronin, V. Vogel, A. Robidoux, N. Dimitrov, J. Atkins, M. Daly, S. Wieand, E. Tan-Chiu, L. Ford, N. Wolmark and other National Surgical Adjuvant Breast, Bowel Project Investigators, J. Natl. Cancer Inst., 1998, 90, 1371 CrossRef CAS PubMed.
- G. Steinbach, P. M. Lynch, R. K. S. Phillips, M. H. Wallace, E. Hawk, G. B. Gordon, N. Wakabayashi, B. Saunders, Y. Shen, T. Fujimura, L. K. Su, B. Levin, L. Godio, S. Patterson, M. A. Rodriguez-Bigas, S. L. Jester, K. L. King, M. Schumacher, J. Abbruzzese, R. N. DuBois, W. N. Hittelman, S. Zimmerman, J. W. Sherman and G. Kelloff, N. Engl. J. Med., 2000, 342, 1946 CrossRef CAS PubMed.
- M. M. Bertagnolli, C. J. Eagle, A. G. Zauber, M. Redston, S. D. Solomon, K. Kim, J. Tang, R. B. Rosenstein, J. Wittes, D. Corle, T. M. Hess, G. M. Woloj, F. Boisserie, W. F. Anderson, J. L. Viner, D. Bagheri, J. Burn, D. C. Chung, T. Dewar, T. R. Foley, N. Hoffman, F. Macrae, R. E. Pruitt, J. R. Saltzman, B. Salzberg, T. Sylwestrowicz, G. B. Gordon and E. T. Hawk, N. Engl. J. Med., 2006, 355, 873 CrossRef CAS PubMed.
- L. Bernstein, D. Deapen, J. R. Cerhan, S. M. Schwartz, J. Liff, E. McGann-Maloney, J. A. Perlman and L. Ford, J. Natl. Cancer Inst., 1999, 91, 1654 CrossRef CAS PubMed.
- S. D. Solomon, J. J. V. McMurray, M. A. Pfeffer, J. Wittes, R. Fowler, P. Finn, W. F. Anderson, A. Zauber, E. Hawk and M. Bertagnolli, N. Engl. J. Med., 2005, 352, 1071 CrossRef CAS PubMed.
- V. Speirs, C. Malone, D. S. Walton, M. J. Kerin and S. L. Atkin, Cancer Res., 1999, 59, 5421 CAS.
- M. S. Glickman and C. L. Sawyers, Cell, 2012, 148, 1089 CrossRef CAS PubMed.
- E. A. Klein, I. M. Thompson, C. M. Tangen, J. J. Crowley, M. S. Lucia, P. J. Goodman, L. M. Minasian, L. G. Ford, H. L. Parnes, J. M. Gaziano, D. D. Karp, M. M. Lieber, P. J. Walther, L. Klotz, J. K. Parsons, J. L. Chin, A. K. Darke, S. M. Lippman, G. E. Goodman, F. L. Meyskens and L. H. Baker, JAMA, J. Am. Med. Assoc., 2011, 306, 1549 CrossRef CAS PubMed.
- R. Garcia, C. Gonzalez, A. Agudo and E. Riboli, Nutr. Cancer, 1999, 35, 212 CrossRef CAS PubMed.
- C. Arts, P. Holmann, H. Bueno de Mesquita, E. Feskens and D. Kromhout, Int. J. Cancer, 2001, 92, 298 CrossRef PubMed.
- S. S. Bansal, M. Goel, F. Aqil, M. V. Vadhanam and R. C. Gupta, Cancer Prev. Res., 2011, 4, 1158 CrossRef CAS PubMed.
- C. V. Rao, A. Rivenson, B. Simi and B. S. Reddy, Cancer Res., 1995, 55, 259 CAS.
- M. Nagpal and S. Sood, J. Nat. Sci., Biol. Med., 2013, 4, 3 CrossRef CAS PubMed.
- K. Nagahama, T. Kumano, N. Oyama and J. Kawakami, Biomater. Sci., 2015, 3, 1566 RSC.
- D. W. Liu and Z. W. Chen, J. Breast Cancer, 2013, 16, 133 CrossRef PubMed.
- A. Kumar, A. Ahuja, J. Ali and S. Baboota, Crit. Rev. Ther. Drug Carrier Syst., 2010, 27, 279 CrossRef CAS PubMed.
- W. Scarano, P. de Souza and M. H. Stenzel, Biomater. Sci., 2015, 3, 163 RSC.
- H. H. Tønnesen and J. Karlsen, Z. Lebensm.-Unters. Forsch., 1985, 180, 402 CrossRef.
