DOI:
10.1039/C6NH00167J
(Communication)
Nanoscale Horiz., 2017,
2, 89-98
Tuneable fluidics within graphene nanogaps for water purification and energy storage†
Received
13th September 2016
, Accepted 4th January 2017
First published on 4th January 2017
Abstract
Precise control of liquid–solid interactions within sub-micrometer spaces is critical to maximize the active surface areas in porous materials, yet is challenging because of the limited liquid penetration. Here we discover an effective, dry-climate natural plant-inspired approach to guide water into sub-micrometer graphene microwells (Sub-μGWs) and to tune the transition from the hydrophobic to superhydrophilic states. Dry plasma texturing of Sub-μGWs by graphene ‘nano-flaps’ which adjust the tilt and density upon controlled liquid evaporation leads to controlled and stable sub-micrometer-scale surface modification and variable wettability in a wide range. This effect helps capture Au nanoparticles on the Sub-μGW surfaces as a proof-of-principle water purification platform and tune the charge-storage capacity and frequency response of Sub-μGW-based supercapacitors without altering the Sub-μGW backbones. The outcomes may be extended into diverse materials and solutions thus opening new opportunities for next-generation devices, systems and applications.
Conceptual insights
Controlling the surface wettability of nanostructures is critical for a range of applications, yet it remains challenging to achieve super-hydrophilic or super-hydrophobic states without using functional chemical coatings. The mechanism of wetting at the micro- and nano-scale, in particular at the interior surfaces of the nanostructures where air is trapped, remains poorly understood. Herein, inspired by a dry-climate plant Callitris endlicheri to utilize capillary effects to transport and store water, we demonstrate a new concept to precisely tune the wetting states of graphene nanostructures in a broad range from intrinsically hydrophobic to super-hydrophilic. Contrary to common chemical surface modification approaches, this was enabled by plasma-assisted growth of graphene “nano-flaps” covalently bonded to the backbones of micro-sized vertical graphenes inter-networked into sub-micrometer graphene wells (Sub-μGWs). The capillary effects within the confined sub-micrometer spaces lead to better water purification from metal nanoparticles and electrochemical performance of Sub-μGWs in supercapacitors. This bio-inspired conceptual approach can be used to effectively control the wettability of diverse porous, hierarchical and microstructured surfaces without changing the elemental composition, and may lead to new and enhanced nanoscale applications in energy storage and conversion, water purification, drug release, industrial catalysis, photosynthesis, and biomedical devices.
|
Introduction
The outstanding promise of nanomaterials in energy and environmental applications mainly stems from their low-dimensional structure, high specific surface area, and unique physical, chemical, and mechanical properties.1,2 Typical examples include capture of ions from electrolytes for energy storage (e.g., supercapacitors)3,4 and particulate impurities from liquids for water purification.5 These and many other applications rely on the optimum utilization of nanomaterials' surfaces in liquids for the adsorption of species.6,7 In nanomaterials with porous, hierarchical and closely packed structures, the liquid–solid interactions within sub-micrometer spaces are critical to confine and/or capture ionic, radical and particulate species.8–13 In this context, surface areas wetted by the liquid play a more important role in applications than other exposed but not wetted areas. This phenomenon limits diverse applications of many porous nanomaterials and requires attention.
The extent of wetting of the interior surfaces determines the fraction of the total surface area interfaced by the liquid as opposed to by the penetrating air. When the surface energy is higher, this fraction increases (the surface becomes more hydrophilic), and the exchange of electrons, atomic, ionic, radical and molecular species and/or clusters and nanoparticles between the interior solid surfaces and the liquid medium becomes more effective. Tuning the surface energy within sub-micrometer spaces may thus lead to the presently limited ability to harvest the controlled liquid penetration and interfacing with the interior solid surfaces (sub-microfluidics). This ability may maximize the accessibility and utilization of the available surface areas of nanomaterials. Consequently, patterns of functional nanostructures could be created within the spaces leading to localized exchange of electrons and other species. These effects may enable new functionalities and enhance device performance.
The surface energy is commonly controlled by surface coatings or texturing at the micro- and nanometer scales.14–18 However, it is challenging to implement either of these strategies within the sub-micrometer wells or channels. Apart from the obvious water repellence by hydrophobic materials, sub-micrometer wells may resist liquid penetration (e.g., by air capture) even if their walls are made of hydrophilic materials.15,19–22 This is why common wet chemistry is often ineffective because liquid solutions of the surface modifier agents cannot penetrate within the micro-spaces, apart from the obvious environmental hazards they pose.
Bio-inspired surface texturing provides conceptually novel approaches for precise, purpose-driven surface modifications. For example, bio-mimetics of surface topographies of Namib desert beetles, cacti, and Nepenthes pitcher plants are very effective for controlling dropwise condensation.23 While mimicking self-adaptation of natural plants to adjust their wetting behaviour in response to environmental factors has been utilized in many bio-mimetic materials and systems, wet-chemistry free surface texturing in sub-micrometer confined spaces is quite challenging because the textures should be very small, made of the same material as the walls, and show sufficient stability and functionality in operation. With over 460 million years of evolution and natural selection, many plants have developed hierarchical structures and microscopic surface textures to adapt to diverse and harsh environments. Studies of the internal structure of these plants help utilize the concept of microscopic surface texture-induced hydrophilicity in various applications.18,24–27 One such plant, black cypress pine Callitris endlicheri (Fig. 1a) uses capillary effects in tracheids to transport and store water. Inside the wall of tracheids, hairy micrometer-sized trichomes form superhydrophilic surfaces and play a critical role in water transport (Fig. 1b).28 This work demonstrates a similar ability, but at much smaller (sub-micrometer) scales, and utilizes it for water purification and energy storage.
