DOI:
10.1039/C6RA03790A
(Paper)
RSC Adv., 2016,
6, 24320-24330
High-rate and long-life of Li-ion batteries using reduced graphene oxide/Co3O4 as anode materials†
Received
10th February 2016
, Accepted 18th February 2016
First published on 22nd February 2016
Abstract
Metal oxides as Li-ion battery anodes have received a great deal of attention because they offer a higher specific capacity than state-of-the-art commercial graphite. However, a large volume change and severe particle aggregation during battery operation have greatly impeded their practical application. Herein, we report a facile one-step microwave-assisted route for growing Co3O4 nanoparticles on reduced graphene oxide that results in a high performance anode material for Li-ion batteries. The lithium battery performances of several systems with varied reduced graphene oxide contents were studied. The optimized composites exhibit a high surface area of 222 m2 g−1, and a wide pore size distribution of 1.4 to 300 nm. More importantly, the Li-ion battery shows a high capacity of ∼1300 mA h g−1 at a high rate of 1C (1C = 890 mA g−1), long life of over 600 cycles, good capacity retention, and excellent rate capability. The synthesis process is simple, energy efficient, and time-saving, providing a new path in designing high-performance electrodes for Li-ion batteries.
1 Introduction
The rapid international growth in energy consumption, coupled with the threat of global climate change from carbon dioxide emitted from traditional sources, have made the development of coupled sustainable energy generation and storage systems of paramount importance. Lithium-ion batteries (LIBs), are widely considered as the most promising energy storage media due to their high power density and high energy density, and they are considered to be environmentally friendly.1–4 To meet the requirements of both high power and high energy density applications (e.g., electrical vehicles, portable devices, and grid-scale storage), a significant amount of work has been devoted to develop alternative high-performance electrode materials for LIBs.5–7 Various metal oxides, such as SnO2,8 Fe2O3,9 Co3O4,10 TiO2,11 NiO,12 Mn3O4
13 have been studied as candidates for the LIB anode. Among them, Co3O4 has a high theoretical specific capacity of 890 mA h g−1, ∼2 times larger than that of the state-of-the-art graphite (372 mA h g−1) anode, even higher than most other metal oxides, e.g. SnO2, TiO2, NiO. Meanwhile, compared to Mn3O4, Co3O4 shows high electrical conductivity which is beneficial to improve the capacity of the LIBs. All these are expected to meet the future requirements of LIBs.14 In this respect, a tremendous effort has been made on the synthesis of Co3O4 with different morphologies, including mesoporous hollow spheres, nanowires, nanoparticles, nanocages, and nanotubes, used as anode materials in LIBs. However, the large volume change and severe particle aggregation during the lithiation process have led to electrode degradation and, consequently, previous researchers have found rapid capacity fade of these Co-based electrodes.
Graphene, an amazing new class of two-dimensional carbon materials, has been used extensively as a substrate to support active nanomaterials for energy applications due to its high electrical conductivity, large surface area, light weight, and chemical stability.15–20 Several strategies have been developed to produce graphene. The most popular approach is to reduce chemically exfoliated graphene oxide (GO) obtained by the Hummers method.21 In previous studies, researchers have already found that Co3O4 shows relatively poor performance in electrochemical applications by itself, however, when grown onto graphene sheets, the composite exhibits surprisingly high performance. Work done by Dai's group,22 showed that the synergistic effect of reduced graphene oxide (rGO)/Co3O4 hybrid leads to surprisingly high performance in both ORR and OER applications. Cheng et al.23 studied Co3O4/graphene composites as anode electrodes, achieving a high performance in LIBs. Unfortunately, although several reports have demonstrated some enhancement in rate capacity using the Co3O4/graphene composite material as the anode, they were taken at relatively low current densities and/or short cycling times, thus less useful for commercial applications.24–26 It is much more challenging to explore Co3O4/graphene hybrid material with long cycling life and high rate capacity.