- M. H. M. Leung, H. Colangelo and T. W. Kee, Langmuir, 2008, 24, 5672 CrossRef CAS PubMed.
- W. Wichitnithad, U. Nimmannit, P. S. Callery and P. Rojsitthisak, J. Pharm. Sci., 2011, 100, 5206 CrossRef CAS PubMed.
- M. P. Rayman, Lancet, 2000, 356, 233 CrossRef CAS.
- M. P. Rayman, Proc. Nutr. Soc., 2005, 64, 527 CrossRef CAS PubMed.
- H. W. Zeng and G. F. Combs, J. Nutr. Biochem., 2008, 19, 1 CrossRef CAS PubMed.
- J. X. Lu and C. Jiang, Antioxid. Redox Signaling, 2005, 7, 15 Search PubMed.
- M. H. Yazdi, M. Mahdavi, E. Kheradmand and A. R. Shahverdi, Arzneim.–Forsch./Drug Res., 2012, 62, 525 CrossRef CAS PubMed.
- E. A. Klein, J. Urol., 2004, 171, S50 CrossRef CAS PubMed.
- P. D. Whanger, Br. J. Nutr., 2004, 91, 11 CrossRef CAS PubMed.
- F. Meyer, P. Galan, P. Douville, I. Bairati, P. Kegle, S. Bertrais, C. Estaquio and S. Hercberg, Int. J. Cancer, 2005, 116, 182 CrossRef CAS PubMed.
- A. F. S. Amaral, K. P. Cantor, D. T. Silverman and N. Malats, Cancer Epidemiol., Biomarkers Prev., 2010, 19, 2407 CrossRef CAS PubMed.
- G. F. Combs, J. Nutr., 2005, 135, 343 CAS.
- Y. C. Chen, K. S. Prabhu and A. M. Mastro, Nutrients, 2013, 5, 1149 CrossRef CAS PubMed.
- H. W. Zeng, W. H. Cheng and L. K. Johnson, J. Nutr. Biochem., 2013, 24, 776 CrossRef CAS PubMed.
- R. Brigelius-Flohe, Chem. Biodiversity, 2008, 5, 389 CAS.
- S. S. Cao, A. Durrani and Y. M. Rustum, Clin. Cancer
Res., 2004, 10, 2561 CrossRef CAS PubMed.
- Z. S. Li, L. Carrier, A. Belame, A. Thiyagarajah, V. A. Salvo, M. E. Burow and B. G. Rowan, Breast Cancer Res. Treat., 2009, 118, 33 CrossRef CAS PubMed.
- D. Umysova, M. Vitova, I. Douskova, K. Bisova, M. Hlavova, M. Cizkova, J. Machat, J. Doucha and V. Zachleder, BMC Plant Biol., 2009, 9, 58 CrossRef PubMed.
- A. Tarze, M. Dauplais, I. Grigoras, M. Lazard, N. T. Ha-Duong, F. Barbier, S. Blanquet and P. Plateau, J. Biol. Chem., 2007, 282, 8759 CrossRef CAS PubMed.
- B. J. Wycherly, M. A. Moak and M. J. Christensen, Nutr. Cancer, 2004, 48, 78 CrossRef CAS PubMed.
- J. S. Zhang, H. L. Wang, X. X. Yan and L. D. Zhang, Life Sci., 2005, 76, 1099 CrossRef CAS PubMed.
- J. S. Zhang, X. F. Wang and T. W. Xu, Toxicol. Sci., 2008, 101, 22 CrossRef CAS PubMed.
- H. L. Wang, J. S. Zhang and H. Q. Yu, Free Radical Biol. Med., 2007, 42, 1524 CrossRef CAS PubMed.
- M. Panahi-Kalamuei, M. Mousavi-Kamazani, M. Salavati-Niasari and S. M. Hosseinpour-Mashkani, Ultrason. Sonochem., 2015, 23, 246 CrossRef CAS PubMed.
- M. Panahi-Kalamuei, M. Salavati-Niasari and S. M. Hosseinpour-Mashkani, J. Alloys Compd., 2014, 617, 627 CrossRef CAS.
- M. Panahi-Kalamuei, M. Salavati-Niasari, Z. Zarghami, M. Mousavi-Kamazani, H. Taqriri and A. Mohsenikia, J. Mater. Sci.: Mater. Electron., 2015, 26, 2851 CrossRef CAS.
- K. K. Vekariya, J. Kaur and K. Tikoo, Nanomedicine, 2012, 8, 1125 CAS.
- Y. J. Wang, M. H. Pan, A. L. Cheng, L. I. Lin, Y. S. Ho, C. Y. Hsieh and J. K. Lin, J. Pharm. Biomed. Anal., 1997, 15, 1867 CrossRef CAS PubMed.