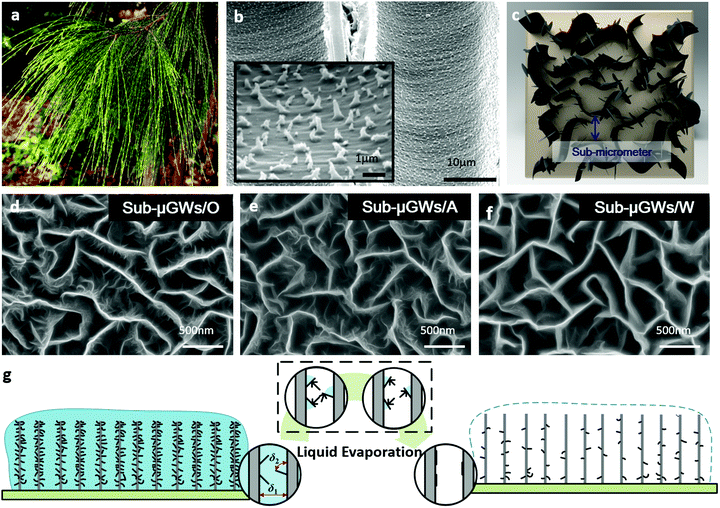 |
| Fig. 1 Bio-inspired nanotextures within sub-micrometer graphene wells (Sub-μGWs). (a) Photo image of the foliage of Callitris endlicheri. (b) SEM image of the internal surfaces of the tracheids of Callitris endlicheri. Inset: Magnified SEM image of surface roughness. Reprinted with permission from ref. 23; Copyright (2006) American Chemical Society. (c) Schematic of the sub-micrometer channels with the ‘nano-flap’ surface nanotextures. SEM images of (d) Sub-μGWs/O, (e) Sub-μGWs/A, and (f) Sub-μGWs/W showing the decreasing nanotexture densities from (d) to (f). (g) Schematic of the capillarity-driven modification process for the adjustment of the nanotexture density in Sub-μGWs. | |
Indeed, here we report on a similar, yet an-order-of-magnitude-downscaled (sub-microfluidic) effect by using sub-micrometer graphene wells (Sub-μGWs), common all-carbon nanomaterials that are actively pursued for energy and environmental applications.29,30 The wells are formed by an interconnected network of vertically-oriented graphenes (VGs) which are intrinsically mildly hydrophilic (water contact angle of ∼86° for a graphitic surface15,31). However, the resulting Sub-μGWs are hydrophobic because the easily captured air bubbles prevent liquid penetration into the wells.
The conceptual approach proposed here to effectively control the surface energy within the confined spaces resolves this issue and is based on wet-chemistry-free formation of graphene ‘nano-flaps’ covalently bonded to the Sub-μGW backbone surfaces (Fig. 1c). The nano-flaps can be regarded as nanoscale equivalents of the trichomes of Callitris endlicheri. The density and tilt of these nanostructures is then varied via slow evaporation of liquid in a simple yet controlled fashion. This leads to tunable sub-microfluidic responses by varying the surface energy of the Sub-μGWs in a broad range (from 8.9 to 73.5 mN m−1). In particular, the elusive transition from the intrinsically hydrophobic to the induced superhydrophilic state becomes possible. The demonstrated effect extends the ability of Callitris endlicheri and other plants both in terms of the range of possible wetting responses and into much smaller, micro/nano-gap spaces.
Our approach has several distinctive features and advantages compared with the existing state-of-the-art. These include simplicity in operation, intrinsic all-carbon structure, controlled wet-chemistry-free nanoscale texturing in sub-micrometer spaces, tunable wettability over a broad range, and a remarkable stability against ageing (see Table S1 in the ESI†). This approach enables effective capture of particulate contamination in liquids and helps tailor the frequency response and energy storage performance of supercapacitors. First, the ‘nano-flap’-textured Sub-μGWs siphon and capture metal nanoparticles from aqueous solution contaminated with particulates. Second, effective charge exchange with deeply penetrating electrolyte ions increases the volumetric specific capacitance of Sub-μGW-based supercapacitor electrodes by ∼2.5 times. Moreover, the controlled adjustment of the ‘nano-flaps’ is used to tune the capacitance and frequency response of the supercapacitors, without altering the morphology of the Sub-μGW backbone structures.
Methods
Fabrication of Sub-μGWs
A custom-made microwave plasma-enhanced chemical deposition (M-PECVD) system was applied for the growth of the vertically-oriented graphenes that form Sub-μGWs. A 2.45 GHz microwave source was coupled to the synthesis reactor vessel, i.e., a cylindrical quartz tube, via a traverse rectangular cavity waveguide. The grown Ni film substrate was placed on the surface of a stainless steel holder. Prior to the growth, the substrate was heated to 650 °C by microwave exposure. Subsequently, a CH4/H2 mixture was introduced into the reactor for the Sub-μGW growth. The pressure in the reactor was maintained at 500 Pa during the growth process. The Sub-μGW structures are formed by the interconnected three-dimensional networks of the vertically-oriented graphenes.
Microscopy and microanalysis
The morphological and structural characteristics of the materials were investigated using a scanning electron microscope (SEM, SU-70, HITACHI). Raman spectra were obtained using a DXR 532 Raman spectrometer (Thermo Fisher Scientific) with an excitation wavelength of 532 nm. Investigation on the elemental composition was carried out using X-ray photoelectron spectroscopy (XPS, VG Escalab Mark II) with a monochromatic Mg Kα X-ray source (1253.6 eV, West Sussex).
Wetting performance measurements
The contact angles and water penetration of the water droplets on Sub-μGWs were measured using a computerized contact angle analyzer (DropMeter A-200, MAIST), with nanotexture densities varied in a broad range. Liquid drops (10 μL) were deposited on the surface of the Sub-μGWs using a motor-driven syringe device. The contact angles were calculated by fitting the captured drop shape to the shape calculated using the Young–Laplace equation. The droplet volume and contact area diameter above the sample top surface were evaluated from the captured droplet images. Droplet impact experiments were conducted using the following procedure. De-ionized water droplets of controlled size (4 μL) were generated by forcing water from a syringe pump through a flat-tipped stainless-steel needle. Droplets fell onto the Sub-μGW surface placed 50 mm below the needle tip, with an impact velocity of 1 m s−1. Images of droplet impact were captured using a high-speed camera (REDLAKE MotionXtra HG-100K). All the tests were performed at atmospheric pressure and room temperature (25 °C).
