DOI:
10.1039/C4RA00781F
(Review Article)
RSC Adv., 2014,
4, 14328-14334
Mesoporous silica nanoparticles: importance of surface modifications and its role in drug delivery
Received
27th January 2014
, Accepted 6th February 2014
First published on 6th February 2014
Abstract
Mesoporous nanoparticles are special examples of silica nanoparticles. They are well known for their variety of applications in various fields including biomedicine. This has been attributed to their size, tenability and easily modifiable capabilities because of the presence of functional groups, and their biocompatibility. In this review, we specifically focus on different modes of surface modifications using amino propyl and organic chains which facilitate maximum drug loading and sustained release. This review provides complete information about the importance of surface modifications and their biological consequences that will be helpful to understand the use of MCM-41 and SBA 15 as suitable drug delivery systems.
1 Introduction
Mesoporous silica nanoparticles (MSNs) have found several applications in the areas of drug delivery,1 drug targeting,2,3 gene transfection,4–7 tissue engineering7–9 and cell tracking.9–12 The reason for this wide range of applications is their unique structure. They have high internal volumes, large surface areas and narrow channels which allow the adsorption of drugs and proteins into their structure. The straight channels allow the diffusion of adsorbed drugs into the surrounding tissues over a period of time (controlled release). This release property depends on a number of characteristics like the nature of the drug, the release medium, pore size, surface functionalization and particle size and morphology. They also offer an advantage that the channels can be used as a reservoir system and its opening and closing can be controlled by different systems, for example polymers,13,14 nano-crystals15 or photoactive derivatives,16 and external triggers such as heat,17 pH,13 light,16 chemicals.15 Generally, the functionalization of mesoporous nanoparticles is done in order to enhance their physical properties to ensure better drug delivery, higher adsorption of the drug and also for sustained release of drugs in the target. The dosage and the delivery rate of a particular drug can be controlled with great efficiency by altering one or more of the following parameters: the structure and composition of the matrix,18,19 the pore size,20,21 pore entrance modification via photo-dimerization,16 and the chemical interaction with the host.22 There are numerous reports available in the literature to describe the drug delivery applications of mesoporous silica nanoparticles. Researchers have made continuous attempts to use this material for different types of pharmacologically important drugs to improve their efficiency and reduce cytotoxicity. In this context, ramipril is the drug used for treatment for myocardial infarction but its causes several side effects like reduce the glucose level, neutropenia, etc. Therefore scientists have demonstrated the sustained delivery of ramipril using mesoporous silica nanoparticles. The findings indicated that mesoporous silica nanoparticles show good response for the sustained release of the ramipril drug.23 In another report on silica nanorods for evaluating bactericidal activity by releasing nitric oxide, the findings indicated that particle size and shape greatly influence the release property of the drug, which also correlates with the bactericidal activity of mesoporous silica nanorods.24 In this review, we attempt to explain the importance of different surface modifications of the mesoporous materials with special emphasis on SBA 15 and MCM-41. We have also analysed the issue of biocompatibility and the possible cytotoxic effects arising due to several such modified silica particles. Fig. 1 depicts the morphology of SBA 15 and MCM 41.
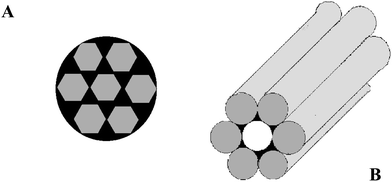 |
| Fig. 1 Schematic representation of MCM-41 (A) and SBA-15 (B). | |
2 Different types of chemical modification of the mesoporous nanoparticles
Mesoporous materials are well known for their ability to be chemically modified because of the presence of silanol groups. A scan of the literature reveals that numerous works have been carried out using modified mesoporous silica to improve their biomedical applications. Fig. 2 depicts the different possible surface modifications in the hydroxyl groups of mesoporous materials which includes thiol, organic chain, amine and hydroxyl modifications.
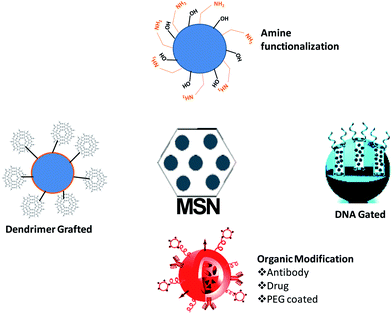 |
| Fig. 2 Different modes of surface modifications in SBA 15 and MCM 41 reported in the literature. | |
The modified surface of the mesoporous materials plays a vital role in the delivery of drugs. Table 1 summarizes important surface modifications and their role in drug delivery. The major function of different surface modifications is their use to control the release of the drug based on particular stimuli or physiological conditions. A scan of the literature clearly indicates that the different modes of surface modification of mesoporous silica nanoparticles definitely improve the drug loading specifically for DNA and siRNA and also play a role in the delivery of drugs in suitable stimulated conditions. This is favourable for the targeted delivery of drugs which improves the therapeutic efficiency of different cytotoxic drugs, as well as reducing their toxicity compared to the free drug.