Recently, microwave-assisted hydrothermal synthesis of inorganic nanomaterials has become the subject of renewed fundamental and technological interest. Microwave synthesis has been shown to yield improved control over nanocrystal formation, time savings, reduced energy consumption, and is environmentally friendly.27,28 Modern microwave reactors, specifically designed for chemical applications, now allow for accurate, homogeneous control of the reaction temperature and pressure, which in most cases are not realized using conventional heating methods, improving the product quality and yield. Meanwhile, the synthesized nanocrystals are more uniform in dimension and composition.29–31
Herein, we report a new one-step microwave-assisted method for growing Co3O4 nanoparticles (NPs) onto mildly oxidized GO sheets to form an rGO/Co3O4 composite as an advanced anode material for LIBs (see Scheme 1). The mildly oxidized GO used in this work was made by a modified Hummers method,21,22 where a reduced amount of KMnO4 and NaNO3, as well as low reaction temperature were applied to form the high quality GO sheets. A two-step reaction mechanism was proposed for the synthesis of Co3O4 NPs on GO sheets: Co(acac)3 precursor was first adsorbed on GO sheets through chemical bonding, and subsequently, followed by Co3+ was partly reduced into Co2+ after urea decomposition and in situ forming Co3O4 NPs. The cauliflower-like Co3O4 NPs were grown on graphene layers via unique microwave hydrothermal heating to form a graphene–Co3O4–graphene sandwich-like structure. The good coupling established between Co3O4 and graphene layer can not only prevent the aggregation of Co3O4 NPs but also provides an efficient medium for lithium ion and electron transport. Moreover, graphene can release mechanical stress during Li-ion insertion/extraction cycling. Our rGO/Co3O4 based electrodes offer high specific capacity of nearly 1300 mA h g−1 at a high rate of 1C (1C = 890 mA g−1), long life of over 600 cycles, good capacity retention, and excellent rate capability.
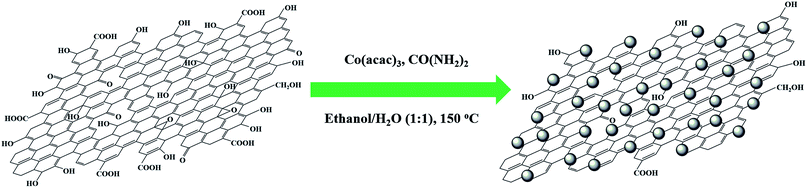 |
| Scheme 1 Schematic illustration for one-step synthesis of rGO/Co3O4 composites. | |
2 Experimental
2.1 Synthesis of graphene oxide (GO)
GO was made by a modified Hummers method, 1 g of graphite flakes (natural, −325 mesh, 99.98%, purchased from Alfa Aesar) and 20 g NaCl were ground for 20–30 minutes. Then the mixture was washed with copious amounts of water in a vacuum filtration apparatus, followed by drying in an oven at 70 °C for 1 h. Then powder was transferred to a 250 mL round bottom flask, 23 mL of concentrated sulfuric acid added, the mixture was allowed to stir at room temperature for 24 hours. Next, 100 mg of NaNO3 was added into the suspension, followed by slowly adding 1.5 g of KMnO4 (3 g for Hummers' GO). After stirring the suspension for 45 minutes, the round bottom flask was transferred into the ice bath, and 50 mL of water was added dropwise into the flask. The flask was removed from the ice bath and the suspension was allowed to stir at room temperature for another 15 minutes, followed by the addition of a mixture of 140 mL of water and 10 mL of 30% H2O2 and stirred for another 5 minutes to end the reaction. The suspension was first washed with 5% HCl solution, and followed by washing with a copious amount of water until the pH was neutral. The final precipitate was dispersed in 150 mL of water and sonicated for 30 min. Finally, a brown homogeneous suspension was collected after centrifuging at 5000 rpm for 5 minutes to remove the residues.
2.2 Synthesis of Co3O4, rGO, and rGO/Co3O4 composites
The microwave reactor used in this study was a Biotage Initiator microwave synthesizer, which was equipped with a built-in computer and touch-screen. The maximum heating temperature reached to 250 °C with a ramp rate of 2–5 °C s−1, and the pressure ranged from 0 to 20 MPa at powers of 0–400 W.
