Doping two-dimensional materials: ultra-sensitive sensors, band gap tuning and ferromagnetic monolayers
Received
25th October 2016
, Accepted 9th December 2016
First published on 9th December 2016
Abstract
The successful isolation of graphene from graphite in 2004 opened up new avenues to study two-dimensional (2D) systems from layered materials. Since then, research on 2D materials, including graphene, hexagonal-BN (h-BN), transition metal dichalcogenides (TMDs) and black phosphorous, has been extensive, thus leading to various possible applications in the fields of optoelectronics, biomedicine, spintronics, electrochemistry, energy storage and catalysis. However, certain barriers still need to be overcome when dealing with real applications, such as graphene's lack of a bandgap, restricting its use in semiconductor electronics. In this context, a possible solution is to tailor the electronic and optical properties of 2D materials by introducing defects or elemental doping. Although defects play a major role in modifying materials properties, the fact that we call them “defects” might have a negative impact. There has been a long debate on whether structurally perfect materials are equally relevant for modifying the properties and for applications. In this focus article, we clarify that although extra large amounts of defects could be detrimental to the materials properties, well-designed defects might lead to unprecedented properties and interesting applications that pristine materials do not have. Given the relatively short history of research on doped 2D layered materials, our objective is to answer and clarify the following fundamental questions: why does nanomaterial doping offer improved physico-chemical properties? What new applications arise from doping? And what are the current challenges along this line?
1. Introduction
Nowadays, the most common material used in the semiconductor industry is crystalline silicon, which takes most of the share of integrated circuits (ICs), liquid crystal displays (LCDs) and solar cells. Silicon, with a moderate band gap of 1.11 eV at room temperature and a Fermi level modulated by extrinsic dopant concentration, is qualified as the building block of directional or gate-controlled conducting devices, i.e. logic devices in digital electronics. Over the past few decades, the desire for constructing denser logic components in integrated circuits has driven the down-sizing of semiconductor transistors. However, there are inevitable difficulties when scaling down Si semiconductors, such as the detrimental scattering caused by dangling bonds, large subthreshold leakage and the diffraction limit of lithography instruments. Thus, two-dimensional (2D) materials, including graphene and transition metal dichalcogenides (TMDs), are brought in as the candidates for future electronics.1–3
2D materials consist of covalently bonded single-layered atoms, and they are therefore ultra-thin and smooth without dangling bonds. It has been proposed that ultra-thin transistors, highly-responsive sensors and spintronic devices will be enabled by the unique physical and chemical properties of atomically thin 2D materials.4 Since the pioneering work on mechanical exfoliation of single-layered graphene and graphene field effect transistors in 2004,5 fascinating properties of 2D materials have been revealed,1,6–10 and enormous effort has been devoted to exploit their unique electronic structure to create novel nanostructures and devices.1,3 A few years later, semiconducting TMDs were put forward as transistor channel materials, with band gaps ranging from 1.0 to 2.0 eV and similar electronic band gaps to bulk semiconductors such as Si and GaAs.2,4,8,11–13
However, the band gap and related electronic structures of 2D materials still need to be tailored in order to implement specific functionalities.14,15 For example, the zero band gap of pristine graphene makes it less desirable for conventional transistors. Inspired by the Si-based electronics, the idea of defect engineering and doping plays a key role in controlling the properties of 2D materials and related devices.14,16–18 For example, doping graphene with heteroatoms (e.g. B, N, Si) is a feasible way to open up a band gap and alter the Fermi level, in order to further modulate the behavior of graphene-based devices.16,17 For instance, defects in doped graphene have resulted in a significant enhancement of the interactions with small molecules, thus boosting the performance of gas sensors as well as Raman scattering.19–21 Likewise, it is also possible to dope TMDs in order to gain n- or p-type semiconducting behavior, and further create in-plane or vertical TMD hetero-junctions, which is analogous to the p–n junction of bulk Si.22–25 Furthermore, defects in TMDs can generate ferromagnetism, thus opening up new avenues for spintronics and valleytronics.26–28
This Focus Article reviews the state-of-the-art progress in the theory, synthesis and functionality of doped 2D materials. We choose a few examples, among many others, to highlight the possibility of enhancing the device performance or benefiting materials' properties by means of defect engineering. We summarize recent reports on: (1) molecular sensing applications of doped graphene, (2) ferromagnetic TMDs synthesized by Mn doping, and (3) doping and constructing 2D heterostructures by TMDs. The final section of this article provides an electronic/optical perspective of applications of 2D materials and some of the challenges that need to be addressed in the near future. By providing these examples, we have demonstrated that imperfect materials are unique and useful, thus allowing scientists to tailor their properties using a large number of combinations and stoichiometries that modern engineering devices require. More comprehensive reviews about recent achievements and challenges regarding doping of 2D materials can be found in recent literature.15–18,29–34
2. Sensor applications of doped graphene
As discussed above, chemical doping could modify the atomic structure of graphene sheets, which could tune their electronic and chemical properties. Based on their unique properties, novel applications have recently been demonstrated, including photo-catalysis,35–40 energy conversion and storage,41–45 field-effect transistors (FET),46–48 superconductors,49 lithium ion batteries50,51 and chemical/biosensors.52 In particular, graphene-based sensors have become highly attractive, as they provide an efficient way to detect trace amounts of molecules (e.g. parts per trillion), thus enabling applications in homeland security, virus detection, and food and environmental safety.
Due to the chemical inertness of pristine graphene (PG), the interaction between PG and the probe molecules is usually very weak. However, substitutionally doped graphene using non-carbon atoms exhibits increased chemical reactivity and could also enhance the biocompatibility of graphene. Based on these findings, Wang et al. demonstrated electrochemical biosensing using N-doped graphene (NG; see Fig. 1(A)). For ascorbic acid (AA) or uric acid (UA), NG could be used for glucose biosensing with concentrations as low as 0.01 mM. The improved selectivity and sensitivity of NG are associated with its excellent biocompatibility53,54 and the fast direct electron transfer for glucose oxidase.55 Besides biosensing applications, doped graphene has also been used as a gas sensor. In this context, Lv et al. synthesized B-doped graphene (BG) and demonstrated for the first time that BG exhibits unique sensing capabilities when detecting toxic gases such as NO2 and NH3.21 The detection limit for the as-synthesized BG can reach as low as 95 ppt for NO2 gas and 60 ppb for NH3 gas (see Fig. 1(B)). It is also noteworthy that when comparing the sensitivity of BG with PG, B-doped graphene has enhanced sensitivity values of 27 and 105 times better for NO2 and NH3 detection. This result is attributed to the presence of B atoms within the graphene lattice that results in high affinity to both donor and acceptor molecules, thus leading to stronger adsorption or interactions between the gas molecules and the graphene surface.