Table 1 The essence of studied surface modifications and the results that were obtained
S. No |
Experiment performed |
Drug loaded |
Inference |
Reference |
1 |
MCM 41 modified with alumina silicate |
Amikacin |
Loading is mainly depends on pore volume and alumina content |
25 |
2 |
Amino-functionalized mesoporous silica nanoparticles |
pcDNA3.1(+)-PKB-HA |
Amine functionalization improves the loading of DNA into the mesoporous silica nanoparticles |
26 |
3 |
Organic functionalized mesoporous silica nanoparticles |
Molsidomine |
Mercaptopropyl-modified mesoporous silica nanoparticles are more favourable for drug loading compared to other modifications |
27 |
4 |
Ruthenium complexes-grafted chemo switchable mesoporous silica nanoparticles |
Cytotoxic drugs |
Ruthenium complex-grafted hybrid mesoporous silica nanoparticles favour the specific release of the cytotoxic drugs to breast cancer cells |
28 |
5 |
Poly (ε–caprolactone) functionalized mesoporous silica nanoparticles |
Ammonium glycyrrhizinate |
Modified mesoporous silica nanoparticles show better efficiency for loading and sustained release |
29 |
6 |
Organic modified SBA 15 |
Nicotinamide |
Sulphopropyl-modified SBA 15 exhibits superior drug loading efficiency and more sustained release kinetics compared to others |
30 |
7 |
Nucleic acid-capped mesoporous silica nanoparticles |
Camptothecin |
Chemo-switchable silica nanoparticles show better selective release of the drug compared to unmodified SBA 15 |
31 |
8 |
Antibody conjugated radio-labelled mesoporous silica nanoparticles |
Doxorubicin |
The in vivo experiments reveal that antibody coated mesoporous silica nanoparticles mainly accumulate in the tumour sites compared to non-tagged silica nanoparticles |
32 |
9 |
Polymer-coated mesoporous silica nanoparticles |
Doxorubicin |
Polymer-coated mesoporous silica nanoparticles show a selective release of drugs in response to redox activity |
33 |
10 |
Stimuli-responsive organic modified mesoporous silica nanoparticles |
Camptothecin and doxorubicin |
Modified silica nanoparticles release the drugs only in the acidic environment which mimics the cancer tissue |
34 |
11 |
Thiol-modified mesoporous silica nanoparticles |
Rhodomine B |
Modified silica nanoparticles release the drug in the presence of dithithreitol and did not induce a teratogenic effect in the Zebra fish |
35 |
12 |
PAMAM dendrimer-grafted silica nanoparticles |
Aluminum phthalocyanine tetrasulfonate |
They show better efficiency to release the drug in the target tissue compared to free drug against breast cancer cells |
36 |
13 |
MCM-41 modification with amino propyl groups |
Aspirin |
Release property depended on amount of amino functional groups |
37 |
14 |
Functionalization of MCM-41 and SBA-15 with 3 amino propyl and secondary amino groups |
Ibuprofen |
Higher adsorption capacity of the drug |
38 |
15 |
Amine functionalization of MCM-41 and SBA-15 |
Pt nano-catalysts |
Restoration of catalytic activity, increased stability and increased life time |
39 |
16 |
Amino group functionalization of spherical MSNs |
Ibuprofen |
Decreased pore size and volume and increased adsorption of the drug |
38 |
17 |
Organic chain modification of MSNs |
Dexmedetomidine |
Release property was governed by the degradation rate of the MSNs |
40 |
18 |
Functionalization with long alkyl chains |
— |
Increased the hydrophobicity and decreased the degradation rate |
41 |
19 |
PEGylation of MSNs |
Doxorubicin |
Enhanced targeted delivery, increased circulation time, slower degradation rate |
42 |
20 |
Functionalization using PEI |
P-glycoprotein si-RNA |
Targeted delivery to MDR cancer cells and higher stability |
42 |
21 |
Coupling MSNs with DNA switches |
Ru(bipy)32+ |
Could be used for intracellular drug delivery owing to increased permeability and biocompatibility |
43–45 |
22 |
MCM 41 thiol-modified |
5-Fluorouracil |
GSH induced drug release for breast cancer treatment |
1 |
2.1 Influence of amino-propyl groups
Modified MCM-41 was prepared by Zeng et al.46 and the model drug was loaded by mixing equal concentrations of aspirin and MCM-41. On performing the release experiment in a medium mimicking the body fluid it was found that the release properties were found to directly depend on the amount of amino-propyl groups present on the surface of the mesoporous silica particles. One of the perks of such a modification was that the ordered structure of the mesoporous silica particle remained unaltered. More importantly the channels present in MCM-41 were also found to be intact. It was also proved that surface functionalization had a key role in other important parameters like adsorption capacity of the drug as shown by Wang et al.47 The study indicated that adsorption capacity of a drug depended on both its own properties and also the properties of the functional groups used for modification of the surface. Wang et al. showed that the drug ibuprofen had a higher adsorption capacity when it was adsorbed on to MCM-41 and SBA-15 mesoporous particles that were surface functionalized with 3-amino propyl groups and secondary amine groups. Elsewhere the nanoparticles which were surface functionalized using organic groups showed a higher adsorption capacity for the model drug, rhodamine. A plausible reason for this result is that ibuprofen contains a carboxylic group (–COOH) which can interact with the amino groups (–NH2 and –NH) present in the amine functionalized mesoporous particles through strong hydrogen bonding. The release properties as against the adsorption properties were found to be influenced by the type of interaction between the drug molecule and the surface groups. The release of ibuprofen was influenced by the hydrogen bonding. Vallet-Regí et al.48 proved that apart from the mentioned parameters, the loading of the drug or the drug
:
host ratio also plays a key role in the release kinetics. Interestingly the samples of Wang et al. showed a slower drug release profile initially and then later showed a fast release profile (Fig. 3).