- R. Jagannathan, P. M. Abraham and P. Poddar, J. Phys. Chem. B, 2012, 116, 14533 CrossRef CAS PubMed.
- A. Kunwar, A. Barik, R. Pandey and K. I. Priyadarsini, Biochim. Biophys. Acta, Gen. Subj., 2006, 1760, 1513 CrossRef CAS PubMed.
- Y. X. Feng, J. Y. Su, Z. N. Zhao, W. J. Zheng, H. L. Wu, Y. B. Zhang and T. F. Chen, Dalton Trans., 2014, 43, 1854 RSC.
- W. Liu, X. L. Li, Y. S. Wong, W. J. Zheng, Y. B. Zhang, W. Q. Cao and T. F. Chen, ACS Nano, 2012, 6, 6578 CrossRef CAS PubMed.
- S. Kundu and U. Nithiyanantham, RSC Adv., 2013, 3, 25278 RSC.
- D. K. Singh, R. Jagannathan, P. Khandelwal, P. M. Abraham and P. Poddar, Nanoscale, 2013, 5, 1882 RSC.
- P. K. Mohan, G. Sreelakshmi, C. V. Muraleedharan and R. Joseph, Vib. Spectrosc., 2012, 62, 77 CrossRef CAS.
- H. Souguir, F. Salaün, P. Douillet, I. Vroman and S. Chatterjee, Chem. Eng. J., 2013, 221, 133 CrossRef CAS.
- M. Magro, R. Campos, D. Baratella, G. Lima, K. Holà, C. Divoky, R. Stollberger, O. Malina, C. Aparicio, G. Zoppellaro, R. Zbořil and F. Vianello, Chem. - Eur. J., 2014, 20, 1191 CrossRef PubMed.
- Y. Sun, Free Radical Biol. Med., 1990, 8, 583 CrossRef CAS PubMed.
- F. Boess, M. Kamber, S. Romer, R. Gasser, D. Muller, S. Albertini and L. Suter, Toxicol. Sci., 2003, 73, 386 CrossRef CAS PubMed.
- C. Syng-ai, A. L. Kumari and A. Khar, Mol. Cancer Ther., 2004, 3, 1101 CAS.
- B. D. Chithrani, A. A. Ghazani and W. C. W. Chan, Nano Lett., 2006, 6, 662 CrossRef CAS PubMed.
- I. M. Ghobrial, T. E. Witzig and A. A. Adjei, Ca-Cancer J. Clin., 2005, 55, 178 CrossRef PubMed.
- S. Y. Sun, N. Hail and R. Lotan, J. Natl. Cancer Inst., 2004, 96, 662 CrossRef CAS PubMed.
- R. Sinha and K. El-Bayoumy, Curr. Cancer Drug Targets, 2004, 4, 13 CrossRef CAS PubMed.
- F. Wang, Y. C. Wang, S. Dou, M. H. Xiong, T. M. Sun and J. Wang, ACS Nano, 2011, 5, 3679 CrossRef CAS PubMed.
- R. J. Tallarida, J. Pharmacol. Exp. Ther., 2001, 298, 865 CAS.
- S. X. Yu, W. T. Zhang, W. Liu, W. X. Zhu, R. C. Guo, Y. S. Wang, D. H. Zhang and J. L. Wang, Nanotechnology, 2015, 26, 145703 CrossRef PubMed.
- T. F. Chen, Y. S. Wong and W. J. Zheng, Phytochemistry, 2006, 67, 2424 CrossRef CAS PubMed.
- J. A. Kim, H. J. Lee, H. J. Kang and T. H. Park, Biomed. Microdevices, 2009, 11, 287 CrossRef PubMed.
- A. Sasidharan, L. S. Panchakarla, P. Chandran, D. Menon, S. Nair, C. N. R. Rao and M. Koyakutty, Nanoscale, 2011, 3, 2461 RSC.
- L. Chen, R. A. Yokel, B. Hennig and M. Toborek, Journal of NeuroImmune Pharmacology, 2008, 3, 286 CrossRef PubMed.
Footnote |
† Electronic supplementary information (ESI) available: In vitro ionization and stability of Cur in an alkaline aqueous solution (pH = 8.5), quantification of the content of Cur loaded on the surface of SeNPs@Cur, magnifying TEM images of HepG2 cells showing uptake of SeNPs and SeNPs@Cur, free radical scavenging activities of SeNPs and Cur at higher concentrations, and Cur-induced activation of caspases and production of intracellular ROS. See DOI: 10.1039/c6ra13291j |
|
This journal is © The Royal Society of Chemistry 2016 |