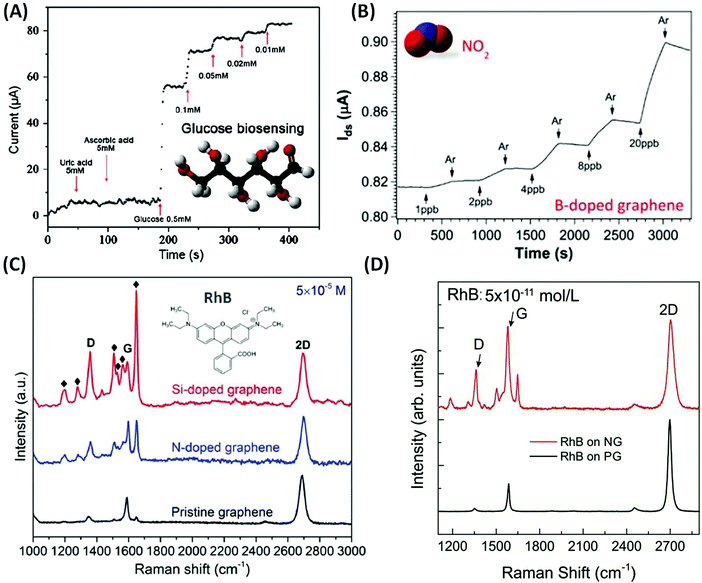 |
| Fig. 1 Different sensor applications of doped graphene. (A) Chronoamperometric responses to successive addition of uric acid, ascorbic acid, and glucose on the NG electrode.55 Inset of (A) shows the molecular structure of glucose. (B) Gas sensing properties of the as-synthesized B-doped graphene when different concentrations of NO2 are applied.21 Inset of (B) shows the molecular structure of NO2. (C) Comparison of Raman enhancement effect when RhB molecules are applied on top of pristine, N-doped and Si-doped graphene, respectively,19 Inset of (C) shows the molecular structure of RhB. (D) Raman spectrum of RhB with concentration as low as 5 × 10−11 mol L−1 deposited on top of NG and PG. It could be observed that NG sheets can still detect such low concentrations of molecules.57 | |
Another possible application of graphene is its use as a substrate to enhance Raman signals for specific molecules: graphene enhanced Raman scattering (GERS).56 Lv et al. synthesized NG and Si-doped graphene (SiG) and demonstrated that the introduction of nitrogen or silicon atoms into the graphene sub-lattice could significantly enhance the Raman signal of certain fluorescent molecules, such as rhodamine B (RhB), crystal violet (CRV) and methylamine blue (MB). SiG exhibits an enhancement factor 10–40 times higher than that of PG (see Fig. 1(C)).19,20 More recently, Feng et al. showed that by using NG as a substrate, Raman fingerprints of specific dye molecules can be detected at a record low concentration of 10−11 M (see Fig. 1(D)). It is estimated that there are only around 100 molecules within the laser spot area (∼1 μm2) at such low concentration and this is very close to single molecule detection. The authors further demonstrated that the enhanced Raman sensing requires the Fermi energy of the substrate to match the LUMO level of the probe molecule, which allows an effective charge-transfer excitation.57
Other sensing applications of doped graphene, such as detection of acids58 and oxides,59,60 have also been explored experimentally and theoretically. Such studies indicate that graphene sensors would be extremely sensitive, ultrafast, and exhibit reduced size with high reliability for a wide range of applications.
3. Ferromagnetic doping of TMD
It has been demonstrated in the previous section how doped graphene could dramatically change its original properties, being beneficial for various sensor applications. However, another group of 2D materials, transition metal dichalcogenides (TMDs), such as molybdenum disulfide monolayers, have recently attracted researchers' attention as new direct bandgap semiconductors, and studies of these materials have been intensified, involving optoelectronics, spintronics and catalysis. Similar to graphene, the modification of TMDs could improve their performance and hence extend their applications. One example, among all other possibilities, is trying to control and tune the magnetic properties of TMDs. The ferromagnetism of MoS2 has recently been an attractive topic.61,62 The magnetic ordering arising from defects such as edges and vacancies has been studied in detail.27,28,63–69 A possible way to achieve ferromagnetism is by introducing heteroatoms into TMD monolayers. In this respect, substitutional doping of the transition metal sites has been considered theoretically.70–75 For example, theoretical studies show that the magnetic properties of Fe-doped MoS2 depend largely on the number of layers, transitioned from monolayer-ferromagnetic to bi- and tri-layer-antiferromagnetic.29 Theory also predicted that Co-doped MoS2 will generate strong antiferromagnetic coupling between cobalt atoms, and it is considered a promising candidate as a dilute magnetic semiconductor.70 Another candidate to achieve dilute magnetic semiconductors is Mn, as magnetic properties arise upon the inclusion of Mn into MoS2 and was predicted by different groups.70,76 Although the calculated Curie temperature and magnetic ordering are found to be sensitive to the doping elements and the theory employed, the possibility of reaching room temperature ferromagnetism is triggering increasing interest among experimentalists and it is likely that in the near future some ferromagnetic TMDs would be synthesized.