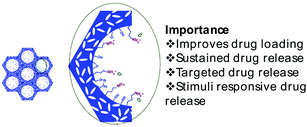 |
| Fig. 3 Representation of amine-functionalized mesoporous silica nanoparticles and their role in drug delivery. | |
When spherical MCM-41 and SBA-15 particles were amino-functionalized it was reported that there was a significant decrease in the pore size and volume.38 Modification of the MCM-41 and SBA-15 surfaces with amino groups increased the adsorptive capacity for the model drug ibuprofen.38 Recently, scientists demonstrated the efficacy of amine-functionalized mesoporous silica nanoparticles for gene delivery applications. The results indicated that amino group-modified mesoporous silica nanoparticles potentially improve the loading of pcDNA3.1(+)-PKB-HA DNA and also increase the transfection of DNA for gene delivery applications.26
2.2 Functionalization using organic chains
Mesoporous silica nanoparticles have a large pore size and a high pore volume properties which are required for us to effectively utilize these silica particles for drug delivery purposes. It was found that SBA-15, when calcined, acquired the ability to absorb large amounts of the drug inside the porous network and the release properties were also quantitative under in vitro conditions.18,48 However to control the drug release the chemical nature of the pore must be modified in a manner similar to that of MCM-41.22,49 Experiments on the drug release properties of these surface-functionalized silica particles revealed that the release of the drug, for example dexmedetomidine, was controlled by the degradation of the silica.40 Another observation was that modification of these silica nanoparticles by alkyl chains increased the hydrophobicity of the resultant material and decreased its degradation rate, thus making it simple for us to control the release properties.50 Functionalization with long chain organic compounds like C8 and C18 is bound to have certain effects on the silica nanoparticles. Elaborating upon that we find that such modifications will: first, decrease the pore size; second, modify the chemical interaction between the adsorbed drug molecules and pore surface; and third, decrease the wettability of the pore surface by aqueous solutions.
The functionalization procedures using C8 and C18 chains did not affect the structural order of the mesoporous particles.41 An effective method to control the erythromycin release rate was achieved by functionalization of SBA-15 with long chain alkyl groups.41 Another reason for functionalizing the MSNs with organic chains is to improve their biofunctionality. This has lead to the development of hybrid MSNs. These particles incorporate organic polymers like polyethylene glycol (PEG) or polyethyleneimine (PEI) onto their inner or outer surfaces. These organic modifications have several applications, like increasing stability of drugs and enhancing their targeted delivery. They also enable the MSNs to react to the environment e.g. smart nanoparticles.
PEGylation of nanoparticles increases their time of circulation, increases their resistance to proteolysis and decreases their susceptibility to immune reaction.51,52 Ho et al. coated PEGylated phospholipids onto hydrophobic polymers.52 This increases their suspension stability in water and also decreases non-specific protein binding ability.53 It was shown that MSNs which were PEGylated had a greater suspension in PBS and lower non specific binding when compared to normal MSNs. On the other hand when MSNs were modified using PEI, they showed an enhanced drug delivery primarily because PEI promotes the cytoplasmic release of the drug by destabilizing the lysosomal membranes.54,55 However one of the principle advantages of organic modifications is that the MSNs can be used to deliver more than one commodity into cells. Meng et al. proposed a dual drug delivery system using this principle. It was used to deliver doxorubicin and P-glycoprotein si-RNA to multi drug resistant (MDR) cancer cells. This dual delivery system had greater cytotoxic effects because of their synergism.42 There are other reports on different organic modifications of mesoporous silica nanoparticles for drug delivery applications available in the literature. A recent report on the organic modification of mesoporous nanoparticles indicated that mercaptopropyl-modified silica nanoparticles are more favourable for molsidomine drug loading and also shows better sustained release properties compared to other organic modifications of the materials.27 Other reports in the literature demonstrated that polycaprolactone-coated mesoporous silica nanoparticles exhibit better efficiency for loading ammonium glycyrrhizinate and show more sustained release properties compared to unmodified mesoporous materials. Another report on polymer-coated mesoporous silica nanoparticles also exhibited better efficiency for loading doxorubicin as well as exhibiting a sustained release pattern for the release of drugs from the matrix compared to unmodified mesoporous silica nanoparticles based on the reduction and oxidation behaviour of polymer.33 This may be used as a suitable drug delivery carrier for therapeutic applications. Likewise, different propyl group-tagged mesoporous nanoparticles have also been demonstrated as useful for drug delivery applications. The findings indicated that sulphopropyl-modified silica nanoparticles exhibit the maximum loading efficiency and also have better sustained release properties compared to other modifications.30 On the other side, mesoporous silica nanoparticles coated with the PAMAM dendrimer show better stability and release the photodynamic agent aluminum phthalocyanine tetrasulfonate to the target tissue specifically in the breast cancer, and also show superior cytotoxicity when a light source is applied, compared to free drug36 (Fig. 4).