Co3O4 was made by adding 0.533 g of Co(acac)3 and 0.18 g of urea into 7.5 mL of distilled water and 7.5 mL of ethanol in a 20 mL reactor vial and sealed. The apparatus was equipped with a magnetic stirrer. A homogenous solution was obtained after sonicating for 15 min. The reaction was carried out in the Biotage Initiator microwave synthesizer, which was programmed to maintain 150 °C for 30 min. The synthesized product was collected by centrifuging and washed with distilled water and absolute ethanol repeatedly, and dried in the oven at 80 °C overnight.
rGO/Co3O4 composites with various concentrations of GO were synthesized by adjusting the starting solutions with different nominal ratios of GO. The volume of ethanol and total reaction solution remained unchanged. The ratios of the composites were calculated according the mass ratio of GO to Co3O4. Typically, 0.533 g of Co(acac)3, 0.18 g of urea and 1.58 mL of GO (the GO used in the following reaction all with a concentration of 7.6 mg mL−1, was determined by measuring the mass of the GO lyophilized from a certain volume of the suspension) into 5.92 mL of distilled water and 7.5 mL of ethanol in a 20 mL reactor vial and sealed. The apparatus was equipped with a magnetic stirrer. A homogenous solution was obtained after sonication for 15 min. The reaction was carried out in the Biotage Initiator microwave synthesizer, which was programmed to stay at 150 °C for 30 min. The synthesized product was collected by centrifugation and washed with distilled water and absolute ethanol repeatedly, and dried in the oven at 80 °C overnight.
rGO was made through the same steps used for making rGO/Co3O4 without adding any Co salt in the first step. The final product was lyophilized.
2.3 Materials characterization
Powder X-ray diffraction (XRD) patterns were recorded on a Rigaku Ultima IV instrument with Cu Kα radiation (λ = 0.154056 nm) at a beam voltage of 40 kV and a 44 mA beam current. Field emission scanning electron microscopy (FESEM) images were obtained on a JSM-6335F instrument at an acceleration voltage of 10 kV. Energy dispersive high resolution transmission electron microscopy (HR-TEM) images were collected using a JEOL 2010 FasTEM with an accelerating voltage of 200 kV. X-ray photoelectron spectroscopy (XPS) was done on a PHI model 590 spectrometer with multiprobes (Φ Physical Electronics Industries Inc.), using Al Kα radiation (λ = 1486.6 eV) as the radiation source. Raman spectra were collected using a Renishaw 2000 Ramascope Micro-Raman coupled with a 514 nm Ar ion laser and a charge-coupled device (CCD) detector. The Raman band of a silicon wafer at 520 cm−1 was used to calibrate the spectrometer. N2 adsorption measurements were performed on a Quantachrome Autosorb-1-1C automated sorption system. The samples were degassed at 150 °C for 6 h prior to the experiments. The surface areas were calculated by the Brunauer–Emmett–Teller (BET) method, and the pore size distributions were obtained by the Barrett–Joyner–Halenda (BJH) method from the desorption branch of the isotherms. The atomic force microscopy (AFM) images were performed in a Veeco Nanoscope IV multimode system. Thermogravimetric analysis (TGA) was carried out with a TA Instrument TGA Q-500 in a flowing air atmosphere (60.0 cm3 min−1). Thermal analysis was recorded ranging from room temperature to 900 °C, with a heating rate of 10 °C min−1.
2.4 Electrochemical test
All the electrodes were fabricated by preparing a slurry containing 80 wt% of active material, 10 wt% carbon black (Vulcan XC-72R, Cabot) as the conducting agent, and 10 wt% binder, made by dispersing polyvinylidene fluoride (PVDF, Kynar blend) in N-methylpyrrolidone (NMP, Acros, 99.5% Extra Dry). The slurry was homogenized through repeated and successive sonication and stirring, coated onto a copper foil (Alfa Aesar, 99.999%) and dried at 100 °C under vacuum for 12 h, then pressed at 1500 lbs for 5 min. For all electrodes fabricated in this study, the active loading was held at 0.35 mg cm−2. The material loading is for the mass of active material only. Coin cells were constructed to test the electrochemical properties of anodes in a half-cell configuration. Coin cells (2 cm in diameter, Hohsen Corp.) were assembled in an argon-filled glove box (Labconco) with the samples as test electrodes, lithium metal (Alfa Aesar, 99.9%) as the cathodes, Celgard 2320 tri-layer PP/PE/PP as the separator, and a mixture of 1 M lithium hexafluorophosphate (LiPF6, Acros 98%) in ethylene carbonate (EC, Acros 99+%)/dimethyl carbonate (DMC, Acros 98+%)/diethyl carbonate (DEC, Acros 99%) (1
:
1
:
1 by volume percent) as the electrolyte. All charge–discharge C-rate calculations of Co3O4 and rGO/Co3O4 were based on the theoretical capacity for Co3O4 of 890 mA h g−1, while the rGO electrode charge–discharge C-rate calculation was based on the theoretical capacity for graphite of 372 mA h g−1. Charge–discharge measurements were carried out at various current densities over a voltage range of 0.001 to 3 V (vs. Li+/Li) using an Arbin MSTAT battery test system. Cyclic Voltammetry (CV) was collected at a scan rate of 0.1 mV s−1 over the same voltage windows as the charge/discharge cycles.