A chemical vapor deposition (CVD) method has been used to grow MoS2 monolayers, and Mn could be incorporated into the lattice (Fig. 2).77 A fundamental distinction between CVD and DFT calculation is that the former always involves a growth substrate while the latter normally deals with freestanding sheets. However, the choice of the growth substrate is critical for achieving Mn lattice doping. Around two atomic percent of Mn has been incorporated into monolayers of MoS2, preferentially on the edges of the sheets or at grain boundaries, when graphene is used as a substrate.77 However, the common substrate (e.g. SiO2) unfortunately reacts with Mn, and is therefore not suitable for the growth of doped MoS2 monolayers.77 An assumption made when constructing a model for Mn doped MoS2 is that the crystal structure of MoS2 does not change. However, experimental work confirmed that this assumption only holds below a doping threshold level within the current growth techniques.77 Although the study of doping induced ferromagnetism is still in its infancy, the successful doping of Mn into 2D materials has triggered us to develop novel synthesis techniques and achieve doping of TMDs with other elements (e.g. Fe, Co, Ni, Cu). It has also highlighted the importance of bringing together theorists, material scientists, and surface chemists to tackle this challenging subject. As these new materials will possess both semiconducting and ferromagnetic properties, it is believed that the application of these hybrid semiconductor–ferromagnet systems will revolutionize the performance of various devices, including flash memory devices and single-photon emitters. The possible magneto-transport and magneto-optical effects could also allow us to use them as magnetic field sensors, magneto-optical devices or even all-magnetic logic, which exhibit low power consumption and radiation hardness.78 All of these possible applications will open up new avenues for both magnetic and semiconducting industries.
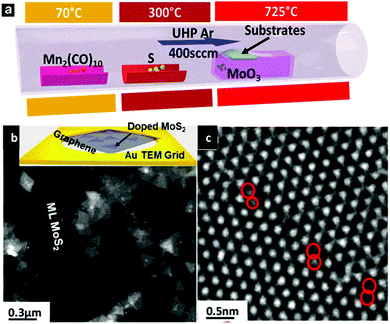 |
| Fig. 2 (a) Schematics of the vapor deposition setup used to synthesize Mn doped MoS2 monolayers. Mn-doped MoS2 can be directly grown on a graphene covered TEM grid. (b) Low magnification TEM image showing the doped MoS2 triangular domains. Inset of (b) shows an image of directly grown Mn doped MoS2 triangles on a graphene covered TEM grid. (c) HRTEM image showing the preferred location of Mn dopants along grain boundaries.77 | |
4. van der Waals heterostructures: synthesis and applications
The previous sections have demonstrated that doping could be beneficial to the properties and applications of 2D materials, including graphene and TMDs. As the family of 2D materials is large and ranges from a zero-gap semimetal (graphene) to metals (NbSe2), semiconductors (n-type MoS2 and p-type WSe2) and insulators (h-BN), fascinating possibilities by constructing new layer-by-layer solids could be achieved by using these layers as building blocks.5,73,79–85 For example, when these layers are stacked vertically, or “atomically stitched” in-plane,86–90 their properties could change dramatically at the interface,91 thus enabling unprecedented properties and applications in nanoelectronics, including photodetectors,86,92–94 high performance field-effect-transistors,71,90,95–97 and solar cells.22,98–100 In this section, we will briefly summarize the recent achievements in the synthesis and applications of van der Waals heterostructures or van der Waals solids.
It is noteworthy that 2D materials offer a full library of bandgaps: graphene has zero bandgap; semiconducting TMDs exhibit bandgaps typically in the visible range, while boron nitride is an insulator with a large band gap of ∼6.0 eV. Based on these properties, it is possible to tune the bandgap of materials by synthesizing 2D alloys or sharp heterostructures. In this context, Gong et al. developed a single-step synthesis method to grow MoS2(1−x)Se2x monolayers with tunable compositions.101 By tuning the growth parameters, a controlled selenium doping level could be achieved, and the resulting optical bandgap could be continuously modified between 1.85 and 1.60 eV (see Fig. 3(a)). The correlation between Se doping concentration and the shift of the optical bandgap agrees with local-density approximation (LDA) bandgap calculations. In addition to chalcogen alloys, Lin et al. obtained alloys of MoxW1−xS2 by transition metal doping.14 An in-plane stoichiometric gradient and a continuous shift of the optical bandgap could be observed from Raman and PL measurements (see Fig. 3(b)). It is noteworthy that this continuously tunable direct bandgap exists within the same monocrystalline domain. Both studies on controlling over the band structure of the material pave the way for future applications in electronics and optoelectronics.
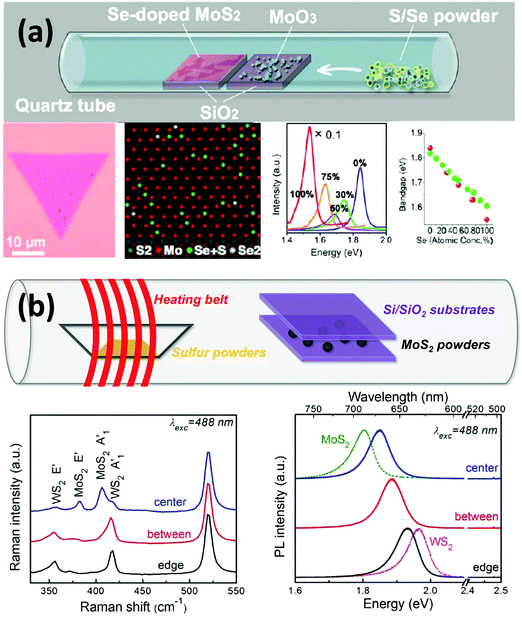 |
| Fig. 3 Synthesis and characterization of various 2D material alloys showing the possibility of tuning the optical bandgap. (a) Se-doped MoS2 monolayer triangle. HRTEM images show Se atoms in the MoS2 sublattice. PL measurements and theoretical simulation demonstrate that the optical bandgap of the material varies according to the Se doping rate.101 (b) Synthesis of WxMo1−xS2 alloys in which Mo atoms are more concentrated in the center while the W atoms are more concentrated around the edge of the triangles, resulting in the tunability of the optical bandgap within the same sample.14 | |