Alternative plasma surface modification
The oxygen plasma treatment was performed in an Oxford Instruments PlasmaLab System 100 (see S6 in the ESI†) at a 100 W radio-frequency (RF) power (13.56 MHz). Before the treatment, the chamber was evacuated to a base pressure of ∼0.005 mTorr using a turbo-molecular pump backed by a dry mechanical pump. Then the oxygen plasma treatments were conducted at atmospheric pressure and at a relatively lower pressure of 20 mTorr, respectively, with pure oxygen employed as the feeding gas. All the treatments were conducted for 10 s.
Nanoparticle capture from solution
The samples were prepared by dipping Sub-μGWs/O and Sub-μGWs/W into an aqueous Au nanoparticle solution (0.1%, 15–20 nm size) for 1 h, followed by freeze drying. The nanoparticle loading mass on the Sub-μGW supports was obtained from inductively coupled plasma mass spectrometry (ICP-MS, XSENIES, Thermo Electron Corporation) tests. The deposited specimens submitted for ICP-MS analysis were dissolved in aqua regia at room temperature.
Electrochemical measurements
Supercapacitors were assembled in a two-electrode configuration with a layered structure and all the components were sandwiched between two pieces of plastic sheets. Two pieces of Sub-μGW electrodes (∼15 mm in diameter) were separated by a porous polypropylene film in a 6 M KOH aqueous electrolyte solution. Electrochemical performance was tested by cyclic voltammetry (CV) and electrochemical impedance spectroscopy (EIS) on an electrochemical workstation (PGSTAT302N, Metrohm Autolab B.V.) at room temperature. The methods of electrochemical measurements and supercapacitor electrode performance evaluations followed well-established protocols and recommendations.32
Results and discussion
Nanotextured Sub-μGWs
Fig. 1d shows a top-view SEM image of the nanotextured Sub-μGWs with a hierarchical structure. The backbone of the Sub-μGWs was formed by interconnected vertically-oriented graphene flakes grown by M-PECVD.33 Compared with the conventional chemical processes for graphene synthesis, M-PECVD produces Sub-μGWs with self-supported rigid and open structures, quasi-uniform inner spaces (see Fig. S1 in the ESI†), and ultralow amounts of impurities. Transmission electron microscopy (TEM) and high-resolution TEM (HRTEM) were also carried out to verify the graphene structure of the Sub-μGWs (presented in Fig. S2 in the ESI†). Importantly, graphene ‘nano-flap’ textures (Fig. 1c and d) with a lateral size of ∼80 nm formed on the side surfaces of the backbones, similar to the trichomes on the tracheid walls in Callitris endlicheri (Fig. 1b).
The key to obtain such a hierarchical structure, i.e., nanotextured Sub-μGWs, is the absence of direct current (dc) bias during the M-PECVD. A strong electric field perpendicular to the substrate directs graphene growth in the same direction.34–36 The dc bias was deliberately switched off to grow the graphenes simultaneously in vertical and horizontal directions, leading to the formation of nanotextured Sub-μGWs.
Tuning ‘nano-flap’ density and tilt
A capillarity-driven modification method is developed for the simple, physical (reactive chemistry-free), and tuneable control of the ‘nano-flap’ density and tilt within the Sub-μGWs. The procedure is sketched in Fig. 1g. Briefly, the original nanotextured Sub-μGWs, labelled as ‘Sub-μGWs/O’, were immersed into a liquid, followed by natural drying in air at room temperature for 24 h. During liquid evaporation, capillary forces induced between the neighbouring nanotextures and the Sub-μGW surface led to the deformation of the graphene ‘nano-flaps’ on the backbones. Moreover, by using liquids with different surface tensions, the surface roughness of the Sub-μGWs can be tailored. Fig. 1e and f present representative SEM micrographs of Sub-μGWs modified in acetone and water (surface tensions γ = 23.46 and 71.99 mN m−1 at 25 °C,37 respectively), labelled as ‘Sub-μGWs/A’ and ‘Sub-μGWs/W’, respectively. Deviations from the original Sub-μGWs/O morphology are seen, with the densities of the graphene ‘nano-flaps’ inside the Sub-μGWs decreasing in the following order: Sub-μGWs/W < Sub-μGWs/A < Sub-μGWs/O. Other structures produced using alternative liquid media (e.g., Sub-μGWs/E and Sub-μGWs/T for ethanol and toluene, respectively) can be found in the ESI† (Fig. S3).
Similar structural transformations by capillary forces have been reported previously for vertically-aligned carbon nanotubes (VACNTs).15 However, in contrast to VACNTs which collapse into bundles,38–40 the backbone of Sub-μGWs withstand liquid evaporation with only a certain amount of the ‘nano-flaps’ folded. It could be attributed to the self-supported rigid structure of the Sub-μGWs with an interconnected network. Another reason is a larger separation distance δ1 between the adjacent VG backbones than between the graphene ‘nano-flaps’ and the VG backbones δ2 (Fig. 1g). Consequently, the capillary pressure ΔP is larger around the nanoflaps as it is proportional to γ/δ, where γ is the surface tension of the liquid.41
Controlled wetting and surface energy
It is well known that the wettability of a solid surface is closely related to its surface roughness.19,42 The above nanotexture patterns were effective for tuning the surface energy and wetting properties of Sub-μGWs. Based on the Owens–Wendt surface energy theory,43 the surface energies of the Sub-μGCs/O, Sub-μGWs/A, and Sub-μGWs/W were calculated to be 73.5, 62.4, and 8.9 mN m−1, respectively (see S5 in the ESI†). Consequently, the wetting performance of Sub-μGWs can be varied from hydrophobic (Sub-μGWs/W) to hydrophilic (Sub-μGWs/A) and then to superhydrophilic (Sub-μGWs/O).
