DOI:
10.1039/C4RA01047G
(Paper)
RSC Adv., 2014,
4, 27213-27223
Cyto and genotoxicities of graphene oxide and reduced graphene oxide sheets on spermatozoa†
Received
6th February 2014
, Accepted 28th May 2014
First published on 29th May 2014
Abstract
Concentration-dependent cyto and genotoxicities of graphene oxide (GO) and reduced GO (rGO) sheets on spermatozoa were studied. rGO sheets with various surface chemical states were achieved using hydrazine (N2H4) hydrothermal (HT) reactions and green tea polyphenols (GTPs). Although 0.1 μg mL−1 graphene could not change sperm viability and kinetic parameters, <40% and 20% of spermatozoa were viable and progressively motile, after 2 h incubation with 400 μg mL−1 GO or rGO, respectively. All the graphene nanomaterials induced concentration-dependent reductions of adenosine triphosphate and NAD+/NADH produced by spermatozoa for motility and metabolic activity. While GO, N2H4–rGO, and HT-rGO sheets caused increasing reactive oxygen species and sperm nitric oxide production, GO sheets reduced by antioxidant GTPs decreased them. Hence, physical trapping of spermatozoa by graphene (particularly GTP–rGO) is one of the important mechanisms describing the cytotoxicity, in addition to the other reactions, resulting in the inactivation and/or death of spermatozoa. Graphene genotoxicity was initiated by 1.0 μg mL−1 of N2H4–rGO and HT-rGO and 10 μg mL−1 of GO and GTP–rGO sheets. The extremely sharp edge and/or high mobility of N2H4–rGO provided easy penetration of the sheets into spermatozoa to interact with cell nuclei. In contrast, the steric effect induced by GTPs attached on rGO caused a lower genotoxicity.
1. Introduction
The fascinating and promising properties of graphene (as a two-dimensional sheet of graphitized carbon atoms) have opened up numerous novel scientific1–4 and technological5–10 areas. Recently, because of growing demands for the production as well as application of graphene, particularly in biological and medical fields (see, for example, ref. 11), the potential toxic effects of graphene on cells and microorganisms have attracted much attention.
Concentration-dependent cytotoxicity of graphene in human erythrocytes and skin fibroblasts was reported by Liao et al.12 Furthermore, concentration- and time-dependent cytotoxicity of graphene on neural pheochromocytoma-derived PC12,13 A549,14 and human mesenchymal stem cells15 through the generation of reactive oxygen species (ROS) was studied. Damages to cell membrane through direct contact of cells with the ultra sharp edges of graphene sheets is also proposed as one of the main mechanisms which are responsible for cytotoxicity of graphene sheets.16,17 Furthermore, trapping microorganisms within aggregating reduced graphene sheets in a suspension is suggested as another mechanism describing cytotoxicity of graphene sheets.18 Very recently, our group demonstrated that graphene nanoplatelets and nanoribbons can exhibit genotoxic effects on human stem cells in addition to their cytotoxic effects.16,19 There are also a few recent reports about the effects of graphene on animals. For instance, Chen et al.20 showed slight delay in the hatching of zebrafish embryos at a high concentration of graphene (50 μg mL−1), although it did not result in significant increase of apoptosis in the embryo. Recently, blood circulation and biodistribution of graphene nanosheets (sheets with nanometer lateral dimensions) in tumor and normal organs of mice were also investigated.21,22
In recent years, graphene sheets have been widely utilized in cancer cell imaging, targeting and therapy,22–26 tissue engineering,27–30 and neural regeneration (as scaffolds to support cellular attachment, proliferation and differentiation),31–33 and drug delivery (as easy functionalizable transporters).34–36 For example, graphene nanoplatelets (sheets with nanometer lateral dimensions) were applied as high NIR absorbent in an in vivo ultra-low laser power cancer therapy22,23 and as vehicles for the delivery of water-insoluble cancer drugs.38 Despite such promising in vivo capabilities of graphene in upcoming nanotechnology-based medicine, no systematic investigation about the side effects of graphene on human health has been reported yet.
The graphene nanosheets distributed in a body may pass through biological membranes such as cell membranes, blood–brain barrier, and blood–testis barrier, and then affect the physiology of cells in the body, as previously observed for nanoparticles (see, for example, ref. 37). Concerning this, it was found that after intravenous injection of nanoparticles38 or graphene nanosheets,22,23 they were present in various organs including lung, blood, testis, spleen, kidney, thymus, heart, liver and brain. Hence, cytotoxic effects of the graphene might interfere in spermatogenesis (as one of its side effects) through influence on spermatozoa in testis. In fact, epigenetic alterations induced by nanoparticles were previously observed in germ cells.39,40 It was suggested that ROS generated by the nanoparticles can damage spermatozoa through DNA and/or sperm membrane damages which the both latter resulted in reduction of sperm motility.41 It was also reported that ROS can decrease the available energy of spermatozoa through damaging mitochondria.42 However, there has been no investigation concerning the impact of graphene on spermatozoa.
In this study, concentration-dependent cyto and genotoxic effects of GO sheets, and GO sheets reduced by hydrazine (N2H4), hydrothermal (HT) reaction, and green tea polyphenols (GTPs) on spermatozoa were investigated. The cytotoxic effects of the graphene nanomaterials with various concentrations (0.1 to 400 μg mL−1) were studied by the evaluation of viability, motility, and progressive motility of spermatozoa. Other kinetic parameters of motile spermatozoa exposed to the graphene nanomaterials were also evaluated to present a more detailed kinetic study. The concentration-dependent cytotoxicity of graphene on motility and metabolic activity of spermatozoa were examined by measuring adenosine triphosphate (ATP) and NAD+/NADH produced by spermatozoa. The effects of the graphene nanomaterials on the generation of ROS and nitric oxide (NO) were investigated to better understand the more effective mechanisms describing the cytotoxicity. Genotoxicity of the GO and rGO sheets was evaluated by monitoring DNA fragmentation of the spermatozoa. In addition, the threshold concentrations for the appearance of the genotoxic properties of the GO and rGO sheets was determined.
2. Experimental
2.1. Synthesis of GO
Natural graphite powder (particle diameter ≤40 μm, Sigma Aldrich) was applied to synthesize graphite oxide suspension by a modified Hummers' method.43,44 In a typical procedure, 0.5 g graphite powder was mixed by 20 mL H2SO4 at 80 °C for 5 h. Then, 0.5 g NaNO3 was added into the mixture and stirred in an ice bath for 10 min. After that, 3.0 g KMnO4 was slowly added into the mixture and stirred for 2 h. Then it was warmed up to room temperature, while stirred continuously in a water bath at 35 °C for 1 h. The prepared suspension was diluted by 40 mL deionized (DI) water. During diluting, temperature of the suspension was maintained at <60 °C. Finally, 3 mL H2O2 (30%) diluted by 100 mL DI water was added into the suspension in order to reduce the residual permanganate to soluble manganese ions to stop the gas evolution of the suspension. The residual acids and salts of the graphite oxide suspension were removed by filtering through an anodic membrane filter (47 mm in diameter, 0.2 μm pore size, Whatman). The filtered graphite oxide powder was dispersed in DI water to obtain an aqueous graphite oxide suspension with yellow-brownish color. Then, the aqueous suspension was centrifuged by an Eppendorf 5702 centrifuge with a rotor radius of 10 cm at 2000 rpm for 15 min and 8000 rpm for 20 min to remove any unexfoliated graphitic plates and tiny graphite particles, respectively. Finally, GO suspension was achieved by ultrasonication of the centrifuged graphite oxide suspension at frequency of 40 kHz and power of 100 W for 30 min.