Heterostructures with functional interfaces can be applied in modern solid-state devices. Lin et al. demonstrated the direct growth of MoS2, WSe2 and h-BN on epitaxial graphene to form large area van der Waals heterostructures.102 They revealed that the direct synthesis of TMDs on epitaxial graphene provides atomically sharp interfaces (see Fig. 4(a)). By fabricating FET devices, they observed that the graphene substrate does not shorten the channel. Instead, the direct growth of MoS2 on epitaxial graphene leads to a 103 improvement in photoresponse compared to MoS2 alone. Gong et al. developed a one-step growth strategy for the synthesis of vertically stacked as well as in-plane heterostructures composed of WS2/MoS2 with atomically clean and sharp interfaces (see Fig. 4(b)), which show locally enhanced photoluminescence and can serve as p–n junctions as evidenced by a photovoltaic effect.22
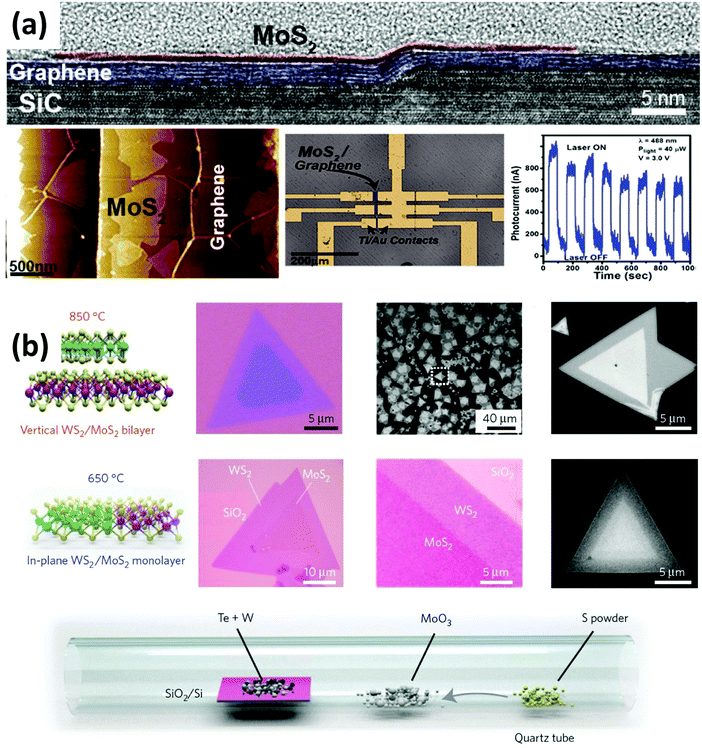 |
| Fig. 4 Synthesis and characterization of various 2D materials heterostructures. (a) Direct growth of MoS2 on top of epitaxial graphene. Cross-section TEM images indicate the sharp interface between graphene and MoS2. FET fabrication measurements demonstrate that the heterostructure performs 1000 times better in photoresponse than the MoS2 sample alone.102 (b) One-step synthesis of WS2–MoS2 heterostructures. By controlling the synthesis temperature, a vertical (high temperature) and lateral (low temperature) heterostructure with atomically clean and sharp interfaces could be synthesized.22 | |
The synthesis and studies on other 2D material heterostructures, such as WSe2–MoSe2,88–90 MoSe2–WS2,103 WSe2–MoS2100 and graphene–h-BN87 were recently reported and various properties and applications have been discovered. One of the biggest challenges in synthesizing heterostructures lies within the fact that direct growth favors the thermodynamically preferred TMD alloys and the interface regions for these lateral junctions are likely to be alloy structures. However, recently reported two-step epitaxial methods might solve this problem.79 With the progress in synthesizing techniques, it could be predicted that by combining both vertical and lateral 2D heterostructures, one would create novel architectures (such as monolayer p–n diodes, light-emitting diodes and bipolar junction transistors) in the semiconducting industry with unprecedented properties.
5. Conclusions and perspective
Recent achievements regarding doped 2D materials and their potential applications were presented. We have demonstrated that dopants could indeed tailor the electrical, optical, magnetic and chemical properties of 2D systems that could lead to diverse and attractive applications such as ultrasensitive molecular sensors, enhanced FET transistors and ferromagnetic functionalities.
Although doping provides novel properties in 2D materials, there are plenty of challenges ahead that need to be addressed. For example, it is still challenging to obtain 2D monocrystals with uniform/homogeneous doping using traditional synthesis methods. Therefore, innovative non-equilibrium synthetic methods need to be proposed and developed in order to achieve controllable doping in 2D lattices. In addition, the dynamics of local dopants are largely unexplored, and these could possibly lead to a better understanding of how dopants affect the physical and chemical properties of materials.
The incorporation of large atoms (e.g. P, S) into graphene is difficult, since the large size of the dopants causes steric hindrance and distortions of the graphene lattice. Another challenge is how to achieve unbalanced doping within sublattices of graphene. This could potentially open a bandgap in graphene which could lead to novel optical and electronic applications.16 However, a major obstacle in doped TMDs, such as MoS2 and WS2, is to prevent the formation of stacked 3D ordering. For example, high level Mn doping into MoS2 will trigger the possibility of forming MnS 3D structures. Doping TMDs with other elements such as Fe, Co and Ni is only achieved by hydrothermal or colloidal synthesis methods, because it is very difficult to control the number of layers and doping sites.18 Another big challenge affecting the ferromagnetic applications of doped TMDs is to maintain the crystalline structure of TMDs while having a decent amount of element doping. Only around 5% Mn doping could be achieved in doped MoS277 and there are reports that higher than 20% doping of Cr into TMDs will create significant disorder in the crystal lattice.104 Unfortunately, only a large amount of doping could be detected using a superconducting quantum interference device (SQUID). It is therefore clear that the field of doped 2D materials is still in its infancy, and joint efforts from physicists, chemists, materials scientists and engineers are required to move forward in this fascinating and emerging field.
Acknowledgements
S. F., Z. L., X. G., and M. T. acknowledge the financial support from the U.S. Army Research Office under MURI grant (W911NF-11-1-0362) and the National Science Foundation (2DARE-EFRI-1433311). R. L. acknowledges the support from the National Natural Science Foundation of China (Grant No. 51372131), the 973 program of China (No. 2014CB932401, 2015CB932500) and the Tsinghua University Initiative Scientific Research Program.
References
- A. H. Castro Neto, F. Guinea, N. M. R. Peres, K. S. Novoselov and A. K. Geim, Rev. Mod. Phys., 2009, 81, 109–162 CrossRef CAS.
- M. Chhowalla, H. S. Shin, G. Eda, L. J. Li, K. P. Loh and H. Zhang, Nat. Chem., 2013, 5, 263–275 CrossRef PubMed.