Fig. 2a–c show the optical microscope images of the apparent contact angle change for Sub-μGWs/O, Sub-μGWs/A, and Sub-μGWs/W, respectively, recorded at 0, 5, 10, 20, and 30 s. Fig. 2d presents the corresponding time dependence of the apparent contact angles for the three representative samples. The results show that water droplets spread rapidly on the Sub-μGWs/O surface within 30 s, exhibiting an equilibrium apparent contact angle of 3°. Sub-μGWs/A also presented a hydrophilic property with an equilibrium apparent contact angle of 40.6°. In contrast, the Sub-μGWs/W surface showed relatively stable and high contact angles ranging from 108° to 110.1°, indicating a clearly hydrophobic nature. With repeated experiments to minimize the statistical error, the contact angles of Sub-μGWs/O, Sub-μGWs/A, and Sub-μGWs/W were measured as 4.4 ± 1.6°, 40.7 ± 3.0°, and 109.6 ± 2.1°, respectively. The achieved effective tailoring of the surface wetting properties was also corroborated by dynamic droplet impact experiments (see S6 in the ESI†). Upon impact, a droplet was captured on the Sub-μGWs/O superhydrophilic surface, while a secondary droplet detaching from the liquid was observed on the hydrophobic Sub-μGWs/W surface.
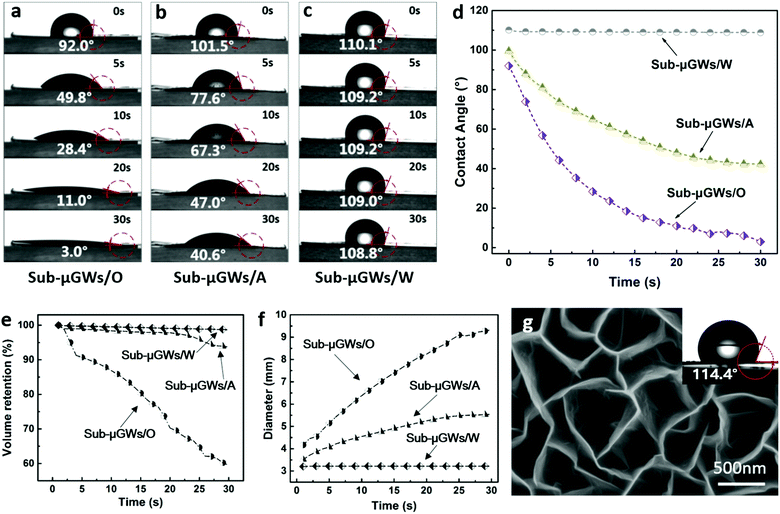 |
| Fig. 2 Controlled wetting, water penetration, and stability of sub-micrometer graphene wells. Optical microscope images of the apparent contact angle changes for (a) Sub-μGWs/O, (b) Sub-μGWs/A, and (c) Sub-μGWs/W. (d) Time dependence for water contact angles on the surfaces of Sub-μGWs within 30 s. (e) Changes in droplet volume and (f) contact area diameter on the top surface of Sub-μGWs/O, Sub-μGWs/A, and Sub-μGWs/W with continuous monitoring for 30 s. (g) SEM image of a Sub-μGWs/O sample after 90 days since fabrication; inset: optical microscopy image of the apparent contact angle. | |
Liquid penetration and spread
The wetting dynamics of Sub-μGWs can be described as a combined process of water spreading on the surface (along the horizontal direction) and water penetration into the voids (along the vertical direction).15Fig. 2e and f show the variation of the size of the water droplets on the surfaces with different levels of water repellence. The results indicate that the enhanced wettability of the Sub-μGWs favours both the penetration of water into the sub-micrometer gaps and the spread of water over the sample surface. This feature is beneficial for various applications including water purification and energy storage selected as representative examples in this work.
Nano-flaps versus oxygen plasma treatment
Our results suggest significant advantages over conventional surface treatment processes, such as oxygen plasma treatment which is also an effective method for tuning a material’s wettability. However, the oxygen plasma treatment causes substantial damage to the structures,44 changes the electrical properties through the introduction of oxygen-containing groups,45 leads to non-uniform patterns (especially for oriented structures with rough surfaces),44 and shows a significant ageing effect.46,47
Control experiments using both high-pressure and low-pressure oxygen plasmas proved that this is also true for our sub-micrometer well structures (see S7 in the ESI†). When pure oxygen is fed into the reactor, a relatively low pressure is required to achieve any significant wettability change (see Fig. S6 in the ESI†). More importantly, the introduction of oxygen groups (and hence wettability change) is only a temporary effect which fades quickly (several hours) due to natural ageing (see Fig. S6 in the ESI†). In contrast, the nano-flap structures demonstrated a high stability. Fig. 2g shows the SEM image and the optical microscope images of the apparent contact angle of a Sub-μGWs/W sample, taken after 90 days since fabrication. Our results also indicate that the simple water-evaporation treatment leads to an almost permanent hierarchical topography within the sub-micrometer gaps (i.e., density of the nano-flaps) and thus a stable wetting response.
Another obvious advantage of our method is that the surface modification does not alter the all-carbon structure of the XPS survey spectra (see Fig. S7a in the ESI†); all the Sub-μGWs exhibited a low oxygen content (0.31%, 0.33%, and 0.57% for Sub-μGWs/O, Sub-μGWs/A, and Sub-μGWs/W, respectively). The fractions of C atoms in C
C/C–C, C–O, C
O, and O
C–O were calculated (see Table S2 in the ESI†) based on the Gaussian line fitted C 1s XPS spectra (see Fig. S7b in the ESI†). No significant difference was observed among the three types of structures.