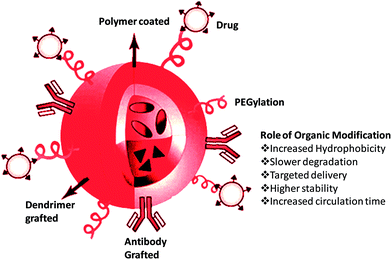 |
| Fig. 4 Influence of different organic chain modifications on the mesoporous materials and their role in drug delivery as reported in the literature. | |
2.3 Functionalization with DNA molecule gated switches
It is possible that mesoporous silica nanoparticles (MSN) could be used for making a stimuli-responsive controlled release system which is functionalized with DNA molecule gated switches.56 The MSNs were fabricated with cytosine rich DNA that was employed as the smart molecule gated switch. In the presence of Ag+ ions the closer C rich DNA hybridises with each other forming a C–Ag+–C structure.56 This depends on the metal dependant pairs of two nucleobases and this is responsible for the blocking of pores and packing of molecules. The controlled release of the particles is because of a competitive displacement reaction in which the DNA containing the C–Ag+–C complex deforms into single stranded DNA in the presence of thiol-containing molecules like dithriothretol (DTT). This leads to uncapping and the subsequent release of the entrapped guest particles. This reversible open and closed configuration can be achieved by altering the concentrations of Ag+ linkers and thiol molecules.56 More importantly this controlled release system showed significantly less cytotoxicity even at high concentrations (200 μg mL−1). Secondly, since this system can easily enter the cells through endocytosis it can be effectively used for the controlled release of nanodevices. There are several stimuli which can trigger this controlled release, including temperature,57,58 redox activation,59 photo-irradiation,60,61 enzymes62,63 and pH.57,61 However the major limitation of the existing capping systems is that they are poorly soluble in water and are toxic. Hence biomolecules like proteins,59 carbohydrates,60,61 nucleotides62,63 could be used as molecular gates. The use of DNA as a molecular gate in stimuli-responsive delivery systems is effective because of its programmable sequence recognition and conformational polymorphism.
These types of carrier systems can be developed by combining these nucleotides with a MSN container. A DNA controlled molecular valve was designed by attaching biotinylated DNA to the pore outlet of MSNs and they were operated by DNA strand melting at the particular melting temperature of the oligonucleotide.64 DNA caps which could undergo conformational changes were reported as gatekeepers for MCM-41 particles.65 Later a DNA nanogate which can be controlled by DNAse I was developed.43 Similarly a DNA controlled release system was developed using ATP binding aptamers which were attached to mesoporous silica particles. This was used for the ATP responsive release of the guest particles. These designs are based on the hydrogen bonding between the nucleotide bases and ensure efficient drug loading and release. However this design is limited by its instability in complex environments and the irreversibility of the closed–open gate system. Thus as an alternative Ren et al. designed a release system based on an i-motif on the DNA which was shown to undergo conformational changes on addition of NaOH or HCl.44,45 One limitation of this design was that it was susceptible to base driven release rendering it unsuitable for biological applications. It is known that metal ions like mercury and silver can form stable complexes with the cytosine or thymine residues of DNA.44,45,66,67 This principle is used to make systems which perform controlled release. DNA rich in cytosine is grafted into the pore outlets of MSNs and the binding with Ag+ ions result in closing of the outlet because of formation of this complex. The opening of the pore is controlled by the change in the conformation of the C–Ag+–C complex. This change again was shown to be mediated by the presence of thiol groups which had a higher affinity for the silver ions than the cytosine residues. It was reported that the amount of release was dependant on the concentration of the thiol groups. When the cellular uptake and cytotoxicity was tested it was shown that uptake was due to endocytosis and there was minimal toxicity. Hence these type of mesoporous silica nanoparticle-based delivery systems could be used for intracellular drug delivery because of their high cellular permeability and biocompatibility45 (Fig. 5). Recently, researchers extended the chemo-switchable drug delivery properties of mesoporous silica nanoparticles through different experimental and modification methodologies to improve the drug delivery