- A. K. Geim and K. S. Novoselov, Nat. Mater., 2007, 6, 183–191 CrossRef CAS PubMed.
- M. Chhowalla, D. Jena and H. Zhang, Nat. Rev. Mater., 2016, 1, 16052 CrossRef.
- K. S. Novoselov, A. K. Geim, S. V. Morozov, D. Jiang, Y. Zhang, S. V. Dubonos, I. V. Grigorieva and A. A. Firsov, Science, 2004, 306, 666–669 CrossRef CAS PubMed.
- A. A. Balandin, S. Ghosh, W. Bao, I. Calizo, D. Teweldebrhan, F. Miao and C. N. Lau, Nano Lett., 2008, 8, 902–907 CrossRef CAS PubMed.
- R. Nair, P. Blake, A. Grigorenko, K. Novoselov, T. Booth, T. Stauber, N. Peres and A. Geim, Science, 2008, 320, 1308 CrossRef CAS PubMed.
- K. S. Novoselov, D. Jiang, F. Schedin, T. J. Booth, V. V. Khotkevich, S. V. Morozov and A. K. Geim, Proc. Natl. Acad. Sci. U. S. A., 2005, 102, 10451–10453 CrossRef CAS PubMed.
- K. S. Novoselov, Z. Jiang, Y. Zhang, S. Morozov, H. Stormer, U. Zeitler, J. Maan, G. Boebinger, P. Kim and A. Geim, Science, 2007, 315, 1379 CrossRef CAS PubMed.
- Y.-W. Son, M. L. Cohen and S. G. Louie, Phys. Rev. Lett., 2006, 97, 216803 CrossRef PubMed.
- S. Das, J. A. Robinson, M. Dubey, H. Terrones and M. Terrones, Annu. Rev. Mater. Res., 2015, 45, 1–27 CrossRef CAS.
- B. Radisavljevic, A. Radenovic, J. Brivio, V. Giacometti and A. Kis, Nat. Nanotechnol., 2011, 6, 147–150 CrossRef CAS PubMed.
- A. Splendiani, L. Sun, Y. Zhang, T. Li, J. Kim, C.-Y. Chim, G. Galli and F. Wang, Nano Lett., 2010, 10, 1271–1275 CrossRef CAS PubMed.
- Z. Lin, M. T. Thee, A. L. Elias, S. M. Feng, C. J. Zhou, K. Fujisawa, N. Perea-Lopez, V. Carozo, H. Terrones and M. Terrones, APL Mater., 2014, 2, 092514 CrossRef.
- H. Terrones, R. Lv, M. Terrones and M. S. Dresselhaus, Rep. Prog. Phys., 2012, 75, 062501 CrossRef PubMed.
-
F. López-Urías, R. Lv, H. Terrones and M. Terrones, in Graphene Chemistry: Theoretical Perspectives, ed. D.-E. Jang and Z. Chen, John Wiley & Sons, Ltd, Chichester, UK, 2013, pp. 183–207 Search PubMed.
- R. T. Lv and M. Terrones, Mater. Lett., 2012, 78, 209–218 CrossRef CAS.
- A. A. Tedstone, D. J. Lewis and P. O’Brien, Chem. Mater., 2016, 28, 1965–1974 CrossRef CAS.
- R. Lv, M. C. dos Santos, C. Antonelli, S. M. Feng, K. Fujisawa, A. Berkdemir, R. Cruz-Silva, A. L. Elias, N. Perea-Lopez, F. Lopez-Urias, H. Terrones and M. Terrones, Adv. Mater., 2014, 26, 7593–7599 CrossRef CAS PubMed.
- R. Lv, Q. Li, A. R. Botello-Mendez, T. Hayashi, B. Wang, A. Berkdemir, Q. Hao, A. L. Elias, R. Cruz-Silva, H. R. Gutierrez, Y. A. Kim, H. Muramatsu, J. Zhu, M. Endo, H. Terrones, J. C. Charlier, M. Pan and M. Terrones, Sci. Rep., 2012, 2, 586 Search PubMed.
- R. T. Lv, G. G. Chen, Q. Li, A. McCreary, A. Botello-Mendez, S. V. Morozov, L. B. Liang, X. Declerck, N. Perea-Lopez, D. A. Culleni, S. M. Feng, A. L. Elias, R. Cruz-Silva, K. Fujisawa, M. Endo, F. Y. Kang, J. C. Charlier, V. Meunier, M. H. Pan, A. R. Harutyunyan, K. S. Novoselov and M. Terrones, Proc. Natl. Acad. Sci. U. S. A., 2015, 112, 14527–14532 CrossRef CAS PubMed.
- Y. J. Gong, J. H. Lin, X. L. Wang, G. Shi, S. D. Lei, Z. Lin, X. L. Zou, G. L. Ye, R. Vajtai, B. I. Yakobson, H. Terrones, M. Terrones, B. K. Tay, J. Lou, S. T. Pantelides, Z. Liu, W. Zhou and P. M. Ajayan, Nat. Mater., 2014, 13, 1135–1142 CrossRef CAS PubMed.
- L. Hao, Y. Liu, W. Gao, Z. Han, Q. Xue, H. Zeng, Z. Wu, J. Zhu and W. Zhang, J. Appl. Phys., 2015, 117, 114502 CrossRef.
- H. Ilatikhameneh, Y. Tan, B. Novakovic, G. Klimeck, R. Rahman and J. Appenzeller, IEEE J. Explor. Solid-State Comput. Solid-State Comput. Devices Circuts, 2015, 1, 12–18 Search PubMed.
- Y. Jin, D. H. Keum, S.-J. An, J. Kim, H. S. Lee and Y. H. Lee, Adv. Mater., 2015, 27, 5534–5540 CrossRef CAS PubMed.
- L. Cai, J. He, Q. Liu, T. Yao, L. Chen, W. Yan, F. Hu, Y. Jiang, Y. Zhao and T. Hu, J. Am. Chem. Soc., 2015, 137, 2622–2627 CrossRef CAS PubMed.
- N. J. Huo, Y. Li, J. Kang, R. X. Li, Q. L. Xia and J. B. Li, Appl. Phys. Lett., 2014, 104, 202406 CrossRef.