3 Results
3.1 Characterization of graphene oxide (GO)
Electrochemical applications for graphene obtained from chemical exfoliation are limited by the quality of GO.32 One way to obtain high quality GO is to lower the degree of oxidation and to create fewer defects.33 Here, a mild synthesis procedure was used to achieve high quality GO. Fig. 1a displays the X-ray diffraction (XRD) patterns of graphite and GO. While graphite has its (002) diffraction peak at a 2θ of 27°, the peak shifts to ∼11° after oxidation, as its interlayer spacing increased from 0.34 to ∼0.87 nm. The Raman spectrum of GO in Fig. 1b exhibits characteristic D, G, 2D, and S3 bands at 1358, 1573, 2687, and 2926 cm−1, respectively, wherein the D band is related to the presence of sp3 defects, G band corresponding to the in-plane vibration of sp2 carbon atoms, and 2D band originates from a two phonon double resonance Raman process.34,35 Atomic force microscope (AFM) was used to identify the number of layers of GO, as shown in Fig. 1c, and the statistical results in Fig. 1d show that the average thickness of the GO flakes was 0.87 ± 0.25 nm, in accordance with the XRD results, indicating single layer sheets.
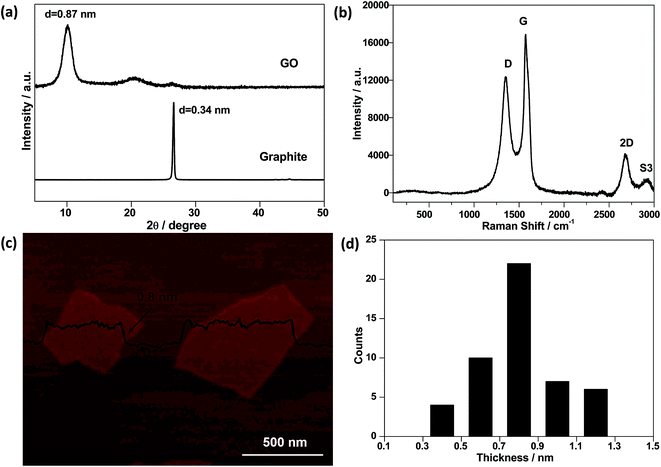 |
| Fig. 1 Characterization of chemical exfoliated GO. (a) XRD patterns for GO and graphite. (b) Typical Raman spectrum and (c) AFM image for selected GO flakes. (d) Statistical measurement results for AFM thickness for randomly selected 53 flakes (particle size > 1 μm), where the thickness ranges from 0.3 to 1.3 nm. | |
3.2 Physicochemical properties
The Biotage Initiator microwave synthesizer used in this study allows for precise control of the reaction temperature and pressure. All the Co3O4-based materials were synthesized under the same conditions; detailed reaction temperature (T), pressure (P), and power (W) profiles are shown in Fig. S1.† The rGO/Co3O4 used in this paper consisted of 10 wt% (nominal ratio) of GO in the composites, unless otherwise stated. Fig. 2a shows a scanning electron microscopy (SEM) image of rGO/Co3O4 where Co3O4 nanocrystals are uniformly grown on the surface of the graphene sheets. High-resolution transmission electron microscopy (HRTEM) images were obtained to gain further insight into the structural and morphological aspects of rGO/Co3O4. As shown in Fig. 2b, Co3O4 NPs grow on the graphene sheets with a diameter of 50–100 nm, and display a cauliflower-like structure. The detailed morphology of the cauliflower-like nanostructure in Fig. 2c reveals that the entire structure of the cauliflower is built from an abundant number of small crystals, and the nanocrystals are interconnected with each other to form a uniform pore structure within the nanoparticles. Interestingly, through SEM, nanocubes mixed with a few nanorods were observed for pure Co3O4 under the same synthetic condition, as shown in Fig. 2d. The nanocube has a particle size of less than 50 nm (Fig. 2e). HRTEM image in Fig. 2f shows similar formation process for pure single Co3O4 NP compared with Co3O4 NP grown on the graphene layer.