Nanotexture effects prevail
Generally, the wettability of a solid surface is controlled by the interplay of morphological (surface texture), elemental composition and chemical structure effects.14,15,19,41,42 Considering that all the Sub-μGWs have almost the same elemental composition (see Fig. S7 in the ESI†), it is reasonable to attribute the change in the surface energy and wetting performance to their varied nanotexture densities rather than the elemental composition. The surface morphologic structure of materials has a tremendous impact on their wetting behaviors, which would be more significant in nanoscale dimensions. It has been reported that a tiny change in the surface defect content could achieve a contact angle reduction of vertically-oriented graphene from 131° to 73°.48 In this work, Raman spectroscopy measurements on the Sub-μGW samples with different surface nanotexture densities were conducted (Fig. 3a) to identify their minor morphologic structure differences. The findings in ref. 48 and our work are consistent with the very recent report49 which suggests that even small nanoscale surface topography modifications can change the intrinsic wettability of the surfaces from hydrophobic to hydrophilic. This work also shows that the wettability is indeed affected by the nano-bubbles trapped by the nano-textures, and proposes a new empiric model based on the Vasilev–Ramiasa equation that reasonably accurately captures the experimental data and makes it possible to predict the wetting behaviour of the nano-textured surfaces. The ratios of D to G peak intensities (ID/IG) for Sub-μGWs/O, Sub-μGWs/A, and Sub-μGWs/W were calculated accordingly. Fig. 3b shows the relationship between the ID/IG value and the contact angle. For carbon-based materials, ID/IG usually reflects the presence of defects and an amorphous phase.50 For vertically-oriented graphenes, the ID/IG value decreases nearly linearly with the lateral dimensions of the graphene sheets.51 Given a subtle difference in the elemental composition from the XPS measurements, the relatively higher ID/IG value of Sub-μGWs/O, as compared to Sub-μGWs/W and Sub-μGWs/A, can be attributed to the presence of a large amount of nanotextures with smaller lateral dimensions. These results show that a higher density of graphene ‘nano-flaps’ on the Sub-μGWs led to a higher ID/IG value with a smaller apparent contact angle.
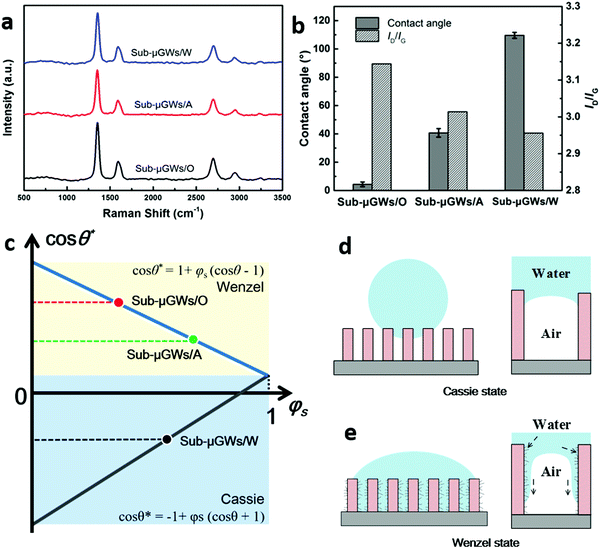 |
| Fig. 3 Relationship between the nanotextures and wetting of Sub-μGWs. (a) Raman spectra of Sub-μGWs. (b) Dependence of the apparent contact angle on the ID/IG value. (c) Plots corresponding to the Wenzel and Cassie models. (d) and (e) Schematics of the transition of Sub-μGWs' wettability between the Cassie and the Wenzel states. | |
Cassie–Wenzel wetting transition
The observed wetting properties of Sub-μGWs with the varied densities of graphene ‘nano-flaps’ can be explained by the Wenzel and Cassie mechanisms.19,42 The apparent contact angle θ* in the Wenzel and Cassie states can be calculated as:52 | cos θ* = 1 + φs(cos θ − 1) | (1) |
and | cos θ* = −1 + φs(cos θ + 1) | (2) |
respectively, where θ is the inherent or Young angle53 of the solid surface (herein θ = 86° for graphite15,31), and φs is the fraction of the solid–liquid interface in the apparent substrate surface (1 − φs is the fraction of the air–liquid interface under the droplet). Fig. 3c shows a plot of cos
θ* versus φs as described in the Wenzel and Cassie models, where two straight lines intersect at φs = 1. The apparent contact angles show that Sub-μGWs/O and Sub-μGWs/A are in the Wenzel state, whereas Sub-μGWs/W is in the Cassie state.
The transition of Sub-μGWs' wetting properties between the Cassie and Wenzel states is related to the intrinsic mildly hydrophilic state of the VG backbone structures. The thermodynamically stable state of a water droplet on the Sub-μGWs is in the Wenzel state. However, as air can be trapped within Sub-μGWs, the actual wetting state is influenced by the repulsive force from the air. Upon water penetration into the cavities, the trapped air is compressed. The resulting wetting state is thus determined by the interplay between the capillary force arising from the wetting of VG walls and the repulsion from the compressed air. Fig. 3d and e show the balancing forces at the solid–liquid–air interface at the microscopic scale. As for Sub-μGWs/W, the capillary force is not strong enough to completely displace the trapped air due to a large distance between the adjacent Sub-μGW backbones (according to the Laplace equation41). Thus, water can only contact the top edges (Fig. 3d), hence leading to a spherical shape (Cassie state19) with an apparent contact angle of 109.6 ± 2.1°.
On the other hand, the capillary force between neighbouring nano-flaps and the backbone in Sub-μGWs/O could be one magnitude higher compared to Sub-μGWs/W due to the much smaller separation. In such a nanoscale well, the electrolyte transport is strongly dominated by the capillary force originating from the wetting of the graphene surface, while the inertia force, viscous force, and liquid gravity can be neglected. The nano-flaps thus help the wetting of Sub-μGWs/O. And it becomes possible to displace the trapped air while the liquid can permeate along the Sub-μGW walls, leading to the Wenzel state42 (Fig. 3e). As such, Sub-μGWs/A and Sub-μGWs/O with higher nanotexture densities can render the hydrophilic and superhydrophilic properties, respectively.
Nanoparticle capture and ion adsorption
The superhydrophilic nature of Sub-μGWs/O, which enables unimpeded permeation of water into the sub-micrometer wells, has been demonstrated to be advantageous for the effective capture of nanoparticles and ion adsorption from aqueous solutions for water purification and energy storage applications. Fig. 4a and b show the SEM images of Au nanoparticles adsorbed on Sub-μGWs/W and Sub-μGWs/O samples, respectively. For Sub-μGWs/W without nano-flaps, nanoparticles are spontaneously attached to the exposed graphene edges (see Fig. 4a), consistent with our previous observations.54 In contrast, the dense nano-flaps introduce nanoparticles into the voids of Sub-μGWs/O, leading to the adsorption of nanoparticles on the side walls (see Fig. 4b). The much improved wetting and water penetration performance in this work also led to the increase of the mass loading of Au nanoparticles on the Sub-μGWs/O surface by ∼9.5 times, as was confirmed by the ICP-MS. This result is a proof-of-principle demonstration and contributes to the development of graphene-based hybrid platforms for water purification and other devices for environmental sensing, photo-catalytic detoxification and fuel production, and some other applications such as drug delivery.