- S. Mathew, K. Gopinadhan, T. K. Chan, X. J. Yu, D. Zhan, L. Cao, A. Rusydi, M. B. H. Breese, S. Dhar, Z. X. Shen, T. Venkatesan and J. T. L. Thong, Appl. Phys. Lett., 2012, 101, 102103 CrossRef.
- G. R. Bhimanapati, Z. Lin, V. Meunier, Y. Jung, J. Cha, S. Das, D. Xiao, Y. Son, M. S. Strano, V. R. Cooper, L. Liang, S. G. Louie, E. Ringe, W. Zhou, S. S. Kim, R. R. Naik, B. G. Scumpter, H. Terrones, F. Xia, Y. Wang, J. Zhu, D. Akinwande, N. Alem, J. A. Schuller, R. E. Schaak, M. Terrones and J. A. Robinson, ACS Nano, 2015, 9, 11509–11539 CrossRef CAS PubMed.
- Z. Lin, B. R. Carvalho, E. Kahn, R. Lv, R. Rao, H. Terrones, M. A. Pimenta and M. Terrones, 2D Mater., 2016, 3, 022002 CrossRef.
- R. Lv, J. A. Robinson, R. E. Schaak, D. Sun, Y. Sun, T. E. Mallouk and M. Terrones, Acc. Chem. Res., 2014, 48, 56–64 CrossRef PubMed.
- R. Lv, H. Terrones, A. L. Elías, N. Perea-López, H. R. Gutiérrez, E. Cruz-Silva, L. P. Rajukumar, M. S. Dresselhaus and M. Terrones, Nano Today, 2015, 10, 559–592 CrossRef CAS.
- K. Novoselov, A. Mishchenko, A. Carvalho and A. C. Neto, Science, 2016, 353, aac9439 CrossRef CAS PubMed.
- Q. Zeng, H. Wang, W. Fu, Y. Gong, W. Zhou, P. M. Ajayan, J. Lou and Z. Liu, Small, 2015, 11, 1868–1884 CrossRef CAS PubMed.
- B. Han, S. Q. Liu, Z. R. Tang and Y. J. Xu, J. Energy Chem., 2015, 24, 145–156 CrossRef.
- Y. Hou, Z. H. Wen, S. M. Cui, X. R. Guo and J. H. Chen, Adv. Mater., 2013, 25, 6291–6297 CrossRef CAS PubMed.
- L. Jia, D. H. Wang, Y. X. Huang, A. W. Xu and H. Q. Yu, J. Phys. Chem. C, 2011, 115, 11466–11473 CAS.
- F. K. Meng, J. T. Li, S. K. Cushing, M. J. Zhi and N. Q. Wu, J. Am. Chem. Soc., 2013, 135, 10286–10289 CrossRef CAS PubMed.
- Z. G. Mou, Y. J. Wu, J. H. Sun, P. Yang, Y. K. Du and C. Lu, ACS Appl. Mater. Interfaces, 2014, 6, 13798–13806 CAS.
- D. Usachov, O. Vilkov, A. Gruneis, D. Haberer, A. Fedorov, V. K. Adamchuk, A. B. Preobrajenski, P. Dudin, A. Barinov, M. Oehzelt, C. Laubschat and D. V. Vyalikh, Nano Lett., 2011, 11, 5401–5407 CrossRef CAS PubMed.
- D. Higgins, P. Zamani, A. Yu and Z. Chen, Energy Environ. Sci., 2016, 9, 357–390 CAS.
- Y. Jiao, Y. Zheng, M. T. Jaroniec and S. Z. Qiao, Chem. Soc. Rev., 2015, 44, 2060–2086 RSC.
- F. Bonaccorso, L. Colombo, G. H. Yu, M. Stoller, V. Tozzini, A. C. Ferrari, R. S. Ruoff and V. Pellegrini, Science, 2015, 347, 41 CrossRef CAS PubMed.
- J. J. Duan, S. Chen, M. Jaroniec and S. Z. Qiao, ACS Catal., 2015, 5, 5207–5234 CrossRef CAS.
- R. Raccichini, A. Varzi, S. Passerini and B. Scrosati, Nat. Mater., 2015, 14, 271–279 CrossRef CAS PubMed.
- P. Solis-Fernandez, S. Okada, T. Sato, M. Tsuji and H. Ago, ACS Nano, 2016, 10, 2930–2939 CrossRef CAS PubMed.
- X. Y. Li, T. Tang, M. Li and X. C. He, Appl. Phys. Lett., 2015, 106, 013110 CrossRef.
- J. S. Kim, B. J. Kim, Y. J. Choi, M. H. Lee, M. S. Kang and J. H. Cho, Adv. Mater., 2016, 28, 4803–4810 CrossRef CAS PubMed.
- J. Chapman, Y. Su, C. A. Howard, D. Kundys, A. N. Grigorenko, F. Guinea, A. K. Geim, I. V. Grigorieva and R. R. Nair, Sci. Rep., 2016, 6, 23254 CrossRef CAS PubMed.
- X. F. Li, D. S. Geng, Y. Zhang, X. B. Meng, R. Y. Li and X. L. Sun, Electrochem. Commun., 2011, 13, 822–825 CrossRef CAS.
- A. L. M. Reddy, A. Srivastava, S. R. Gowda, H. Gullapalli, M. Dubey and P. M. Ajayan, ACS Nano, 2010, 4, 6337–6342 CrossRef CAS PubMed.
- J. Q. Liu, Z. Liu, C. J. Barrow and W. R. Yang, Anal. Chim. Acta, 2015, 859, 1–19 CrossRef CAS PubMed.
- A. L. Mihalchik, W. Ding, D. W. Porter, C. McLoughlin, D. Schwegler-Berry, J. D. Sisler, A. B. Stefaniak, B. N. Snyder-Talkington, R. Cruz-Silva and M. Terrones, Toxicology, 2015, 333, 25–36 CrossRef CAS PubMed.
- J. Carrero-Sanchez, A. Elias, R. Mancilla, G. Arrellin, H. Terrones, J. Laclette and M. Terrones, Nano Lett., 2006, 6, 1609–1616 CrossRef CAS PubMed.
- Y. Wang, Y. Y. Shao, D. W. Matson, J. H. Li and Y. H. Lin, ACS Nano, 2010, 4, 1790–1798 CrossRef CAS PubMed.