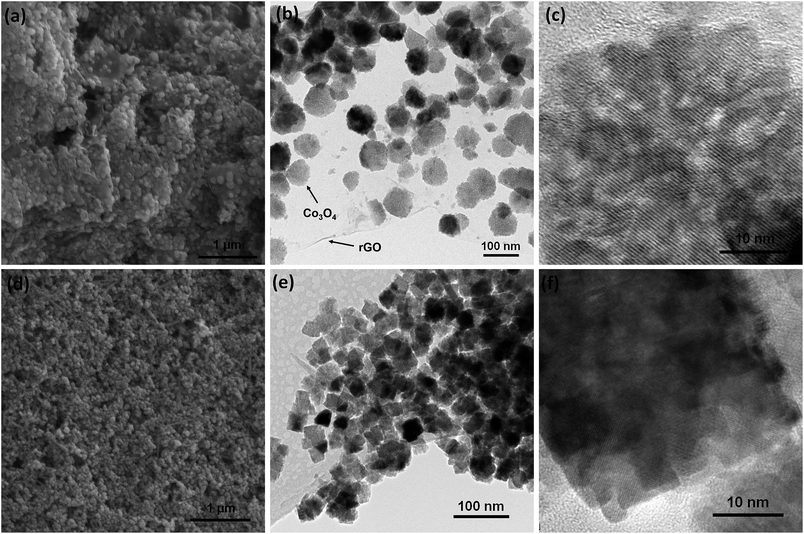 |
| Fig. 2 (a) SEM image for rGO/Co3O4. (b) and (c) HRTEM images for rGO/Co3O4. (d) SEM image for Co3O4 NPs. (e) and (f) HRTEM images for Co3O4 NPs. | |
The XRD pattern of rGO/Co3O4 in Fig. 3a exhibits the characteristic peaks of Co3O4, matching well with JCPDS no. 42-1467. No graphene diffraction peaks were observed for the rGO/Co3O4 composites, which may be due to the low graphene content (the amount of graphene was ∼6.75 wt% as revealed by thermogravimetric measurements in Fig. S2,† corresponding to the nominal ratio of 10 wt% rGO/Co3O4) as well as the relatively weak diffraction intensity of graphene materials compared with Co3O4 within the composites. The XRD patterns of rGO/Co3O4 composites with a different content of GO (Fig. S3†), show consistent characteristic diffraction peaks as pure Co3O4. The Raman spectrum of rGO, Co3O4, and rGO/Co3O4 are shown in Fig. 3b. For rGO/Co3O4, exhibits not only the characteristic bands at 191, 467, 511, 608, and 671 cm−1 corresponding to Eg, F12g, F22g and A1g modes of Co3O4, but also D and G bands of graphene at ∼1354 and 1620 cm−1. Compared with as-prepared GO, there was an obvious G band shift upward, mainly caused by the stress induced by Co3O4 NPs grown on the surface.36 The Raman D/G intensity ratios of rGO and rGO/Co3O4 composite are 0.79 and 1.11, respectively, higher than the as-made GO of 0.73, indicating a decrease in the average size of the sp2 domains, but larger in number.35 N2 adsorption measurements in Fig. 3c and d, further reveal that rGO/Co3O4 possesses a high surface area of 222 m2 g−1 and pore volume of 0.27 cm3 g−1 compared with Co3O4 values of 70 m2 g−1 and 0.29 cm3 g−1, respectively. The pore sizes range from microporous to macroporous for both Co3O4 and rGO/Co3O4 formed under the same conditions.