It is noteworthy that our survey X-ray photoelectron spectroscopy showed no trace related to heavy metal impurities (which can act as toxic agents) in the GO samples, as expected from the high pure (>99.99%) graphite prepared from Sigma Aldrich. Moreover, recently, we found that even if one uses carbonaceous wastes having heavy metal impurities (e.g., newspaper, which contains Pb) for graphitization and then synthesis of GO, low concentrations (<5 at%) of heavy metal impurities remained in the GO synthesized by the Hummers' method.45
2.2. Reduction of GO by hydrazine
For chemical reduction of GO aqueous suspension by hydrazine, 100 mL GO suspension with a concentration of 0.5 mg mL−1 was used. At first, pH of the suspension was adjusted ∼9.0 by adding a diluted ammonia solution. After that, 100 μL of hydrazine solution (35%) was added to the suspension while it was stirring. Then, the suspension was refluxed at 90 °C for 3 h in an oil bath. Finally, the prepared N2H4–rGO suspension was centrifuged (at 8000 rpm for 5 min) and filtered (to remove the residual ammonia and hydrazine) and resuspended in DI water for the next stages.
2.3. Reduction of GO by a hydrothermal method
To reduce the GO suspensions by a hydrothermal method, at first, pH of the suspension was adjusted ∼9.0 by a diluted ammonia solution. Then, 100 mL of the GO suspension (0.5 mg mL−1) was inserted in a hydrothermal autoclave reactor containing a 150 mL Teflon chamber. The hydrothermal reduction occurred at 120 °C for 5 h. Finally, the prepared HT-rGO suspension was centrifuged (at 8000 rpm for 5 min) and filtered (to remove the oxygen dissolved in the water) and resuspended in DI water for the next stages.
2.4. Reduction of GO by GTP
To extract the polyphenol components of the tea, 10 g GT (produced by Shahsavand Tea Co. Mashhad, Iran) were infused in 100 mL DI water at temperature of 80–86 °C for 10 min. Then, the extracted suspension was filtered twice through a 0.44 μm cellulose membrane to achieve a clear solution of the infused GT with a light brownish color. To reduce the GO suspension (with concentration of 0.5 mg mL−1), the GT solution was added (with equal volume) into the GO suspension, at room temperature. Using a magneto-stirrer heater, the prepared GO–GT suspensions were stirred at 400 rpm while heated at 80 °C in the presence of a Fe foil (with dimensions of 10 mm × 10 mm × 1 mm) in air for 10 min. Finally, the prepared GTP–rGO suspension was centrifuged (at 8000 rpm for 5 min) and filtered (to remove the residual GTPs) and resuspended in DI water for the next stages. Some detailed results about this method can be found elsewhere.46
2.5. Material characterization
Surface topography and height profile of the graphene sheets were investigated by atomic force microscopy (AFM, Digital Instruments NanoScope V) in tapping mode. The AFM samples were prepared by drop-casting a diluted suspension (0.01 mg mL−1) onto a cleaned Si(100) substrate. XPS was applied to study the chemical state variations of the GO sheets under the various reducing conditions. The data were achieved by using a hemispherical analyzer supplied by an Al Kα X-ray source (hν = 1486.6 eV) operating at a vacuum better than 10−7 Pa. For quantitative analyses, the XPS peaks were deconvoluted by using Gaussian components after a Shirley background subtraction. Also, quantitative elemental compositions were evaluated using peak area ratio of the XPS core levels and the sensitivity factor of each element in XPS. Raman spectroscopy was carried out at room temperature using a HR-800 Jobin-Yvon with 532 nm Nd-YAG excitation source to investigate about the carbon structure of the samples. The samples for XPS and Raman spectroscopy were prepared by casting conc. graphene suspension onto the substrate and removing the solvent through drying at 200 °C for 30 min. Zeta potentials of the GO and rGO samples were evaluated by using a Zetasizer (Malvern Instruments).
2.6. Animals
Male BalbC mice aged 8 weeks were maintained at 20 ± 2 °C on a 12
:
12 h light/dark cycle with ad libitum access to food and water. All animal procedures were performed based on the guideline of the AVMA Guidelines for the Euthanasia of Animals. All experiments were performed in compliance with the relevant laws and institutional guidelines (approved by National Research Center for Transgenic Mouse and the Research Council of Sharif University of Technology).
2.7. Sperm preparation and treatment
The mice were sacrificed by cervical dislocation and then cauda epididymides were removed and carefully dissected in Human Tubal Fluid (HTF) medium supplemented by 10% fetal bovine serum (FBS). Spermatozoa were then obtained from swim-up cauda epididymides as described previously.47 Counting the spermatozoa was performed and 1 × 106 spermatozoa were incubated with a desired graphene suspension (the GO or the rGO suspension with various GO concentrations of 400, 100, 10, 1, and 0.1 μg mL−1) in a 5% CO2 atmosphere at 37 °C for 2 h. The graphene-treated spermatozoa were used for further following experiments.
2.8. Sperm motility assessment
The GO- and rGO-treated sperm motility parameters were analyzed by computer-assisted sperm motility analysis software (CASA, version 12; Hamilton Thorne Research, Beverly, MA). The average of kinetic parameters including total sperm motility, progressive motility, straight-line velocity (VSL), curvilinear velocity (VCL), amplitude of lateral head (ALH), and beat cross frequency (BCF) were evaluated only for motile spermatozoa. Images are captured by a video camera (Basler Vision, A312FC at 50 fps; Tecnologie Co., Ahrensburg, Germany) at 100× magnification.
2.9. ATP level measurement
In this stage, the GO and rGO-treated spermatozoa were centrifuged at 2000 rpm for 10 min. The pellets were resuspended in a lysis buffer containing 100 mM Tris–HCl and 4 mM EDTA at pH 7.8 and boiled at 95 °C for 5 min. Then, the samples were centrifuged at 2000 rpm for 15 min and aliquots of the supernatants were analyzed in duplicate using an ATP bioluminescence assay kit CLS II (Roche Applied Science).
2.10. ROS level measurement
The ROS level was measured by a fluorimetric assay using 2′,7′-dichlorofluorescin diacetate (DCFH-DA; Sigma). The treated spermatozoa were incubated with DCFH-DA (10 mmol) for 30–60 min at 37 °C and centrifuged at 400 rpm. Then, the cells were washed three times with PBS. The conversion of DCFH to dichlorofluorescin (DCF) was measured using a fluorescence spectrophotometer with excitation at 480 nm and emission at 520 nm.
2.11. Sperm nitric oxide production
The GO- and rGO-treated spermatozoa were centrifuged at 1000 rpm for 10 min. The sperm NO production was measured by assessing the levels of nitrate (NO3) and nitrite (NO2) in supernatant using Griess reagent (Promega) colorimetric method. The NO2 level was determined at 540 nm after the addition of Griess reagent. Finally, the concentration of NO2 was normalized to the initial number of sperm cells (1 × 106 cells).
2.12. NAD+/NADH level measurement
NAD+/NADH level was assayed using NAD+/NADH quantification kit (Bio Vision). Total NAD+ level was measured according to the kit manufacturer's instruction. To evaluate NADH, at first, NAD+ was decomposed by heating at 60 °C for 30 min and then transferred to a microplate. After adding 10 mL NADH developer to each plate, optical density was measured at 450 nm every 30 min for 5 h.