properties of silica nanoparticles. In this context, ruthineum complexed DNA gated chemo-switchable mesoporous silica nanoparticles act as better gated drug delivery systems for different cytotoxic drugs including paclitaxel and docetaxel. The results indicated that surface modified mesoporous silica nanoparticles selectively release the ruthenium with respect to photo-induction to the breast cancer cells that may lead to these modified materials being used as a suitable candidate for drug delivery.28 Likewise, another recent report indicated that nucleic acid-capped mesoporous silica nanoparticles show superior characteristics for the delivery one of anticancer drug, camptothecin, to reduce toxicity as well as sustaining in the target location.31
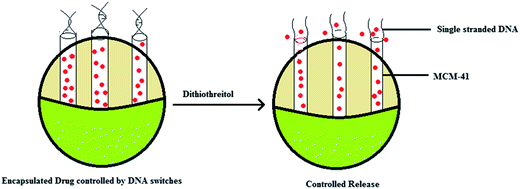 |
| Fig. 5 DNA gated drug release from mesoporous materials such as MCM 41 and SBA 15. | |
2.4 Biocompatibility and cytotoxicity
Although there is evidence that silica nanoparticles are biocompatible and degrade over a period of time in our body,68–71 less is known about the inherent biocompatibility and biodegradability of these MSN's especially after surface modifications. In vitro toxicity studies show that at low concentrations these exhibit low toxicity,15,72,73 however as the concentration of the MSNs increase their toxicity also was found to increase. In vivo studies have shown that the toxicity depends on the size of the particles.74 It was observed that the tissue toxicity of MSNs was comparable to the other drug carrier particles even when given in larger concentrations,75 whereas the systemic toxicity was much higher when compared to conventional polymeric drug carriers. Hudson et al. reported that when 30 mg of the nanoparticles were injected into an animal, it caused either death or extreme distress which necessitated euthanasia whereas even when 100 mg of poly(lactic-co-glycolic acid) polymer was injected it did not elicit any substantial lethal effects.76 Hence, choosing an appropriate system for the delivery of the nanoparticles is essential especially if a large quantity of particles must be delivered. However it should be noted that these toxicity effects can be mitigated by using strategies like the surface adsorption of proteins, functional groups of polymers etc. Blumen et al. showed that PEGylation reduced the toxicity of these MSNs in the lung tissue.56 Such strategies could be used to reduce the toxicity of the nanoparticles. The toxicity of the mesoporous nanoparticles is not due to the degradation of the particles but due to interaction with the particles themselves as revealed by the 100 percent cell viability in particle-free suspensions of SBA-15. The cell death was found to increase with increases in the time of exposure. In the cytotoxicity assays done by Hudson et al. it was found that MCM-41 was more toxic to mesothelial cells but slightly more toxic to macrophages when compared to other particles. This toxicity was dose and time dependant. The particle size was found to play no significant role in cellular cytotoxicity. It was reported that mesoporous silica particles accumulated in the sciatic nerve region and in the subcutaneous layer up to 3 months. When doses of 30 mg were administered the mice died within 24 hours or had to be euthanized. The non fatal dosage was estimated to be below 5 mg. Unfunctionalised nanoparticles showed local biocompatibility but also systemic toxicity. Since these particles are not soluble in solution their toxicity is measured by their Q50 value. On the contrary, some of the reports indicated that the toxicity of mesoporous silica nanoparticles mainly depends on the mass of the materials which is their M50 value.77
3 Conclusion
This review highlights the effects of functionalization of mesoporous nanoparticles on drug adsorption and its controlled delivery. However, we face a lot of challenges in making this system advanced and available for clinical purposes. We have to address biocompatibility issues and also it is critical that these MSNs are engineered for controlled release of multiple drugs. Similarly achieving a high drug loading capacity and large scale synthesis is also important. While drug delivery is important it is equally essential to ensure that there is an even distribution of the drug inside our body. Further a detailed study of the acute and chronic toxicities elicited by these MSNs must be studied under in vivo conditions.
Acknowledgements
The authors wish to acknowledge SASTRA University for financial and infrastructural support.