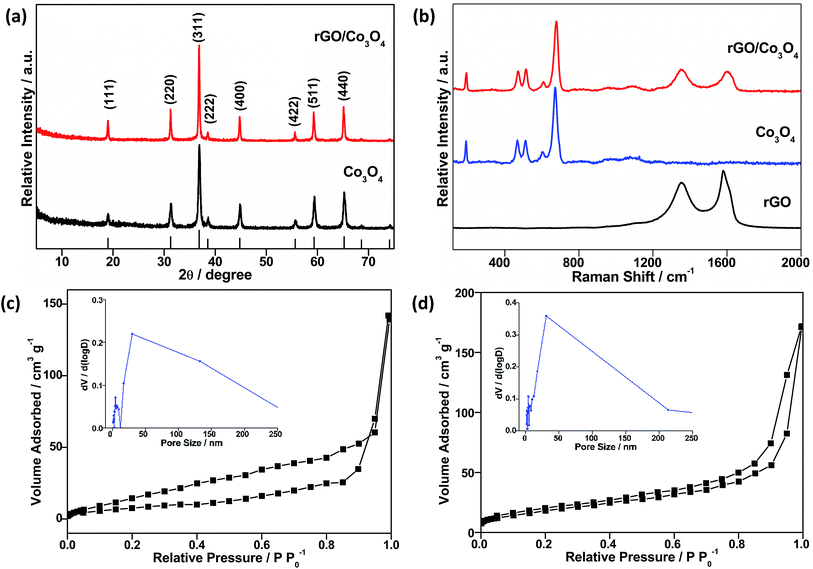 |
| Fig. 3 (a) Powder XRD patterns for Co3O4 and rGO/Co3O4. (b) Raman spectra for Co3O4, rGO/Co3O4, and rGO. (c) and (d) N2 sorption isotherms for Co3O4 and rGO/Co3O4, respectively. The inset figures are the BJH desorption pore-size distributions for each sample. | |
The chemical composition of rGO/Co3O4 was investigated by X-ray photoelectron spectroscopy (XPS), as shown in Fig. 4. Two peaks centered at 780.4 and 795.8 eV were observed, Fig. 4b, corresponding to the Co 2p3/2 and Co 2p1/2 of Co3O4, respectively. The O 1s peak at 529.9 eV in Fig. 4c further confirmed the presence of Co3O4, while the rest of the components at 531.5 eV, 533.1 eV, and 534.5 eV can be attributed to “carbon–oxygen” functional groups.23,37 Further, the C 1s spectrum in Fig. 4d reveals the different functional groups of carbon atoms: the nonoxygenated ring C, the C in C–N and C–O bonds, the carbonyl C, and the carboxylate carbon.38 These results demonstrate that Co3O4 nanocrystals grow on rGO sheets form tightly coupled composites.
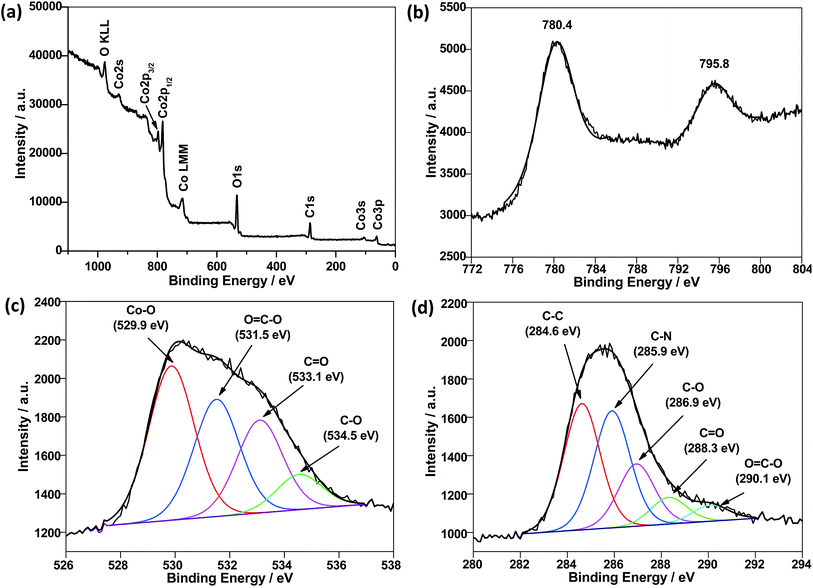 |
| Fig. 4 XPS spectra for rGO/Co3O4 composites: (a) survey spectrum and high resolution (b) Co 2p, (c) O 1s, and (d) C 1s spectra. | |
3.3 Electrochemical characterization
Cyclic voltammograms (CV) were first obtained to evaluate the electrochemical performance of the rGO/Co3O4 at a scan rate of 0.1 mV s−1 at room temperature. As shown in Fig. 5a, the initial cycle exhibits two anodic peaks at 1.4 and 2.1 V, corresponding to the oxidation of Co0 to Co2+ and Co3+ (3Co (s) + 4Li2O (s) → Co3O4 (s) + 8Li+ + 8e−). Simultaneously, the two cathodic peaks observed at 0.7 and 1.4 show the reduction of Co2+ and Co3+ to Co0 (Co3O4 (s) + 8Li+ + 8e− → 3Co (s) + 4Li2O (s)). The sharp cathodic peak indicates fast kinetics for the phase transformation of Co3O4 and the formation of the solid electrolyte interphase (SEI) on the surface of rGO/Co3O4 nanocomposite.39 In the following two cycles, both the anodic and cathodic peaks are consistent, whereas, the two cathodic peaks shift to 1 and 1.3 V, respectively. These results indicate that the electrochemical reversibility of rGO/Co3O4 is achieved after the initial cycle where the SEI formation occurs.40 For comparison, CV curves of Co3O4 are also presented, as shown in Fig. 5b, which exhibit consistent features with rGO/Co3O4.