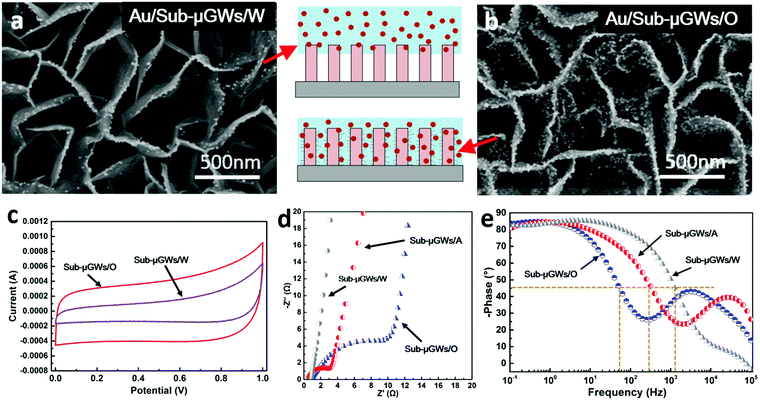 |
| Fig. 4 Controlled sub-microfluidics of sub-micrometer graphene wells (Sub-μGWs) in water purification and energy storage applications. SEM images of (a) Sub-μGWs/W and (b) Sub-μGWs/O samples decorated by Au nanoparticles captured from particulate-contaminated water. (c) CV curves of the supercapacitors employing Sub-μGWs/O and Sub-μGWs/W electrodes. (d) Nyquist and (e) Bode plots of supercapacitors employing Sub-μGWs with different wetting properties. | |
Similar observations were made when Sub-μGWs were used as the active materials for ion adsorption in energy storage devices. Fig. 4c shows the CV curves of supercapacitors employing Sub-μGWs/O and Sub-μGWs/W electrodes at a scan rate of 1.0 V s−1. Based on the CV profiles, the volumetric specific capacitance of the Sub-μGWs/O electrode was calculated as 4.08 F cm−3, which is much higher compared to the Sub-μGWs/W electrode (1.58 F cm−3). This evidences that better water permeation within sub-micrometer wells indeed enhanced the ion capture ability of the Sub-μGWs intended for charge storage applications.
Tuneable application performance
Our capillarity-driven modification method also allows a facile yet efficient manipulation of the nanotextures within the Sub-μGWs, providing a promising alternative for tuning the application performance. For instance, the Sub-μGWs/A electrode presented a volumetric specific capacitance of 2.16 F cm−3 (a CV curve is available in Fig. S8 in the ESI†), which is higher than that of Sub-μGWs/W but is lower than that of Sub-μGWs/O. Meanwhile, the ionic resistance and the frequency response of the supercapacitor electrodes can be effectively controlled by adjusting the nanotexture density in the Sub-μGWs. Fig. 4d shows a comparison between the complex plane (Nyquist) impedance for Sub-μGWs/W, Sub-μGWs/A, and Sub-μGWs/O electrodes. A clear loop with a line intersecting the real axis at a near −45° angle in the Nyquist plot of the Sub-μGWs/O electrode at relatively high frequencies indicates a reasonably high ionic resistance.
In this case, the high density of nanotextures increased the electrode porosity and improved distributed charge storage, similar to porous carbonaceous electrodes.55,56 At a lower nanotexture density, the porosity effects weakened (see the Nyquist plots of Sub-μGWs/A and Sub-μGWs/W). According to the Bode plots (phase responses of the frequencies) of the three electrodes (Fig. 4e), the Sub-μGWs/W electrode exhibited a wider frequency range for the orders of magnitude higher knee frequency than those of the Sub-μGWs/O electrode. Consequently, the frequency responses could be varied in a wide range, from 50 Hz (Sub-μGWs/O) to 316 Hz (Sub-μGWs/A) and 1258 Hz (Sub-μGWs/W), at the phase angle of 45°.
Table S3 in the ESI† presents the frequency responses of supercapacitors employing different active materials. Most of the previous practices to achieve supercapacitors with a certain frequency response commonly rely on the use of different types of active materials or a substantial modification of their morphology. For example, the impedance phase angle of supercapacitors employing VGs with an individual nanosheet size and inter-sheet spacing of a few tens of nanometers reached −45° at ∼41 Hz,57 in comparison with 15
000 Hz for the counterparts with an individual nanosheet size and inter-sheet spacing of several hundred nanometers.55 In contrast, the current method realized the tunable frequency response at a broad range (over two orders of magnitude) with a facile modification of the surface roughness inside the sub-micrometer channels without significantly changing the backbones of the VGs. This approach could facilitate the application of supercapacitors in electronic and communications devices that require tuneable responses in specific frequency ranges.
A common issue for nanomaterials (such as graphene) is that although they typically feature very large specific surface areas, the actual utilization of these areas in applications may be low, e.g., because of poor accessibility of these areas by a liquid reactant. This is why substantial effort has been devoted to increasing the exposed surface area, for example, by inserting carbon black nanoparticles, carbon spheres, or water into the inter-graphene-sheet spaces to mitigate re-stacking of graphene layers.58–60 However, only a small fraction of the exposed surface is typically in close contact with the liquid, while the rest remains interfaced with air. The surface-supported, non-agglomerated Sub-μGWs normally show hydrophobic properties.20–22 In other words, only a small fraction of the surface can be wetted by water (Cassie state). Inspired by the near-complete wetting state in the xylem of Callitris endlicheri, we have solved this problem by wet-chemistry-free formation of graphene ‘nano-flaps’ inside the Sub-μGWs, leading to a wetting behaviour change from intrinsically hydrophobic to induced superhydrophilic.