References
- B. Murugan, L. Narashimhan Ramana, S. Gandhi, S. Sethuraman and U. M. Krishnan, J. Mater. Chem. B, 2013, 1, 3494–3505 RSC
. - L. Pasqua, F. Testa, R. Aiello, S. Cundari and J. B. Nagy, Microporous Mesoporous Mater., 2007, 103, 166–173 CrossRef CAS PubMed
. - J. Gu, W. Fan, A. Shimojima and T. Okubo, Small, 2007, 3, 1740–1744 CrossRef CAS PubMed
. - D. R. Radu, C.-Y. Lai, K. Jeftinija, E. W. Rowe, S. Jeftinija and V. S. Y. Lin, J. Am. Chem. Soc., 2004, 126, 13216–13217 CrossRef CAS PubMed
. - S. R. Blumen, K. Cheng, M. E. Ramos-Nino, D. J. Taatjes, D. J. Weiss, C. C. Landry and B. T. Mossman, Am. J. Respir. Cell Mol. Biol., 2007, 36, 333–342 CrossRef CAS PubMed
. - B. G. Trewyn, J. A. Nieweg, Y. Zhao and V. S. Y. Lin, Chem. Eng. J., 2008, 137, 23–29 CrossRef CAS PubMed
. - I. Izquierdo-Barba, L. Ruiz-González, J. C. Doadrio, J. M. González-Calbet and M. Vallet-Regí, Solid State Sci., 2005, 7, 983–989 CrossRef CAS PubMed
. - I. Izquierdo-Barba, Á. Martinez, A. L. Doadrio, J. Pérez-Pariente and M. Vallet-Regí, Eur. J. Pharm. Sci., 2005, 26, 365–373 CrossRef CAS PubMed
. - X. Li, J. Shi, Y. Zhu, W. Shen, H. Li, J. Liang and J. Gao, J. Biomed. Mater. Res., Part B, 2007, 83B, 431–439 CrossRef CAS PubMed
. - Y.-S. Lin, C.-P. Tsai, H.-Y. Huang, C.-T. Kuo, Y. Hung, D.-M. Huang, Y.-C. Chen and C.-Y. Mou, Chem. Mater., 2005, 17, 4570–4573 CrossRef CAS
. - S.-H. Wu, Y.-S. Lin, Y. Hung, Y.-H. Chou, Y.-H. Hsu, C. Chang and C.-Y. Mou, ChemBioChem, 2008, 9, 53–57 CrossRef CAS PubMed
. - C.-P. Tsai, Y. Hung, Y.-H. Chou, D.-M. Huang, J.-K. Hsiao, C. Chang, Y.-C. Chen and C.-Y. Mou, Small, 2008, 4, 186–191 CrossRef CAS PubMed
. - Q. Yang, S. Wang, P. Fan, L. Wang, Y. Di, K. Lin and F.-S. Xiao, Chem. Mater., 2005, 17, 5999–6003 CrossRef CAS
. - H. J. Kim, H. Matsuda, H. Zhou and I. Honma, Adv. Mater., 2006, 18, 3083–3088 CrossRef CAS
. - C.-Y. Lai, B. G. Trewyn, D. M. Jeftinija, K. Jeftinija, S. Xu, S. Jeftinija and V. S. Y. Lin, J. Am. Chem. Soc., 2003, 125, 4451–4459 CrossRef CAS PubMed
. - N. K. Mal, M. Fujiwara and Y. Tanaka, Nature, 2003, 421, 350–353 CrossRef CAS PubMed
. - Z. Zhou, S. Zhu and D. Zhang, J. Mater. Chem., 2007, 17, 2428–2433 RSC
. - A. L. Doadrio, E. M. B. Sousa, J. C. Doadrio, J. Pérez Pariente, I. Izquierdo-Barba and M. Vallet-Regí, J. Controlled Release, 2004, 97, 125–132 CrossRef CAS PubMed
. - Q. He and J. Shi, J. Mater. Chem., 2011, 21, 5845–5855 RSC
. - P. Horcajada, A. Rámila, J. Pérez-Pariente and R. Vallet-Regí, Microporous Mesoporous Mater., 2004, 68, 105–109 CrossRef CAS PubMed
. - H. Hata, S. Saeki, T. Kimura, Y. Sugahara and K. Kuroda, Chem. Mater., 1999, 11, 1110–1119 CrossRef CAS
. - B. Muñoz, A. Rámila, J. Pérez-Pariente, I. Díaz and M. Vallet-Regí, Chem. Mater., 2002, 15, 500–503 CrossRef
. - Q.-Z. Zhai, Y.-Y. Wu and X.-H. Wang, J. Inclusion Phenom. Macrocyclic Chem., 2013, 77, 113–120 CrossRef CAS
. - Y. Lu, D. L. Slomberg, B. Sun and M. H. Schoenfisch, Small, 2013, 9, 2189–2198 CrossRef CAS PubMed
. - S. Nastase, L. Bajenaru, C. Matei, R. A. Mitran and D. Berger, Microporous Mesoporous Mater., 2013, 182, 32–39 CrossRef CAS PubMed
. - K. Zheng, H. Yang, L. Wang, S. Jing, H. Huang, J. Xu and P. Zhang, J. Porous Mater., 2013, 1–6 CrossRef CAS PubMed
. - N. A. Alyoshina and E. V. Parfenyuk, J. Solid State Chem., 2013, 205, 211–216 CrossRef CAS PubMed