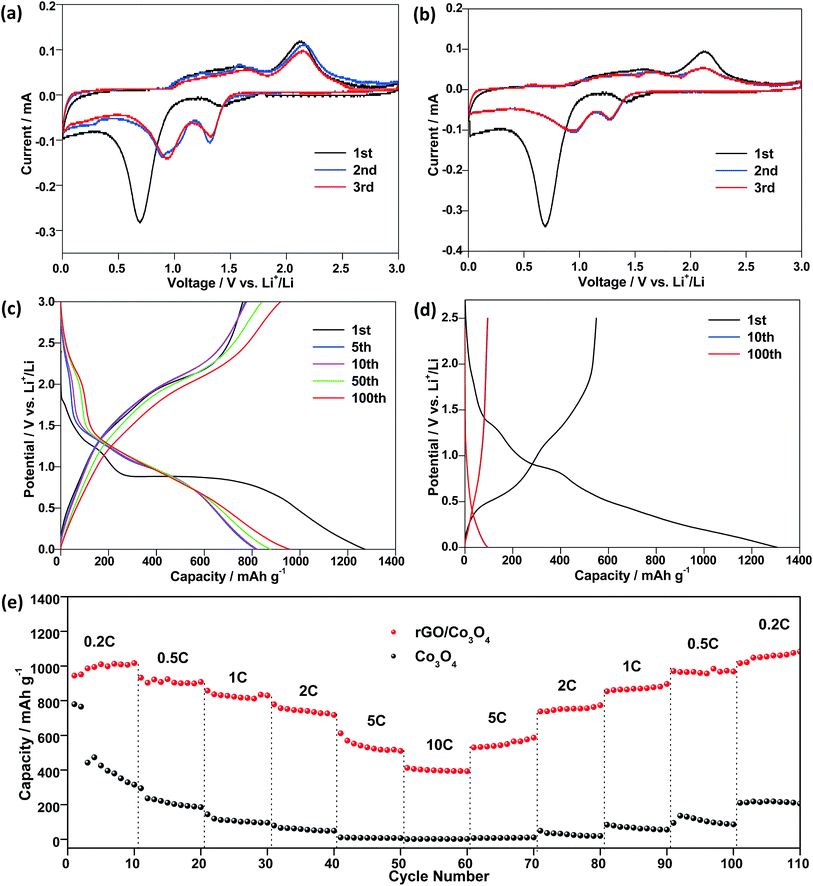 |
| Fig. 5 Electrochemical test: cyclic voltammograms (CV) curves of rGO/Co3O4 (a) and Co3O4 (b) at a scanning rate of 0.1 mV s−1, galvanostatic charge–discharge curves of rGO/Co3O4 (c) and Co3O4 (d) at a current rate of 1C, (e) rate performance of rGO/Co3O4 and Co3O4 at various current densities between 0.2C and 10C. | |
To further corroborate the high performance of the as-prepared rGO/Co3O4 composite anode, galvanostatic charge–discharge cycling was evaluated at a current rate of 1C (1C = 890 mA g−1). Fig. 5c shows a representative charge–discharge profile of rGO/Co3O4 in the voltage range of 0.001–3.0 V. A long voltage plateau at approximately 0.8 V is observed for the first discharge process, which is related to the formation of SEI on the surface of the rGO/Co3O4 composite. The lithium which participates in the formation of SEI is not reversible.41 The sloping curve down to the cutoff voltage of 0.001 V in the first discharge step, corresponds to the formation of a polymer/gel-like film.42 The first charge and discharge capacities were 761 and 1272 mA h g−1, respectively, giving a first cycle coulombic efficiency of 59.8%, mostly due to the formation of the SEI layer. From the fifth to the hundredth cycle, the charge–discharge curves are almost identical, indicating that the electrochemical process is stable during the lithium insertion–deinsertion reactions. In contrast, a short voltage plateau for Co3O4 was observed in the first discharge step, as shown in Fig. 5d. Meanwhile, after the tenth cycle, the charge and discharge capacities degrade sharply to less than 100 mA h g−1. A similar phenomenon was observed for rGO (at a current rate of 1C, 1C = 372 mA g−1, as shown in Fig. S4†). These results are very different than those of raw Co3O4, which showed poor performance and rapid capacity loss over the same number of cycles.