2.13. Comet assay
Comet assay was utilized to monitor DNA damages in the spermatozoa treated with the GO and rGO nanomaterials, based on the protocol described by Tice et al.48 with some modifications. Slides were prepared by dipping frosted slides in a molten regular agarose and dried at 50 °C. The coated slides were immersed in a lysing solution containing 2.5 M NaCl, 100 mM EDTA, 10 mM Tris, 1% Triton X-100 and 10 mM DL-dithiothreitol for 2 h and then placed on a horizontal electrophoretic unit. The slides were left in an electrophoresis buffer containing 500 mM NaCl, 100 mM Tris, 1 mM EDTA and 0.2% DMSO for 20 min, and then electrophoresed at 10 V and 250 mA for 60 min. After electrophoresis, the slides were washed with phosphate buffer saline (PBS) and dipped in a neutralizing solution containing 50% ethanol, 20 mM Tris and 1 mg mL−1 spermine for 5 min. This latter step was repeated three times with fresh PBS. After that, the slides were stained with ethidium bromide (20 μg mL−1), washed with distilled water and mounted with cover slips. The spermatozoa were monitored at 400× magnification using a fluorescent microscope (Nikon), and then 50 spermatozoa (from totally 100 spermatozoa) were randomly analyzed in each slide. The results showed a percentage of DNA fragmentation on each slide.
All the biological tests were repeated at least three times. To statistically analyze the differences between the results of graphene and control samples, Wilcoxon test was applied. The differences were considered significant for the P-values < 0.05.
3. Results and discussion
Fig. 1A shows typical AFM image of the GO sheets deposited on the Si substrate. The overlapped sheets are clearly distinguishable in the images. The height profile distribution of the sheets is also presented in Fig. 1B. It is well-known that typical thickness of monolayer (ML) GO sheets is ∼0.8 nm, i.e., ∼0.44 nm thicker than the thickness of graphene (∼0.36 nm) because of the bonding of the oxygen functional groups on either side of the graphene surface.49–51 Since the first peak in the height profile distribution indicates roughness fluctuations of the film surface, the position of the second peak at ∼0.8 nm can be attributed to thickness of 1 ML sheets deposited on the substrate. The position of the third peak at ∼1.5 nm implied the presence of 2 ML GO sheets on the substrate (but with a lower frequency as compared to that of the 1 ML sheets). The presence of a relatively weak peak at ∼2.2 nm indicated that abundance of the sheets with thicknesses ≥3 MLs was negligible. Although the reduction of the GO sheets caused nearly no significant change in the surface topography of the sheets, thickness of the monolayer sheets changed to ∼0.4 after reduction by N2H4 as well as HT method and ∼1.4 nm after reduction by GTPs. The increase in the thicknesses of monolayer GTP–rGO sheets can be assigned to the attachment of the GTP molecules on both sides of the rGO sheets, resulting in ∼1 nm increase in the thickness of the reduced sheets, as demonstrated for aromatic molecules by Su et al.52 and previously observed for GO sheets reduced by melatonin,53 vitamin C,54 GTPs,49 and ginseng.55
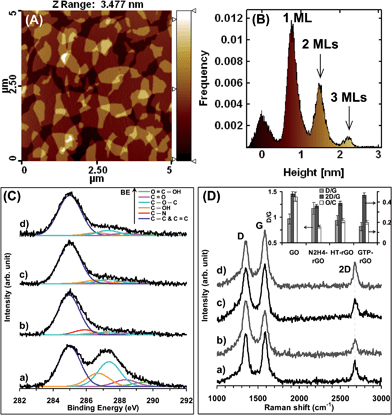 |
| Fig. 1 (A) AFM image of GO sheets, (B) height profile distribution of the GO sheets shown in (A) with 1–3 monolayer (ML) structures, (C) XPS and (D) Raman spectra of (a) GO, (b) N2H4–rGO, (c) HT-rGO, and (d) GTP–rGO sheets. The inset of (D) shows D/G and 2D/G intensity ratios (obtained through the Raman spectra) and O/C atomic ratio (obtained through XPS). | |
Fig. 1C shows XPS peak deconvolution of C(1s) core levels of the GO, N2H4–rGO, HT-rGO and GTP–rGO sheets. In the peak deconvolution, the peak fixed at 285.0 eV was assigned to the C–C and C
C bonds. The other deconvoluted peaks located at the binding energies of 286.7, 287.3, 288.3 and 289.4 eV were attributed to the C–OH, C–O–C, C
O, and O
C–OH oxygen-containing functional groups, respectively (see, for example, ref. 56 and 57). Fig. 1C indicates that the reduction efficacies obtained through HT method or by using GTPs (as biocompatible antioxidants) are comparable with the deoxygenation obtained by N2H4 (as a strong but toxic chemical reductant). It should be noted that the C(1s) core level of the samples reduced by hydrazine showed another peak component at 285.9 eV, which can be assigned to the formation of C–N bonds on the surface of the N2H4–rGO sheets.56 No peak relating to formation of C–N bonds was found in the XPS of the GO reduced through HT method and/or by GTPs. To obtain a quantitative analysis on the chemical state variations of the GO sheets at the different reduction conditions, the O/C atomic ratio of the samples was evaluated and presented in the inset of Fig. 1D.
Raman spectroscopy was applied to investigate the carbon structure of the GO sheets and the reduced ones. Fig. 1D shows increase in the intensity of the D band (∼1350 cm−1) and decrease in the that of the G band (∼1585 cm−1) after the reduction of the GO sheets by hydrazine. The increase in the D/G intensity ratio of the N2H4–rGO sheets (from 0.96 for the GO to 1.17) can be attributed to decreasing the graphitic domain size and/or increasing the defects, due to formation of the C–N bonds (consistent with the results of XPS analysis). On the other hand, the D/G ratio of the GO sheets decreased after reduction through HT method or by GTPs. The decrease of the ratio, especially for the GTP–rGO sheets, can be attributed to recovering the graphitic structure of the GO sheets during the chemical reduction and/or through π–π stacking of GTP molecules on the reduced sheets.
Raman spectroscopy can also be used to distinguish the single- and multi-layer properties of graphene sheets, because its 2D band is highly sensitive to stacking of the sheets.58 For example, single-layer graphene sheets present a 2D band at 2679 cm−1, while the 2D band of multi-layer graphene sheets (including 2–4 layers) appears as a broadened peak with 19 cm−1 shift into higher wavenumbers.59 Furthermore, the typical 2D/G intensity ratios of single-, double-, triple- and multi- (>4) layer graphene sheets are >1.6, ∼0.8, ∼0.30 and ∼0.07, respectively (see, e.g., ref. 60–62). The 2D/G intensity ratios of our samples are shown in the inset of Fig. 1D. In this work, not only all graphene samples showed 2D bands at ∼2680 cm−1, but also the 2D/G ratio changed slightly from 0.48 (for the GO sheets) to 0.36 (for the N2H4–rGO sheets), suggesting the presence of graphene sheets with ≤3 MLs in our samples (consistent with the results of AFM analysis).
The surface charge of nanomaterials plays an important role in cytotoxicity and cell–nanomaterial interactions because cell membranes themselves are usually charged.63 Hence, before cytotoxicity experiments, characterization of the surface charge of the graphene sheets is necessary, especially in biological media. Concerning this, Table 1 shows the zeta potentials of all GO and rGO sheets used in this work. It was found that the zeta potential of all the GO and rGO sheets were negative, independent from the medium of dispersion. The higher zeta potential of the GO sheets than the rGO ones can be assigned to the oxygen content of the former. Consistently, the lowest zeta potential of the N2H4–rGO can be attributed to its decreased oxygen amount, as found by its XPS analysis and poor aqueous dispersity.