. - M. Frasconi, Z. Liu, J. Lei, Y. Wu, E. Strekalova, D. Malin, M. W. Ambrogio, X. Chen, Y. Y. Botros, V. L. Cryns, J.-P. Sauvage and J. F. Stoddart, J. Am. Chem. Soc., 2013, 135, 11603–11613 CrossRef CAS PubMed
. - Y. Zhang, Z. Wang, W. Zhou, G. Min and M. Lang, Appl. Surf. Sci., 2013, 276, 769–775 CrossRef CAS PubMed
. - M. Moritz, Appl. Surf. Sci., 2013, 283, 537–545 CrossRef CAS PubMed
. - Z. Zhang, D. Balogh, F. Wang, S. Y. Sung, R. Nechushtai and I. Willner, ACS Nano, 2013, 7, 8455–8468 CrossRef CAS PubMed
. - F. Chen, H. Hong, Y. Zhang, H. F. Valdovinos, S. Shi, G. S. Kwon, C. P. Theuer, T. E. Barnhart and W. Cai, ACS Nano, 2013, 7, 9027–9039 CrossRef CAS PubMed
. - J. T. Sun, J. G. Piao, L. H. Wang, M. Javed, C. Y. Hong and C. Y. Pan, Macromol. Rapid Commun., 2013, 34, 1387–1394 CrossRef CAS PubMed
. - Z.-Y. Li, Y. Liu, X.-Q. Wang, L.-H. Liu, J.-J. Hu, G.-F. Luo, W.-H. Chen, L. Rong and X.-Z. Zhang, ACS Appl. Mater. Interfaces, 2013, 5, 7995–8001 CAS
. - P. Nadrah, U. Maver, A. Jemec, T. Tišler, M. Bele, G. Dražić, M. Benčina, A. Pintar, O. Planinšek and M. Gaberšček, ACS Appl. Mater. Interfaces, 2013, 5, 3908–3915 CAS
. - X. Tao, Y.-J. Yang, S. Liu, Y.-Z. Zheng, J. Fu and J.-F. Chen, Acta Biomater., 2013, 9, 6431–6438 CrossRef CAS PubMed
. - A. Datt, I. El-Maazawi and S. C. Larsen, J. Phys. Chem. C, 2012, 116, 18358–18366 CAS
. - A. Szegedi, M. Popova, I. Goshev and J. Mihály, J. Solid State Chem., 2011, 184, 1201–1207 CrossRef CAS PubMed
. - T. Huang and W. Tu, Appl. Surf. Sci., 2009, 255, 7672–7678 CrossRef CAS PubMed
. - P. Kortesuo, M. Ahola, M. Kangas, T. Leino, S. Laakso, L. Vuorilehto, A. Yli-Urpo, J. Kiesvaara and M. Marvola, J. Controlled Release, 2001, 76, 227–238 CrossRef CAS
. - J. C. Doadrio, E. M. B. Sousa, I. Izquierdo-Barba, A. L. Doadrio, J. Perez-Pariente and M. Vallet-Regí, J. Mater. Chem., 2006, 16, 462–466 RSC
. - H. Meng, M. Liong, T. Xia, Z. Li, Z. Ji, J. I. Zink and A. E. Nel, ACS Nano, 2010, 4, 4539–4550 CrossRef CAS PubMed
. - A. Schlossbauer, S. Warncke, P. M. E. Gramlich, J. Kecht, A. Manetto, T. Carell and T. Bein, Angew. Chem., Int. Ed., 2010, 49, 4734–4737 CrossRef CAS PubMed
. - C. Chen, J. Geng, F. Pu, X. Yang, J. Ren and X. Qu, Angew. Chem., Int. Ed., 2011, 50, 882–886 CrossRef CAS PubMed
. - C. Chen, F. Pu, Z. Huang, Z. Liu, J. Ren and X. Qu, Nucleic Acids Res., 2011, 39, 1638–1644 CrossRef CAS PubMed
. - W. Zeng, X.-F. Qian, Y.-B. Zhang, J. Yin and Z.-K. Zhu, Mater. Res. Bull., 2005, 40, 766–772 CrossRef CAS PubMed
. - G. Wang, A. N. Otuonye, E. A. Blair, K. Denton, Z. Tao and T. Asefa, J. Solid State Chem., 2009, 182, 1649–1660 CrossRef CAS PubMed
. - M. Vallet-Regí, J. C. Doadrio, A. L. Doadrio, I. Izquierdo-Barba and J. Pérez-Pariente, Solid State Ionics, 2004, 172, 435–439 CrossRef PubMed
. - A. Rámila, B. Muñoz, J. Pérez-Pariente and M. Vallet-Regí, J. Sol-Gel Sci. Technol., 2003, 26, 1199–1202 CrossRef
. - M. Otsuka, K. Tokumitsu and Y. Matsuda, J. Controlled Release, 2000, 67, 369–384 CrossRef CAS
. - J. V. Jokerst, T. Lobovkina, R. N. Zare and S. S. Gambhir, Nanomedicine, 2011, 6, 715–728 CrossRef CAS PubMed
. - L.-S. Wang, L.-C. Wu, S.-Y. Lu, L.-L. Chang, I. T. Teng, C.-M. Yang and J.-A. A. Ho, ACS Nano, 2010, 4, 4371–4379 CrossRef CAS PubMed