- X. Ling, L. Xie, Y. Fang, H. Xu, H. Zhang, J. Kong, M. S. Dresselhaus, J. Zhang and Z. Liu, Nano Lett., 2009, 10, 553–561 CrossRef PubMed.
- S. M. Feng, M. C. dos Santos, B. R. Carvalho, R. T. Lv, Q. Li, K. Fujisawa, A. L. Elias, Y. Lei, N. Perea-Lopez, M. Endo, M. H. Pan, M. A. Pimenta and M. Terrones, Sci. Adv., 2016, 2, e1600322 Search PubMed.
- Z. H. Sheng, X. Q. Zheng, J. Y. Xu, W. J. Bao, F. B. Wang and X. H. Xia, Biosens. Bioelectron., 2012, 34, 125–131 CrossRef CAS PubMed.
- Y. Zou, F. Li, Z. H. Zhu, M. W. Zhao, X. G. Xu and X. Y. Su, Eur. Phys. J. B, 2011, 81, 475–479 CrossRef CAS.
- Z. M. Ao, J. Yang, S. Li and Q. Jiang, Chem. Phys. Lett., 2008, 461, 276–279 CrossRef CAS.
-
P. Lee, Physics and chemistry of materials with layered structures: optical and electrical properties, 1976 Search PubMed.
- S. S. P. Parkin and R. H. Friend, Physica B+C, 1980, 99, 219–223 CrossRef CAS.
- A. R. Botello-Mendez, F. Lopez-Urias, M. Terrones and H. Terrones, Nanotechnology, 2009, 20, 325703 CrossRef CAS PubMed.
- Y. Li, Z. Zhou, S. Zhang and Z. Chen, J. Am. Chem. Soc., 2008, 130, 16739–16744 CrossRef CAS PubMed.
- H. Pan and Y. W. Zhang, J. Mater. Chem., 2012, 22, 7280–7290 RSC.
- S. Tongay, S. S. Varnoosfaderani, B. R. Appleton, J. Q. Wu and A. F. Hebard, Appl. Phys. Lett., 2012, 101, 123105 CrossRef.
- Z. Yang, D. Gao, J. Zhang, Q. Xu, S. Shi, K. Tao and D. Xue, Nanoscale, 2015, 7, 650–658 RSC.
- J. Zhang, J. M. Soon, K. P. Loh, J. Yin, J. Ding, M. B. Sullivian and P. Wu, Nano Lett., 2007, 7, 2370–2376 CrossRef CAS PubMed.
- Z. Zhang, X. Zou, V. H. Crespi and B. I. Yakobson, ACS Nano, 2013, 7, 10475–10481 CrossRef CAS PubMed.
- Y. Cheng, Z. Zhu, W. Mi, Z. Guo and U. Schwingenschlögl, Phys. Rev. B: Condens. Matter Mater. Phys., 2013, 87, 100401 CrossRef.
- C. R. Dean, A. F. Young, I. Meric, C. Lee, L. Wang, S. Sorgenfrei, K. Watanabe, T. Taniguchi, P. Kim, K. L. Shepard and J. Hone, Nat. Nanotechnol., 2010, 5, 722–726 CrossRef CAS PubMed.
- N. Feng, W. Mi, Y. Cheng, Z. Guo, U. Schwingenschlögl and H. Bai, Sci. Rep., 2014, 4, 3987 Search PubMed.
- X. Lin and J. Ni, J. Appl. Phys., 2014, 116, 044311 CrossRef.
- H. Shu, P. Luo, P. Liang, D. Cao and X. Chen, ACS Appl. Mater. Interfaces, 2015, 7, 7534–7541 CAS.
- W. S. Yun and J. Lee, Phys. Chem. Chem. Phys., 2014, 16, 8990–8996 RSC.
- A. Ramasubramaniam and D. Naveh, Phys. Rev. B: Condens. Matter Mater. Phys., 2013, 87, 195201 CrossRef.
- K. Zhang, S. Feng, J. Wang, A. Azcatl, N. Lu, R. Addou, N. Wang, C. Zhou, J. O. Lerach, V. Bojan, M. J. Kim, L.-Q. Chen, R. M. Wallace, M. Terrones, J. Zhu and J. A. Robinson, Nano Lett., 2015, 15, 6586 CrossRef CAS PubMed.
- T. Dietl, Nat. Mater., 2010, 9, 965–974 CrossRef CAS PubMed.
- Y. J. Gong, S. D. Lei, G. L. Ye, B. Li, Y. M. He, K. Keyshar, X. Zhang, Q. Z. Wang, J. Lou, Z. Liu, R. Vajtai, W. Zhou and P. M. Ajayan, Nano Lett., 2015, 15, 6135–6141 CrossRef CAS PubMed.
- H. R. Gutiérrez, N. Perea-López, A. L. Elías, A. Berkdemir, B. Wang, R. Lv, F. López-Urías, V. H. Crespi, H. Terrones and M. Terrones, Nano Lett., 2012, 13, 3447–3454 CrossRef PubMed.
- J.-K. Huang, J. Pu, C.-L. Hsu, M.-H. Chiu, Z.-Y. Juang, Y.-H. Chang, W.-H. Chang, Y. Iwasa, T. Takenobu and L.-J. Li, ACS Nano, 2013, 8, 923–930 CrossRef PubMed.
- Y. H. Lee, X. Q. Zhang, W. Zhang, M. T. Chang, C. T. Lin, K. D. Chang, Y. C. Yu, J. T. W. Wang, C. S. Chang and L. J. Li, Adv. Mater., 2012, 24, 2320–2325 CrossRef CAS PubMed.
- K. F. Mak, C. Lee, J. Hone, J. Shan and T. F. Heinz, Phys. Rev. Lett., 2010, 105, 136805 CrossRef PubMed.
- L. Song, L. Ci, H. Lu, P. B. Sorokin, C. Jin, J. Ni, A. G. Kvashnin, D. G. Kvashnin, J. Lou and B. I. Yakobson, Nano Lett., 2010, 10, 3209–3215 CrossRef CAS PubMed.
- P. Vogt, P. Capiod, M. Berthe, A. Resta, P. De Padova, T. Bruhn, G. Le Lay and B. Grandidier, Appl. Phys. Lett., 2014, 104, 021602 CrossRef.