Table 1 Zeta potentials of GO and rGO sheets with concentration of 50 μg mL−1 in DI water and HTF
Sample |
Zeta potential (mV) |
In DI water |
In HTF |
GO |
−41.2 ± 3.1 |
−23.5 ± 1.2 |
N2H4–rGO |
−20.9 ± 1.7 |
−9.7 ± 0.8 |
HT-rGO |
−36.4 ± 2.1 |
−17.6 ± 1.3 |
GTP–rGO |
−27.1 ± 2.5 |
−14.8 ± 1.7 |
Since all of the GO and rGO sheets exhibited negative surface charges, they would present the same electrostatic (i.e., attractive) interactions with the cell membranes. Furthermore, the different values of zeta potential of the samples can induce various cytotoxic effects, due to the aggregation of the charged sheets. In fact, it was found that the GO sheets with the highest zeta potential exhibited the highest dispersity in HTF up to 3 h (the electrostatic stabilization). Although the GO sheets settled out in HTF after 24 h (due to charge neutralization of oxygen groups of GO sheets by ionic salt species of the medium), this aggregation was reversible by simply an external agitation. In contrast, the N2H4–rGO sheets with the lowest zeta potential showed a fast (within 3 h) and irreversible aggregation in HTF, due to more π–π stacking interactions between the deoxygenated surfaces. The irreversible aggregation of the rGO sheets in the culture media containing the spermatozoa can induce further cytotoxicity, due to trapping the spermatozoa by the aggregated sheets, as studied in the following.
Microscopic images of the untreated spermatozoa (the spermatozoa in the absence of any graphene), the spermatozoa exposed to GO sheets with concentrations of 10 and 400 μg mL−1, and N2H4–rGO with concentration of 400 μg mL−1 are shown in Fig. 2. The clusters of aggregated graphene sheets are distinguishable in Fig. 2a and c. Although the motile and immotile spermatozoa can be easily observed by the movies prepared from them (see the ESI†), the arrows in the dashed-line squares and circles show typical motile and immotile spermatozoa, respectively. It is seen that the motile and immotile spermatozoa were trapped by aggregated graphene sheets. Nearly all of the other spermatozoa, particularly the spermatozoa presented in Fig. 2d, are progressive motile ones. Therefore, inactivation of spermatozoa through the transition of the progressive motile ones into motile (but not progressive), immotile and finally dead ones can strongly depend on the concentration and chemical state of graphene sheets, as discussed in more details in the following.
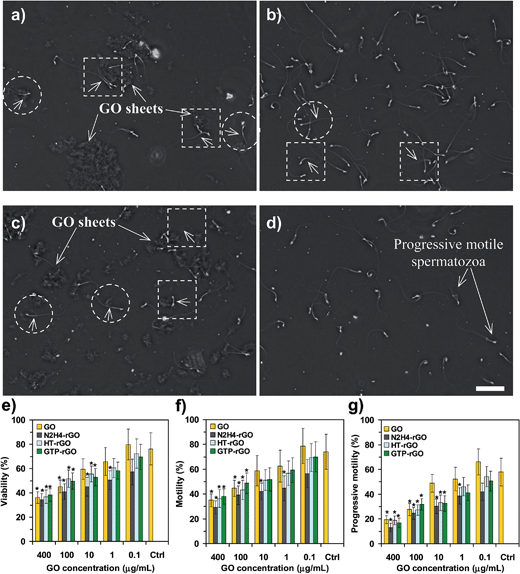 |
| Fig. 2 Microscopic images of spermatozoa exposed to graphene sheets: (a) GO (400 μg mL−1), (b) GO (10 μg mL−1), and (c) N2H4–rGO (400 μg mL−1), as compared to (d) the normal state of spermatozoa in the absence of any graphene sheets (control group). The clusters of graphene sheets are distinguishable in (a) and (c). The arrows in the dashed-line squares and circles indicate typical motile and immotile spermatozoa, respectively. Nearly all the other spermatozoa, especially the ones shown in (d), are progressive motile ones. The scale bar shows 50 μm. (e) Viability, (f) motility, and (g) progressive motility of spermatozoa after 2 h incubation with GO, N2H4–rGO, HT-rGO, and GTP–rGO with various GO concentrations as compared to the control sample. Significant results are marked by asterisks (*) for P-values < 0.05 (n = 3). | |
Fig. 2e–g show viability, motility, and progressive motility of the spermatozoa after 2 h incubation with the GO, N2H4–rGO, HT-rGO, and GTP–rGO sheets with various GO concentrations as compared to the control sample (the medium containing no graphene), respectively. It is seen that the percent of viable, motile, and progressive motile spermatozoa decreased by increasing the concentration of graphene materials (independent from the chemical states of GO and rGO sheets). Although the GO, HT-rGO, and GTP–rGO sheets showed no significant effects on viability, motility, and progressive motility of the spermatozoa at the lowest concentration testes (i.e., 0.1 μg mL−1, as compared to the control sample), the N2H4–rGO sheets presented some effects even at such concentration. For the concentrations ≤10 μg mL−1, the inactivation effects of the GO sheets on the spermatozoa are significantly lower than the effects of the rGO sheets. By increasing the graphene concentration to 400 μg mL−1, nearly all of the graphene samples (including the GO and rGO sheets) showed similar and significant inactivation effects on the spermatozoa. In fact, at the highest concentration tested, <40% of the spermatozoa were viable as well as motile and only <20% of the spermatozoa were progressively motile after a 2 h incubation with the graphene materials. This means that some of the viable and motile spermatozoa did not exhibit progressive motility due to their physical trapping by the graphene sheets.
It is worth noting that in a preliminary in vivo study, we found that an intravenous injection of 200 μL of the graphene suspensions with initial concentration of 400 μg mL−1 (corresponding to ∼1 mg graphene per kilogram of the mice body weight) resulted in toxic effects which were comparable with the effects observed for the in vitro toxicity of graphene with concentration of ∼10 μg mL−1. This means that although the toxicity of in vivo tests was substantially lower than the in vitro ones (due to in vivo protein corona18 and/or dilution23,25 effects), the in vivo toxicity can still be significant, especially at high graphene concentrations (≥100 μg mL−1) and/or high volume injections (≥100 μL).
Since the suitable performance of spermatozoa strongly depends on their mobility, some other kinetic parameters such as VSL, VCL, ALH, and BCF were studied in some more details, as shown in Fig. 3. It is seen that the values assigned to all the kinetic parameters decreased by increasing the concentration of graphene materials, but the rGO sheets could further limit the parameters as compared with the GO ones. Fig. 3a presents that the interaction of graphene nanomaterials (especially the reduced ones) with spermatozoa significantly decreased the VSL of the motile spermatozoa, even at the lowest concentration tested (0.1 μg mL−1). VSL of the motile spermatozoa in the presence of the rGO sheets at the highest concentration tested (400 μg mL−1) decreased one order of magnitude relative to the control sample (Fig. 3a), consistent with the results obtained by the progressive motility (Fig. 2g). Fig. 3b and c show that reduction observed for VCL and ALH of the motile spermatozoa was not as strong as observed for VSL. Therefore, physical trapping of the spermatozoa by the graphene nanomaterials can be considered as one of the main mechanisms describing the cytotoxicity of the graphene sheets (in addition to the mechanisms relating to death of the spermatozoa). Consistently, Fig. 3a shows that VSL of the motile spermatozoa is nearly negligible at 400 μg mL−1, while based on Fig. 3d, the motile spermatozoa with non-vanishing BCF are still present at such concentration (although the BCF decreased ∼63% relative to the control sample for the rGO sheets). The existence of unprogressive but vibrating spermatozoa at the highest concentration tested (400 μg mL−1) further confirmed the trapping of spermatozoa by the graphene sheets as one of the effective inactivation mechanisms.