. - C.-P. Tsai, C.-Y. Chen, Y. Hung, F.-H. Chang and C.-Y. Mou, J. Mater. Chem., 2009, 19, 5737–5743 RSC
. - A. K. Varkouhi, M. Scholte, G. Storm and H. J. Haisma, J. Controlled Release, 2011, 151, 220–228 CrossRef CAS PubMed
. - M. Neu, D. Fischer and T. Kissel, J. Gene Med., 2005, 7, 992–1009 CrossRef CAS PubMed
. - S. R. Blumen, K. Cheng, M. E. Ramos-Nino, D. J. Taatjes, D. J. Weiss, C. C. Landry and B. T. Mossman, Am. J. Respir. Cell Mol. Biol., 2007, 36(3), 333–342 CrossRef CAS PubMed
. - Y.-Z. You, K. K. Kalebaila, S. L. Brock and D. Oupickỳ, Chem. Mater., 2008, 20, 3354–3359 CrossRef CAS
. - Q. Fu, G. V. R. Rao, L. K. Ista, Y. Wu, B. P. Andrzejewski, L. A. Sklar, T. L. Ward and G. P. López, Adv. Mater., 2003, 15, 1262–1266 CrossRef CAS
. - B. G. Trewyn, I. I. Slowing, S. Giri, H.-T. Chen and V. S. Y. Lin, Acc. Chem. Res., 2007, 40, 846–853 CrossRef CAS PubMed
. - S. Angelos, Y.-W. Yang, N. M. Khashab, J. F. Stoddart and J. I. Zink, J. Am. Chem. Soc., 2009, 131, 11344–11346 CrossRef CAS PubMed
. - E. Aznar, M. D. Marcos, R. n. Martínez-Máñez, F. l. Sancenón, J. Soto, P. Amorós and C. Guillem, J. Am. Chem. Soc., 2009, 131, 6833–6843 CrossRef CAS PubMed
. - K. Patel, S. Angelos, W. R. Dichtel, A. Coskun, Y.-W. Yang, J. I. Zink and J. F. Stoddart, J. Am. Chem. Soc., 2008, 130, 2382–2383 CrossRef CAS PubMed
. - C. Coll, L. Mondragón, R. Martínez-Máñez, F. Sancenón, M. D. Marcos, J. Soto, P. Amorós and E. Pérez-Payá, Angew. Chem., Int. Ed., 2011, 50, 2138–2140 CrossRef CAS PubMed
. - P. D. Thornton and A. Heise, J. Am. Chem. Soc., 2010, 132, 2024–2028 CrossRef CAS PubMed
. - Y. Zhao, B. G. Trewyn, I. I. Slowing and V. S. Y. Lin, J. Am. Chem. Soc., 2009, 131, 8398–8400 CrossRef CAS PubMed
. - E. Climent, R. Martínez-Máñez, F. Sancenón, M. D. Marcos, J. Soto, A. Maquieira and P. Amorós, Angew. Chem., Int. Ed., 2010, 49, 7281–7283 CrossRef CAS PubMed
. - V. C. Özalp and T. Schäfer, Chem. - Eur. J., 2011, 17, 9893–9896 CrossRef PubMed
. - X. Li, L. Zhang, X. Dong, J. Liang and J. Shi, Microporous Mesoporous Mater., 2007, 102, 151–158 CrossRef CAS PubMed
. - C. Barbé, J. Bartlett, L. Kong, K. Finnie, H. Q. Lin, M. Larkin, S. Calleja, A. Bush and G. Calleja, Adv. Mater., 2004, 16, 1959–1966 CrossRef
. - I. Roy, T. Y. Ohulchanskyy, H. E. Pudavar, E. J. Bergey, A. R. Oseroff, J. Morgan, T. J. Dougherty and P. N. Prasad, J. Am. Chem. Soc., 2003, 125, 7860–7865 CrossRef CAS PubMed
. - S. Radin, G. El-Bassyouni, E. J. Vresilovic, E. Schepers and P. Ducheyne, Biomaterials, 2005, 26, 1043–1052 CrossRef CAS PubMed
. - Y.-H. Son, M. Park, Y. B. Choy, H. R. Choi, D. S. Kim, K. C. Park and J.-H. Choy, Chem. Commun., 2007, 2799–2801 RSC
. - J. Lu, M. Liong, J. I. Zink and F. Tamanoi, Small, 2007, 3, 1341–1346 CrossRef CAS PubMed
. - I. S. Carino, L. Pasqua, F. Testa, R. Aiello, F. Puoci, F. Iemma and N. Picci, Drug Delivery, 2007, 14, 491–495 CrossRef CAS PubMed
. - H. Vallhov, S. Gabrielsson, M. Strømme, A. Scheynius and A. E. Garcia-Bennett, Nano Lett., 2007, 7, 3576–3582 CrossRef CAS PubMed
. - S. P. Hudson, R. F. Padera, R. Langer and D. S. Kohane, Biomaterials, 2008, 29, 4045–4055 CrossRef CAS PubMed
. - G. N. Kaluderovic, D. Perez-Quintanilla, Z. Zizak, Z. D. Juranic and S. Gomez-Ruiz, Dalton Trans., 2010, 39, 2597–2608 RSC
.
|
This journal is © The Royal Society of Chemistry 2014 |