- F. Withers, O. Del Pozo-Zamudio, A. Mishchenko, A. P. Rooney, A. Gholinia, K. Watanabe, T. Taniguchi, S. J. Haigh, A. K. Geim, A. I. Tartakovskii and K. S. Novoselov, Nat. Mater., 2015, 14, 301–306 CrossRef CAS PubMed.
- Z. Liu, L. L. Ma, G. Shi, W. Zhou, Y. J. Gong, S. D. Lei, X. B. Yang, J. N. Zhang, J. J. Yu, K. P. Hackenberg, A. Babakhani, J. C. Idrobo, R. Vajtai, J. Lou and P. M. Ajayan, Nat. Nanotechnol., 2013, 8, 119–124 CrossRef CAS PubMed.
- C. M. Huang, S. F. Wu, A. M. Sanchez, J. J. P. Peters, R. Beanland, J. S. Ross, P. Rivera, W. Yao, D. H. Cobden and X. D. Xu, Nat. Mater., 2014, 13, 1096–1101 CrossRef CAS PubMed.
- X. Q. Zhang, C. H. Lin, Y. W. Tseng, K. H. Huang and Y. H. Lee, Nano Lett., 2015, 15, 410–415 CrossRef CAS PubMed.
- D. Sarkar, X. J. Xie, W. Liu, W. Cao, J. H. Kang, Y. J. Gong, S. Kraemer, P. M. Ajayan and K. Banerjee, Nature, 2015, 526, 91–95 CrossRef CAS PubMed.
- K. Kim, S. Larentis, B. Fallahazad, K. Lee, J. M. Xue, D. C. Dillen, C. M. Corbet and E. Tutuc, ACS Nano, 2015, 9, 4527–4532 CrossRef CAS PubMed.
- K. Roy, M. Padmanabhan, S. Goswami, T. P. Sai, G. Ramalingam, S. Raghavan and A. Ghosh, Nat. Nanotechnol., 2013, 8, 826–830 CrossRef CAS PubMed.
- W. J. Yu, Y. Liu, H. L. Zhou, A. X. Yin, Z. Li, Y. Huang and X. F. Duan, Nat. Nanotechnol., 2013, 8, 952–958 CrossRef CAS PubMed.
- L. Britnell, R. M. Ribeiro, A. Eckmann, R. Jalil, B. D. Belle, A. Mishchenko, Y. J. Kim, R. V. Gorbachev, T. Georgiou, S. V. Morozov, A. N. Grigorenko, A. K. Geim, C. Casiraghi, A. H. Castro Neto and K. S. Novoselov, Science, 2013, 340, 1311–1314 CrossRef CAS PubMed.
- L. Britnell, R. V. Gorbachev, R. Jalil, B. D. Belle, F. Schedin, A. Mishchenko, T. Georgiou, M. I. Katsnelson, L. Eaves, S. V. Morozov, N. M. R. Peres, J. Leist, A. K. Geim, K. S. Novoselov and L. A. Ponomarenko, Science, 2012, 335, 947–950 CrossRef CAS PubMed.
- T. Georgiou, R. Jalil, B. D. Belle, L. Britnell, R. V. Gorbachev, S. V. Morozov, Y. J. Kim, A. Gholinia, S. J. Haigh, O. Makarovsky, L. Eaves, L. A. Ponomarenko, A. K. Geim, K. S. Novoselov and A. Mishchenko, Nat. Nanotechnol., 2013, 8, 100–103 CrossRef CAS PubMed.
- W. J. Yu, Z. Li, H. L. Zhou, Y. Chen, Y. Wang, Y. Huang and X. F. Duan, Nat. Mater., 2013, 12, 246–252 CrossRef CAS PubMed.
- X. D. Duan, C. Wang, J. C. Shaw, R. Cheng, Y. Chen, H. L. Li, X. P. Wu, Y. Tang, Q. L. Zhang, A. L. Pan, J. H. Jiang, R. Q. Yu, Y. Huang and X. F. Duan, Nat. Nanotechnol., 2014, 9, 1024–1030 CrossRef CAS PubMed.
- C. H. Lee, G. H. Lee, A. M. van der Zande, W. C. Chen, Y. L. Li, M. Y. Han, X. Cui, G. Arefe, C. Nuckolls, T. F. Heinz, J. Guo, J. Hone and P. Kim, Nat. Nanotechnol., 2014, 9, 676–681 CrossRef CAS PubMed.
- M. Y. Li, Y. M. Shi, C. C. Cheng, L. S. Lu, Y. C. Lin, H. L. Tang, M. L. Tsai, C. W. Chu, K. H. Wei, J. H. He, W. H. Chang, K. Suenaga and L. J. Li, Science, 2015, 349, 524–528 CrossRef CAS PubMed.
- Y. J. Gong, Z. Liu, A. R. Lupini, G. Shi, J. H. Lin, S. Najmaei, Z. Lin, A. L. Elias, A. Berkdemir, G. You, H. Terrones, M. Terrones, R. Vajtai, S. T. Pantelides, S. J. Pennycook, J. Lou, W. Zhou and P. M. Ajayan, Nano Lett., 2014, 14, 442–449 CrossRef CAS PubMed.
- Y. C. Lin, N. Lu, N. Perea-Lopez, J. Li, Z. Lin, X. Peng, C. H. Lee, C. Sun, L. Calderin, P. N. Browning, M. S. Bresnehan, M. J. Kim, T. S. Mayer, M. Terrones and J. A. Robinson, ACS Nano, 2014, 8, 3715–3723 CrossRef CAS PubMed.
- D. Kozawa, A. Carvalho, I. Verzhbitskiy, F. Giustiniano, Y. Miyauchi, S. Mouri, A. H. C. Neto, K. Matsuda and G. Eda, Nano Lett., 2016, 16, 4087–4093 CrossRef CAS PubMed.
- H. Luo, J. W. Krizan, E. M. Seibel, W. Xie, G. S. Sahasrabudhe, S. L. Bergman, B. F. Phelan, J. Tao, Z. Wang and J. Zhang, Chem. Mater., 2015, 27, 6810–6817 CrossRef CAS.
|
This journal is © The Royal Society of Chemistry 2017 |