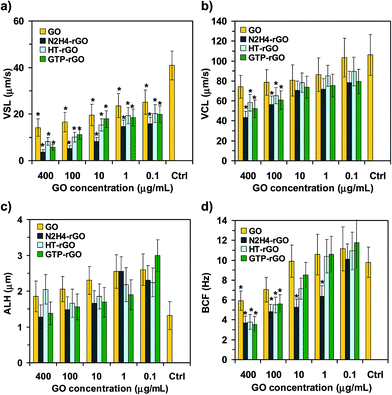 |
| Fig. 3 (a) VSL, (b) VCL, (c) ALH, and (d) BCF of spermatozoa after incubation with GO, N2H4–rGO, HT-rGO, and GTP–rGO with various concentrations as compared to the control sample. Significant results are marked by asterisks (*) for P-values < 0.05 (n = 3). | |
High levels of ATP are required for motility of spermatozoa.50 Hence, we measured the ATP levels of the spermatozoa incubated with the GO, N2H4–rGO, HT-rGO, and GTP–rGO sheets at various concentrations as compared to the ATP level of the spermatozoa in media containing no graphene (control sample), as shown in Fig. 4a. It was found that the ATP level of the spermatozoa decreased by increasing the concentration of graphene nanomaterials (once again, independent from the chemical states of GO and rGO sheets). All the rGO sheets, especially the N2H4–rGO one, showed significant change in the ATP level of the spermatozoa even at the lowest concentration tested (0.1 μg mL−1). In contrast, the GO sheets exhibited no significant ATP change at this concentration. At the highest concentration tested (400 μg mL−1), nearly all the graphene samples (especially N2H4–rGO, which induced the highest reduction of the ATP level) showed strong effects on the ATP level of the spermatozoa. In fact, the GO, HT-rGO, and GTP–rGO sheets with a concentration of 400 μg mL−1 resulted in the reduction of the ATP level by a factor of ∼0.3, while using the N2H4–rGO sheets, it decreased more strongly by a factor of ∼0.18. Since mitochondria are responsible for producing ATP in cells, any changes in ATP levels can be assigned to mitochondria dysfunction induced by graphene nanomaterials. The dysfunctionality of mitochondria can be assigned to ROS generated by the graphene nanomaterials (as discussed in the following) and/or direct physical contact interaction of the extremely sharp graphene sheets with spermatozoa having long flagellum (∼50 μm). In fact, the physical interaction can disrupt the mid parts of the spermatozoa where mitochondria produce ATP of the spermatozoa. Since the ATP level of the spermatozoa incubated with the N2H4–rGO exhibited a saturated (a concentration-independent) behavior at concentrations ≥100 μg mL−1, the prevention of ATP production by the graphene nanomaterials should not be considered as the only factor describing their cytotoxicity.
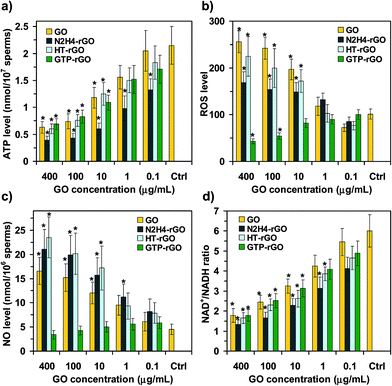 |
| Fig. 4 (a) ATP level, (b) ROS level, (c) NO concentration, and (d) NAD+/NADH ratio of spermatozoa after 2 h incubation with GO, N2H4–rGO, HT-rGO, and GTP–rGO with various concentrations as compared to the control sample. Significant results are marked by asterisks (*) for P-values < 0.05 (n = 3). | |
The viability and motility of spermatozoa can be significantly affected by ROS generation. It is one of the main agents of male infertility through inducing damages to DNA and/or membrane of spermatozoa. Fig. 4b shows ROS levels generated by the GO, N2H4–rGO, HT-rGO, and GTP–rGO sheets at various concentrations. No significant ROS generation occurred at the lowest concentration tested (0.1 μg mL−1). By increasing the concentration to 400 μg mL−1, the ROS level of the GO, HT-rGO and N2H4–rGO sheets increased by factors of ∼2.5, 2.2 and 1.7, respectively. It was found that for the unfiltered HT-rGO suspension, the ROS level sharply increased by a factor of ∼6.1 at the concentrations of 100 and 400 μg mL−1. Such high ROS levels were assigned to the oxygen dissolved in the aqueous solution, as one of the results of hydrothermal reaction. On the other hand, increasing the concentration of the GTP–rGO sheets resulted in a slight decrease of the ROS level. This reduction can be assigned to the presence of GTPs (as radical scavengers) on the reduced sheets. In fact, π–π attachment of the GTP molecules having an aromatic structure on the surface of the rGO sheets was previously demonstrated by using AFM analysis.49 These results (especially the higher ROS level of the GO and HT-rGO than that of the N2H4–rGO and the slight decrease of ROS level by increasing the GTP–rGO concentration) show that there are other factors (such as physical damaging and trapping) in addition to the ROS generation to completely describe the cytotoxic effects of the graphene nanomaterials on spermatozoa, as also studied further in the following.
A number of studies demonstrated that although low concentrations of NO (caused by e.g., ≤100 nM sodium nitroprusside) increase sperm capacitation, high concentrations of NO (caused by e.g., ≥200 nM sodium nitroprusside) induce cytotoxic effects resulting in the reduction of sperm motility and fertility.64 In fact, in recent years, NO has established itself as a key parameter, which plays a decisive role in regulating multiple functions within the reproductive agents such as spermatozoa.65 Fig. 4c presents sperm NO production in the presence of the GO, N2H4–rGO, HT-rGO, and GTP–rGO sheets with various concentrations. The GO sheets showed no significant NO production at 0.1 μg mL−1, while the N2H4–rGO and HT-rGO sheets resulted in slight increases of the NO production as compared to the control sample. By increasing the concentration to 400 μg mL−1, the sperm NO production level significantly increased in the presence of the GO, N2H4–rGO, and especially HT-rGO sheets. Although the ROS generation is one of the important agents in NO production, the higher NO production in the presence of the N2H4–rGO and HT-rGO sheets can be assigned to the nitrogen and oxygen contents of the aqueous solution obtained from the hydrazine reduction and hydrothermal reaction, respectively. The slight reduction of the NO level by increasing the GTP–rGO concentration confirmed the π–π attachment of the antioxidant GTP molecules on the reduced sheets.
NAD+/NADH ratio is an indicator that reflects metabolic and redox state of cells. Changes in NAD+ and NADH levels occur in response to different environmental stimuli. For example, cells increase their intracellular NAD+/NADH ratio under oxidative stress conditions in order to resist possible oxidative damages. Hence, in this study, the NAD+/NADH ratio was measured in order to better understand the toxic effects of the graphene nanomaterials on spermatozoa. Fig. 4d shows that the NAD+/NADH ratio decreased by increasing the concentration of graphene nanomaterials (independent from the chemical states of GO and rGO sheets). For all concentrations studied in this study, the N2H4–rGO sheets exhibited the highest changes in the NAD+/NADH ratio, while the GO sheets showed the lowest (but still significant) changes. At the highest concentration tested (400 μg mL−1), the NAD+/NADH ratio decreased >70% relative to the control sample condition. This means that the metabolic and redox state of spermatozoa were seriously disordered by the graphene nanomaterials. Although reduction in the metabolic activity of the spermatozoa by increasing the concentrations of the GO, N2H4–rGO, and HT-rGO sheets can be assigned to the increase of the ROS and NO production levels, it is not valid for the GTP–rGO sheets, which exhibited the decrease of both ROS and NO levels by increasing the concentration. Therefore, the ability of these functionalized reduced sheets in the physical trapping of the spermatozoa (as also previously reported for inactivation of bacteria19) can be considered as one of the mechanisms describing the cytotoxicity of the rGO sheets (see the movies of the spermatozoa trapped by the stacked rGO sheets in ESI†). Furthermore, physical damage of the cells by the extremely sharp edges of the graphene sheets (especially the unaccumulated rGO sheets having thinner thicknesses than GO) can be considered as another important mechanism describing the cytotoxicity.16–18 Here, the physical damage of the membrane of spermatozoa by the graphene sheets was investigated by monitoring the DNA fragmentation of the spermatozoa exposed to the graphene sheets (see the following).