On the other hand, tuning the hierarchical morphology inside sub-micrometer confined spaces is another well-known challenge. In this regard, we have demonstrated a simple yet effective route for the controlled adjustment of the density and tilt of the graphene ‘nano-flaps’. This method relies on the capillary force induced by the evaporation of a liquid and thus prevents the introduction of chemical impurities. The Sub-μGWs remain almost carbon-pure during the entire process. The water contact angle can be reduced from 109.6 ± 2.1° to only 4.4 ± 1.6° while the surface energy increases from 8.9 to 73.5 mN m−1. Such a superhydrophilic nature favours both water spreading on the top and water penetration into the sub-micrometer voids, and improves the actual utilization of the graphene surface. Moreover, the hierarchical topography within the sub-micrometer gaps and the wetting response are reasonably stable, in contrast to the obvious ageing effect in the commonly used oxygen plasma treatment.
Conclusions
Our results offer a new concept and effective approach for nanoscale tailoring of surface morphology and wetting properties within sub-micrometer wells and channels which may lead to substantial advantages in various advanced applications. Indeed, Sub-μGWs with an induced superhydrophilic response effectively captured nanoparticles and ions from solutions and electrolytes. These effects were used to tune the Sub-μGW application performance, such as the specific capacitance and frequency response in supercapacitors. The proposed method is scalable by a simple design of reactor arrays and further possibilities to manipulate reaction and output gas flows. Finally, the outcomes of this work may be extended to other material systems and lead to the development of next-generation bio-inspired functional nanostructured, multi-purpose sub-microfluidic devices. This in turn may open new opportunities and generate substantial advantages in diverse applications spanning from energy storage and water purification to photocatalysis, biosensors and controlled drug release systems.
Acknowledgements
Financial support from the National Natural Science Foundation of China (No. 51306159), Program of Introducing Talents of Discipline to University (No. B08026), DECRA and Future Fellowships and Discovery Grant from the Australian Research Council (ARC), and CSIRO Science Leadership Program are acknowledged.
References
- B. Su, Y. Tian and L. Jiang, J. Am. Chem. Soc., 2016, 138, 1727–1748 CrossRef CAS PubMed.
- J. Duan, S. Gong, G. Yuan, X. Xie, J. Lei and Q. Cheng, ACS Appl. Mater. Interfaces, 2016, 8, 10545–10550 CAS.
- J. Kim, J. Lee, J. You, M. S. Park, M. S. A. Hossain, Y. Yamauchi and J. H. Kim, Mater. Horiz., 2016, 3, 517–535 RSC.
- J. H. Lee, J. Kim, T. Y. Kim, M. S. A. Hossain, S. W. Kim and J. H. Kim, J. Mater. Chem. A, 2016, 4, 7983–7999 CAS.
- L. Yang, J. Lou, M. Ni, C. Song, J. Wu, N. P. Dasgupta, T. Peng, S. Wen and D. Tao, ACS Appl. Mater. Interfaces, 2015, 8, 772–779 Search PubMed.
- M. Winter and R. J. Brodd, Chem. Rev., 2004, 104, 4245–4269 CrossRef CAS PubMed.
- N. Sano, T. Nakamura, H. Tamon, T. Suntornlohanakul, C. Poonjarernsilp and T. Charinpanitkul, Jpn. J. Appl. Phys., 2014, 115, 154302 CrossRef.
- Y. Zheng, H. Bai, Z. Huang, X. Tian, F.-Q. Nie, Y. Zhao, J. Zhai and L. Jiang, Nature, 2010, 463, 640–643 CrossRef CAS PubMed.
- X.-M. Li, D. Reinhoudt and M. Crego-Calama, Chem. Soc. Rev., 2007, 36, 1350–1368 RSC.
- A. Verma and A. Sharma, Adv. Mater., 2010, 22, 5306–5309 CrossRef CAS PubMed.
- Y. Zhou, S. L. Candelaria, Q. Liu, Y. Huang, E. Uchaker and G. Cao, J. Mater. Chem. A, 2014, 2, 8472–8482 CAS.
- E. I. Varghabutler, S. J. Sveinsson and Z. Policova, Colloids Surf., 1991, 58, 271–286 CrossRef CAS.
- Y. T. Hanba, A. Moriya and K. Kimura, Plant, Cell Environ., 2004, 27, 413–421 CrossRef CAS.
- D. Quéré, Annu. Rev. Mater. Res., 2008, 38, 71–99 CrossRef.
- H. Liu, J. Zhai and L. Jiang, Soft Matter, 2006, 2, 811–821 RSC.
- J. T. Woodward, H. Gwin and D. K. Schwartz, Langmuir, 2000, 16, 2957–2961 CrossRef CAS.
- D. Y. Wan, C. Y. Yang, T. Q. Lin, Y. F. Tang, M. Zhou, Y. J. Zhong, F. Q. Huang and J. H. Lin, ACS Nano, 2012, 6, 9068–9078 CrossRef CAS PubMed.
- X. Q. Dou, D. Zhang, C. L. Feng and L. Jiang, ACS Nano, 2015, 9, 10664–10672 CrossRef CAS PubMed.
- A. Cassie and S. Baxter, Trans. Faraday Soc., 1944, 40, 546–551 RSC.
- J. Dong, Z. Yao, T. Yang, L. Jiang and C. Shen, Sci. Rep., 2013, 3, 542 Search PubMed.
- J. L. Qi, X. Wang, J. H. Lin, F. Zhang, J. C. Feng and W.-D. Fei, Nanoscale, 2015, 7, 3675–3682 RSC.
- H. Watanabe, H. Kondo, M. Sekine, M. Hiramatsu and M. Hori, Jpn. J. Appl. Phys., 2012, 51, 109–117 Search PubMed.
- K.-C. Park, P. Kim, A. Grinthal, N. He, D. Fox, J. C. Weaver and J. Aizenberg, Nature, 2016, 531, 78–82 CrossRef CAS PubMed.
- D. H. Benzing, K. Henderson, B. Kessel and J. Sulak, Am. J. Bot., 1976, 63, 1009–1014 CrossRef.
- A. M. Pridgeon, Am. J. Bot., 1981, 68, 64–71 CrossRef.
- K. Koch and W. Barthlott, Philos. Trans. R. Soc., A, 2009, 367, 1487–1509 CrossRef CAS PubMed.