We also checked the genotoxicity of the GO and rGO sheets by monitoring the DNA fragmentations of the spermatozoa. Fig. 5a and b show fluorescent images of spermatozoa in the absence of graphene (control group) and in the presence of GO sheets having the highest concentration tested (400 μg mL−1), after 2 h incubation. The DNA-fragmented spermatozoa with a long comet tail are observable in Fig. 5b. On the basis of the comet assay, Fig. 5c shows the DNA fragmentation of the spermatozoa (in terms of the percentage of DNA in the tail) exposed to the various concentrations of GO, N2H4–rGO, HT-rGO, and GTP–rGO sheets after 2 h incubation. Although the DNA fragmentation of spermatozoa increased by increasing the concentration of graphene nanomaterials (independent from the chemical states of GO and rGO sheets), the threshold concentration for observing the genotoxicity was found ∼1.0 μg mL−1 for the N2H4–rGO and HT-rGO sheets and ∼10 μg mL−1 for the GO and GTP–rGO sheets. In fact, among the graphene sheets investigated in this work, N2H4–rGO exhibited the highest genotoxicity, especially at concentrations ≥100 μg mL−1. This can be assigned to extremely sharp edges and/or high mobility of the single-layer N2H4–rGO sheets providing more effective interactions with membrane and the nucleus of the spermatozoa. On the other hand, the GTP–rGO sheets exhibited the lowest genotoxicity among all the graphene nanomaterials studied in this work. This can be attributed to the lower penetration of the GTP–rGO sheets into the spermatozoa due to the steric effect induced by the GTPs π–π attached on the surface of the reduced sheets. Therefore, GTPs not only could reduce GO using a green reduction method, but also yielded more biocompatible reduced sheets with lower genotoxic effects, as compared to the N2H4–rGO ones.
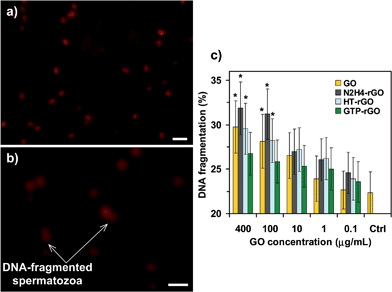 |
| Fig. 5 Comet assay of spermatozoa exposed to graphene sheets: fluorescent images of (a) spermatozoa in the absence of graphene (control group) and (b) the spermatozoa in the presence of GO sheets with concentration of 400 μg mL−1, after 2 h incubation. The DNA-fragmented spermatozoa present a long comet tail in (b). The scale bars show 50 μm. (c) DNA fragmentation of spermatozoa (obtained from the comet assay) after 2 h exposure to GO, N2H4–rGO, HT-rGO, and GTP–rGO with various concentrations as compared to the control sample. In each run, DNA fragmentation of ∼50 spermatozoa was checked. Significant results are marked by asterisks (*) for P-values < 0.05 (n = 3). | |
4. Conclusions
Concentration-dependent cyto and genotoxicities of GO, N2H4–rGO, HT-rGO, and GTP–rGO sheets on spermatozoa were investigated for the first time. All the graphene nanomaterials with concentrations ≥1.0 μg mL−1 exhibited cytotoxic effects on spermatozoa after 2 h incubation. In addition, all the GO and rGO sheets induced concentration-dependent reductions of ATP and NAD+/NADH produced by spermatozoa for motility and metabolic activity. While the GO, N2H4–rGO, and HT-rGO sheets increased the ROS and NO generations, the GTP–rGO decreased them (due to scavenging property of antioxidant GTP molecules attached on the reduced sheets). Thus, the physical trapping of spermatozoa by graphene sheets (especially the GTP–rGO one) was proposed as one of the effective mechanisms describing cytotoxicity, in addition to the reactions, resulting in the inactivation and/or death of spermatozoa. The genotoxic effects of the graphene nanomaterials was observed at the threshold concentrations of 1.0 μg mL−1 for the N2H4–rGO and HT-rGO, 10 μg mL−1 for the GO and GTP–rGO sheets. The high genotoxicity of the N2H4–rGO sheets was assigned to the ultra sharp edge and high mobility of the sheets with the capability of easy penetration into spermatozoa and interaction with the cell nuclei. On the other hand, the steric effect induced by the GTPs attached on the rGO caused a lower genotoxicity. In fact, GTPs not only can reduce GO using a green reaction, but also can yield more biocompatible rGO sheets with lower genotoxic effects, as compared to the N2H4–rGO and even GO ones, for numerous upcoming graphene-based bioapplications. Furthermore, the genotoxicity of GO and N2H4–rGO sheets released in the environment and/or body of living organisms can be significantly suppressed by natural antioxidants such as GTPs.
Acknowledgements
The authors would like to thank the Research Council of Sharif University of Technology for financial support of the work. Many thanks go to the National Institute of Genetic Engineering and Biotechnology for the facilitation of the process to conduct this study. Finally, the authors would like to express their appreciation to Dr Saman Hosseinkhani at Tarbiat Modares University, Dr Mojtaba Dashtizad and Dr Farid Heidari at National Institute of Genetic Engineering and Biotechnology and Miss Nayeralsadat Fatemi at Royan Institute for their technical supports.
References
- Y. B. Zhang, Y. Tan, H. L. Stormer and P. Kim, Experimental observation of quantum Hall effect and Berry's phase in graphene, Nature, 2005, 438, 201–204 CrossRef CAS PubMed.
- M. I. Katsnelson and K. S. Novoselov, Graphene: new bridge between condensed matter physics and quantum electrodynamics, Solid State Commun., 2007, 143, 3–13 CrossRef CAS PubMed.
- J. Bai, X. Zhong, S. Jiang, Y. Huang and X. Duan, Graphene nanomesh, Nat. Nanotechnol., 2010, 5, 190–194 CrossRef CAS PubMed.
- O. Akhavan, Graphene nanomesh by ZnO nanorod photocatalysts, ACS Nano, 2010, 4, 4174–4180 CrossRef CAS PubMed.
- F. Schedin, A. K. Geim, S. V. Morozov, E. W. Hill, P. Blake, M. I. Katsnelson and K. S. Novoselov, Detection of individual gas molecules adsorbed on graphene, Nat. Mater., 2007, 6, 652–655 CrossRef CAS PubMed.
- O. Akhavan, E. Ghaderi and R. Rahighi, Toward single-DNA electrochemical biosensing by graphene nanowalls, ACS Nano, 2012, 6, 2904–2916 CrossRef CAS PubMed.
- N. Mohanty and V. Berry, Graphene-based single-bacterium resolution biodevice and DNA transistor: interfacing graphene derivatives with nanoscale and microscale biocomponents, Nano Lett., 2008, 8, 4469–4476 CrossRef CAS PubMed.
- O. Akhavan and E. Ghaderi, Photocatalytic reduction of graphene oxide nanosheets on TiO2 thin film for photoinactivation of bacteria in solar light irradiation, J. Phys. Chem. C, 2009, 113, 20214–20220 CAS.
- O. Akhavan, E. Ghaderi and K. Rahimi, Adverse effects of graphene incorporated in TiO2 photocatalyst on minuscule animals under solar light irradiation, J. Mater. Chem., 2012, 22, 23260–23266 RSC.
- O. Akhavan, M. Choobtashani and E. Ghaderi, Protein degradation and RNA efflux of viruses photocatalyzed by graphene–tungsten oxide composite under visible light irradiation, J. Phys. Chem. C, 2012, 116, 9653–9659 CAS.
- H. Y. Mao, S. Laurent, W. Chen, O. Akhavan, M. Imani, A. A. Ashkarran and M. Mahmoudi, Graphene: promises, facts, opportunities, and challenges in nanomedicine, Chem. Rev., 2013, 113, 3407–3424 CrossRef CAS PubMed.
- K. H. Liao, Y. S. Lin, C. W. Mac Osko and C. L. Haynes, Cytotoxicity of graphene oxide and graphene in human erythrocytes and skin fibroblasts, ACS Appl. Mater. Interfaces, 2011, 3, 2607–2615 CAS.
- Y. Zhang, S. F. Ali, E. Dervishi, Y. Xu, Z. Li, D. Casciano and A. S. Biris, Cytotoxicity effects of graphene and single-wall carbon nanotubes in neural phaeochromocytoma-derived PC12 cells, ACS Nano, 2010, 4, 3181–3186 CrossRef CAS PubMed.
- Y. Chang, S. T. Yang, J. H. Liu, E. Dong, Y. Wang, A. Cao, Y. Liu and H. Wang, In vitro toxicity evaluation of graphene oxide on A549 cells, Toxicol. Lett., 2011, 200, 201–210 CrossRef CAS PubMed.
- O. Akhavan, E. Ghaderi and A. Akhavan, Size-dependent genotoxicity of graphene nanoplatelets in human stem cells, Biomaterials, 2012, 33, 8017–8025 CrossRef CAS PubMed.
- O. Akhavan and E. Ghaderi, Toxicity of graphene and graphene oxide nanowalls against bacteria, ACS Nano, 2010, 4, 5731–5736 CrossRef CAS PubMed.
- W. Hu, C. Peng, M. Lv, X. Li, Y. Zhang, N. Chen, C. Fan and Q. Huang, Protein corona-mediated mitigation of cytotoxicity of graphene oxide, ACS Nano, 2011, 5, 3693–3700 CrossRef CAS PubMed.
- O. Akhavan, E. Ghaderi and A. Esfandiar, Wrapping bacteria by graphene nanosheets for isolation from environment, reactivation by sonication and inactivation by near-infrared irradiation, J. Phys. Chem. B, 2011, 115, 6279–6288 CrossRef CAS PubMed.
- O. Akhavan, E. Ghaderi, H. Emamy and F. Akhavan, Genotoxicity of graphene nanoribbons in human mesenchymal stem cells, Carbon, 2013, 54, 419–431 CrossRef CAS PubMed.
- L. Q. Chen, P. P. Hu, L. Zhang, S. Z. Huang, L. F. Luo and C. Z. Huang, Toxicity of graphene oxide and multi-walled carbon nanotubes against human cells and zebrafish, Sci. China: Chem., 2012, 55, 2209–2216 CrossRef CAS.
- K. Yang, J. Wan, S. Zhang, B. Tian, Y. Zhang and Z. Liu, The influence of surface chemistry and size of nanoscale graphene oxide on photothermal therapy of cancer using ultra-low laser power, Biomaterials, 2011, 33, 2206–2214 CrossRef PubMed.
- O. Akhavan and E. Ghaderi, Graphene nanomesh promises extremely efficient in vivo photothermal therapy, Small, 2013, 9, 3593–3601 CrossRef CAS PubMed.
- J. T. Robinson, S. M. Tabakman, Y. Liang, H. Wang, H. Sanchez Casalongue, D. Vinh and H. Dai, Ultrasmall reduced graphene oxide with high near-infrared absorbance for photothermal therapy, J. Am. Chem. Soc., 2011, 133, 6825–6831 CrossRef CAS PubMed.
- O. Akhavan, E. Ghaderi and H. Emamy, Nontoxic concentrations of PEGylated graphene nanoribbons for selective cancer cell imaging and photothermal therapy, J. Mater. Chem., 2012, 22, 20626–20633 RSC.
- O. Akhavan, E. Ghaderi, S. Aghayee, Y. Fereydooni and A. Talebi, The use of a glucose-reduced graphene oxide suspension for photothermal cancer therapy, J. Mater. Chem., 2012, 22, 13773–13781 RSC.
- K. Yang, L. Hu, X. Ma, S. Ye, L. Cheng, X. Shi, C. Li, Y. Li and Z. Liu, Multimodal imaging guided photothermal therapy using functionalized graphene nanosheets anchored with magnetic nanoparticles, Adv. Mater., 2012, 24, 1868–1872 CrossRef CAS PubMed.
- M. Kalbacova, A. Broz, J. Kong and M. Kalbac, Graphene substrates promote adherence of human osteoblasts and mesenchymal stromal cells, Carbon, 2010, 48, 4323–4329 CrossRef CAS PubMed.
- T. R. Nayak, H. Andersen, V. S. Makam, C. Khaw, S. Bae, X. Xu, P. R. Ee, J. H. Ahn, B. H. Hong, G. Pastorin and B. Ozyilmaz, Graphene for controlled and accelerated osteogenic differentiation of human mesenchymal stem cells, ACS Nano, 2011, 5, 4670–4678 CrossRef CAS PubMed.
- W. C. Lee, C. H. Y. X. Lim, H. Shi, L. A. L. Tang, Y. Wang, C. T. Lim and K. P. Loh, Origin of enhanced stem cell growth and differentiation on graphene and graphene oxide, ACS Nano, 2011, 5, 7334–7341 CrossRef CAS PubMed.
- O. Akhavan, E. Ghaderi and M. Shahsavar, Graphene nanogrids for selective and fast osteogenic differentiation of human mesenchymal stem cells, Carbon, 2013, 59, 200–211 CrossRef CAS PubMed.
- S. Y. Park, J. Park, S. H. Sim, M. G. Sung, K. S. Kim, B. H. Hong and S. Hong, Enhanced differentiation of human neural stem cells into neurons on graphene, Adv. Mater., 2011, 23, H263–H267 CrossRef CAS PubMed.
- N. Li, X. Zhang, Q. Song, R. Su, Q. Zhang, T. Kong, L. Liu, G. Jin, M. Tang and G. Cheng, The promotion of neurite sprouting and outgrowth of mouse hippocampal cells in culture by graphene substrates, Biomaterials, 2011, 32, 9374–9382 CrossRef CAS PubMed.
- O. Akhavan and E. Ghader, Differentiation of human neural stem cells into neural networks on graphene nanogrids, J. Mater. Chem. B, 2013, 1, 6291–6301 RSC.
- X. Sun, Z. Liu, K. Welsher, J. T. Robinson, A. Goodwin, S. Zaric and H. Dai, Nano-graphene oxide for cellular imaging and drug delivery, Nano Res., 2008, 1, 203–212 CrossRef CAS PubMed.
- Z. Liu, J. T. Robinson, X. Sun and H. Dai, PEGylated nanographene oxide for delivery of water-insoluble cancer drugs, J. Am. Chem. Soc., 2008, 130, 10876–10877 CrossRef CAS PubMed.
- L. Zhang, J. Xia, Q. Zhao, L. Liu and Z. Zhang, Functional graphene oxide as a nanocarrier for controlled loading and targeted delivery of mixed anticancer drugs, Small, 2010, 6, 537–544 CrossRef CAS PubMed.
- P. R. Leroueil, S. Hong, A. Mecke, J. R. Baker Jr, B. G. Orr and M. M. Banaszak Holl, Nanoparticle interaction with biological membranes: Does nanotechnology present a janus face?, Acc. Chem. Res., 2007, 40, 335–342 CrossRef CAS PubMed.