- U. Bauer, M. Scharmann, J. Skepper and W. Federle, Proc. R. Soc. B, 2013, 280, 20122569 CrossRef PubMed.
- M. M. Kohonen, Langmuir, 2006, 22, 3148–3153 CrossRef CAS PubMed.
- Z. Bo, S. Mao, Z. J. Han, K. Cen, J. Chen and K. Ostrikov, Chem. Soc. Rev., 2015, 44, 2108–2121 RSC.
- Y. Yoon, K. Lee, S. Kwon, S. Seo, H. Yoo, S. Kim, Y. Shin, Y. Park, D. Kim, J.-Y. Choi and H. Lee, ACS Nano, 2014, 8, 4580–4590 CrossRef CAS PubMed.
- F. M. Fowkes and W. D. Harkins, J. Am. Chem. Soc., 1940, 62, 3377–3386 CrossRef CAS.
- M. D. Stoller and R. S. Ruoff, Energy Environ. Sci., 2010, 3, 1294–1301 CAS.
- Z. Bo, W. Zhu, W. Ma, Z. Wen, X. Shuai, J. Chen, J. Yan, Z. Wang, K. Cen and X. Feng, Adv. Mater., 2013, 25, 5799–5806 CrossRef CAS PubMed.
- M. Zhu, J. Wang, B. C. Holloway, R. A. Outlaw, X. Zhao, K. Hou, V. Shutthanandan and D. M. Manos, Carbon, 2007, 45, 2229–2234 CrossRef CAS.
- Y. Zhang, J. Du, S. Tang, P. Liu, S. Deng, J. Chen and N. Xu, Nanotechnology, 2012, 23, 015202 CrossRef PubMed.
- Z. Bo, Y. Yang, J. Chen, K. Yu, J. Yan and K. Cen, Nanoscale, 2013, 5, 5180–5204 RSC.
-
D. Lide, CRC Handbook of Chemistry and Physics, CRC Press, 2009 Search PubMed.
- K. K. S. Lau, J. Bico, K. B. K. Teo, M. Chhowalla, G. A. J. Amaratunga, W. I. Milne, G. H. McKinley and K. K. Gleason, Nano Lett., 2003, 3, 1701–1705 CrossRef CAS.
- J. G. Fan, D. Dyer, G. Zhang and Y. P. Zhao, Nano Lett., 2004, 4, 2133–2138 CrossRef CAS.
- N. Chakrapani, B. Q. Wei, A. Carrillo, P. M. Ajayan and R. S. Kane, Proc. Natl. Acad. Sci. U. S. A., 2004, 101, 4009–4012 CrossRef CAS PubMed.
-
A. W. Adamson and A. P. Gast, Physical chemistry of surfaces, Wiley, 1997 Search PubMed.
- R. N. Wenzel, Ind. Eng. Chem., 1936, 28, 988–994 CrossRef CAS.
- D. K. Owens and R. Wendt, Ind. Eng. Chem., 1969, 13, 1741–1747 CAS.
- H. Shimoeda, H. Kondo, K. Ishikawa, M. Hiramatsu, M. Sekine and M. Hori, Appl. Phys. Express, 2013, 6, 095201 CrossRef.
- W. Takeuchi, K. Takeda, M. Hiramatsu, Y. Tokuda, H. Kano, S. Kimura, O. Sakata, H. Tajiri and M. Hori, Phys. Status Solidi A, 2010, 207, 139–143 CrossRef CAS.
- C. Canal, R. Molina, E. Bertran and P. Erra, J. Adhes. Sci. Technol., 2004, 18, 1077–1089 CrossRef CAS.
- D. Jucius, V. Grigaliunas, V. Kopustinskas, A. Lazauskas and A. Guobiene, Appl. Surf. Sci., 2012, 263, 722–729 CrossRef CAS.
- J. L. Qi, X. Wang, J. H. Lin, F. Zhang, J. C. Feng and W. D. Fei, Nanoscale, 2015, 7, 3675–3682 RSC.
- M. Macgregor-Ramiasa, A. Mierczynska, R. Sedev and K. Vasilev, Nanoscale, 2016, 8, 4635–4642 RSC.
- A. C. Ferrari and J. Robertson, Phys. Rev. B: Condens. Matter Mater. Phys., 2000, 61, 14095–14107 CrossRef CAS.
- L. G. Cancado, K. Takai, T. Enoki, M. Endo, Y. A. Kim, H. Mizusaki, A. Jorio, L. N. Coelho, R. Magalhaes-Paniago and M. A. Pimenta, Appl. Phys. Lett., 2006, 88, 163106 CrossRef.
- E. Bormashenko, R. Pogreb, G. Whyman and M. Erlich, Langmuir, 2007, 23, 6501–6503 CrossRef CAS PubMed.
- T. Young, Philos. Trans. R. Soc. London, 1805, 65–87 CrossRef.
- Z. Bo, D. Hu, J. Kong, J. Yan and K. Cen, J. Power Sources, 2015, 273, 530–537 CrossRef CAS.
- J. R. Miller, R. A. Outlaw and B. C. Holloway, Science, 2010, 329, 1637–1639 CrossRef CAS PubMed.
- R. De Levie, Electrochim. Acta, 1964, 9, 1231–1245 CrossRef CAS.
- Z. Bo, Z. Wen, H. Kim, G. Lu, K. Yu and J. Chen, Carbon, 2012, 50, 4379–4387 CrossRef CAS.
- X. Yang, J. Zhu, L. Qiu and D. Li, Adv. Mater., 2011, 23, 2833–2838 CrossRef CAS PubMed.
- G. Wang, X. Sun, F. Lu, H. Sun, M. Yu, W. Jiang, C. Liu and J. Lian, Small, 2012, 8, 452–459 CrossRef CAS PubMed.
- Z. Lei, N. Christov and X. Zhao, Energy Environ. Sci., 2011, 4, 1866–1873 CAS.
Footnote |
† Electronic supplementary information (ESI) available. See DOI: 10.1039/c6nh00167j |
|
This journal is © The Royal Society of Chemistry 2017 |