- W. H. de Jong, W. I. Hagens, P. Krystek, M. C. Burger, A. J. A. M. Sips and R. E. Geertsma, Particle size-dependent distribution of gold nanoparticles after intravenous administration, Biomaterials, 2008, 29, 1912–1919 CrossRef CAS PubMed.
- I. D. Adler, Spermatogenesis and mutagenicity of environmental hazards: extrapolation of genetic risk from mouse to man, Andrologia, 2000, 32, 233–237 CrossRef CAS.
- L. Braydich-Stolle, S. Hussain, J. Schlager and M. C. Hofmann, In vitro cytotoxicity of nanoparticles in mammalian germ line stem cells, Toxicol. Sci., 2005, 88, 412–419 CrossRef CAS PubMed.
- K. Tremellen, Oxidative stress and male infertility – A clinical perspective, Hum. Reprod. Update, 2008, 14, 243–258 CrossRef CAS PubMed.
- E. de Lamirande, C. Tsai, A. Harakat and C. Gagnon, Involvement of reactive oxygen species in human sperm arcosome reaction induced by A23187, lysophosphatidylcholine, and biological fluid ultrafiltrates, J. Androl., 1998, 19, 585–594 CAS.
- W. S. Hummers and R. E. Offeman, Preparation of graphite oxide, J. Am. Chem. Soc., 1958, 80, 1339 CrossRef CAS.
- N. I. Kovtyukhova, P. J. Ollivier, B. R. Martin, T. E. Mallouk, S. A. Chizhik, E. V. Buzaneva and A. D. Gorchinskiy, Layer-by-layer assembly of ultrathin composite films from micron-sized graphite oxide sheets and polycations, Chem. Mater., 1999, 11, 771–778 CrossRef CAS.
- O. Akhavan, K. Bijanzad and A. Mirsepah, Synthesis of Graphene from Natural and Industrial Carbonaceous Wastes, RSC Adv., 2014, 4, 20441–20448 RSC.
- O. Akhavan, M. Kalaee, Z. S. Alavi, S. M. A. Ghiasi and A. Esfandiar, Increasing the antioxidant activity of green tea polyphenols in the presence of iron for the reduction of graphene oxide, Carbon, 2012, 50, 3015–3025 CrossRef CAS PubMed.
- W. C. L. Ford, Glycolysis and sperm motility: Does a spoonful of sugar help the flagellum go round?, Hum. Reprod. Update, 2006, 12, 269–274 CrossRef CAS PubMed.
- R. R. Tice, E. Agurell, D. Anderson, B. Burlinson, A. Hartmann, H. Kobayashi, Y. Miyamae, E. Rojas, J. C. Ryu and Y. F. Saski, Single cell gel/comet assay: guidelines for in vitro and in vivo genetic toxicology testing, Environ. Mol. Mutagen., 2000, 35, 206–221 CrossRef CAS.
- H. C. Schniepp, J. L. Li, M. J. McAllister, H. Sai, M. Herrera-Alonso, D. H. Adamson, R. K. Prud'homme, R. Car, D. A. Saville and I. A. Aksay, Functionalized single graphene sheets derived from splitting graphite oxide, J. Phys. Chem. B, 2006, 110, 8535–8539 CrossRef CAS PubMed.
- M. J. Mc Allister, J. L. Li, D. H. Adamson, H. C. Schniepp, A. A. Abdala, J. Liu, M. Herrera-Alonso, D. L. Milius, R. Car, R. K. Prud'homme and I. A. Aksay, Single sheet functionalized graphene by oxidation and thermal expansion of graphite, Chem. Mater., 2007, 19, 4396–4404 CrossRef CAS.
- O. Akhavan, The effect of heat treatment on formation of graphene thin films from graphene oxide nanosheets, Carbon, 2010, 48, 509–519 CrossRef CAS PubMed.
- Q. Su, S. Pang, V. Alijani, C. Li, X. Feng and K. Müllen, Composites of graphene with large aromatic molecules, Adv. Mater., 2009, 21, 3191–3195 CrossRef CAS.
- A. Esfandiar, O. Akhavan and A. Irajizad, Melatonin as a powerful bio-antioxidant for reduction of graphene oxide, J. Mater. Chem., 2011, 21, 10907–10914 RSC.
- J. Gao, F. Liu, Y. Liu, N. Ma, Z. Wang and X. Zhang, Environment-friendly method to produce graphene that employs vitamin C and amino acid, Chem. Mater., 2010, 22, 2213–2218 CrossRef CAS.
- O. Akhavan, E. Ghaderi, E. Abouei, S. Hatamie and E. Ghasemi, Accelerated differentiation of neural stem cells into neurons on ginseng-reduced graphene oxide sheets, Carbon, 2014, 66, 395–406 CrossRef CAS PubMed.
- F. Liu and T. S. Seo, A controllable self-assembly method for large-scale synthesis of graphene sponges and free-standing graphene films, Adv. Funct. Mater., 2010, 20, 1930–1936 CrossRef CAS.
- O. Akhavan and E. Ghaderi, Escherichia coli bacteria reduce graphene oxide to bactericidal graphene in a self-limiting manner, Carbon, 2012, 50, 1853–1860 CrossRef CAS PubMed.
- L. M. Malard, M. A. Pimenta, G. Dresselhaus and M. S. Dresselhaus, Raman spectroscopy in graphene, Phys. Rep., 2009, 473, 51–87 CrossRef CAS PubMed.
- D. Graf, F. Molitor, K. Ensslin, C. Stampfer, A. Jungen, C. Hierold and L. Wirtz, Spatially resolved Raman spectroscopy of single- and few-layer graphene, Nano Lett., 2007, 7, 238–242 CrossRef CAS PubMed.
- K. S. Kim, Y. Zhao, H. Jang, S. Y. Lee, J. M. Kim, K. S. Kim, J. H. Ahn, P. Kim, J. Y. Choi and B. H. Hong, Large-scale pattern growth of graphene films for stretchable transparent electrodes, Nature, 2009, 457, 706–710 CrossRef CAS PubMed.
- I. Calizo, A. A. Balandin, W. Bao, F. Miao and C. N. Lau, Temperature dependence of the Raman spectra of graphene and graphene multilayers, Nano Lett., 2007, 7, 2645–2649 CrossRef CAS PubMed.
- L. Liu, S. Ryu, M. R. Tomasik, E. Stolyarova, N. Jung, M. S. Hybertsen, M. L. Steigerwald, L. E. Brus and G. W. Flynn, Graphene oxidation: thickness-dependent etching and strong chemical doping, Nano Lett., 2008, 8, 1965–1970 CrossRef CAS PubMed.
- R. R. Arivizo, O. R. Miranda, M. A. Thompson, C. M. Pabelick, R. Bhattacharya, J. D. Robertson, V. M. Rotello, Y. S. Prakash and P. Mukherjee, Effect of nanoparticle surface charge at the plasma membrane and beyond, Nano Lett., 2010, 10, 2543–2548 CrossRef PubMed.
- H. Zhang and R. L. Zheng, Possible role of nitric oxide on fertile and asthenozoospermic infertile human sperm functions, Free Radical Res., 1996, 25, 347–354 CrossRef CAS.
- M. Rosselli, P. J. Keller and R. K. Dubey, Role of nitric oxide in the biology, physiology and pathophysiology of reproduction, Hum. Reprod. Update, 1998, 4, 3–24 CrossRef CAS PubMed.
Footnote |
† Electronic supplementary information (ESI) available. See DOI: 10.1039/c4ra01047g |
|
This journal is © The Royal Society of Chemistry 2014 |