The rate capability is another critical parameter for the LIBs. The rGO/Co3O4 composites exhibit excellent rate capability at charge–discharge rates ranging from 0.2 to 10C, as shown in Fig. 5e. Even at a high rate of 10C, the composite still maintained a high reversible capacity of about 400 mA h g−1, which is higher than even the theoretical capacity of state-of-the-art graphite anode materials. More importantly, an average specific capacity of 1054 mA h g−1 can be restored when the current density is decreased to 0.2C. For comparison, the charge–discharge rate of Co3O4 was also presented in Fig. 5e, which shows low specific capacity.
Cycling performances of rGO, Co3O4, and rGO/Co3O4 at a 1C rate are shown in Fig. 6, where rGO/Co3O4 exhibits the best electrochemical lithium storage and cycling performance. The rGO/Co3O4 material shows a similar cycling trend with rGO, where the reversible capacity increases slowly from 821 to 1297 mA h g−1 after 600 cycles, which exceeds the theoretical capacity of Co3O4 of 890 mA h g−1. A stable coulombic efficiency of ∼96% was also observed over 600 cycles for rGO/Co3O4. To search for the best electrode, cycling performances of the composites with various rGO concentrations were investigated under the same electrochemical conditions (Fig. S5†). For 2.5 and 5 wt%, the battery reversible capacity quickly decayed after several dozen cycles. In contrast, 15 and 30 wt%, show consistent and stable cycling performances, but lower reversible capacity with an increase of rGO concentration. Further, we recorded the structure and morphology results for rGO/Co3O4 electrodes after 900 cycles (Fig. S6†). Both XRD and selective area electron diffraction (SAED) patterns show that Co3O4 nanocrystals became amorphous after long time cycling. Meanwhile, the fringe Co3O4 NPs have the same shapes as the initial morphology.
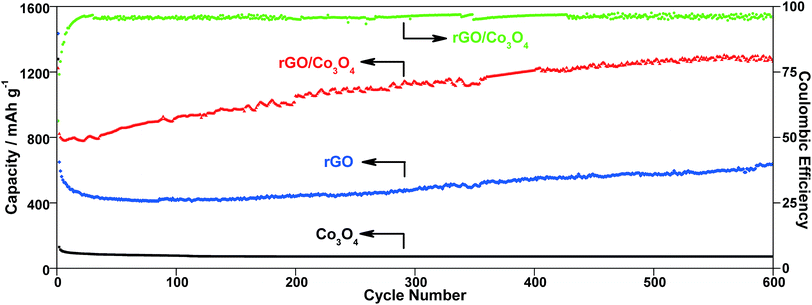 |
| Fig. 6 Cycling performance of rGO, Co3O4, rGO/Co3O4 and corresponding coulombic efficiency at a current rate of 1C (C-rate calculations of Co3O4 and rGO/Co3O4 were based on the theoretical capacity for Co3O4 of 890 mA h g−1, C-rate calculation of rGO was based on the theoretical capacity for graphite of 372 mA h g−1). | |
4 Discussion
Over the past few years, graphene has been thoroughly studied for electrochemical energy storage application due to its amazing properties. Several synthetic routes have been established to prepare graphene, of which reduction of chemically exfoliated GO obtained by the Hummers method is the most popular one.48 The methods adopted for graphene production play a crucial role in determining the properties of the final product. The drawback for chemical oxidation and exfoliation of graphite is the ready creating of defects, including structural defects and oxygen functional groups, especially structural defects that will cause irreversible damage to the material even after post treatment. In this respect, our GO was synthesized with a reduced amount of KMnO4 and NaNO3, as well as a low reaction temperature, which made the method of chemical oxidization and exfoliation of graphite sheets both less consumptive of chemicals and energy intensive compared to previous methods. More importantly, this mild synthesis method is believed to not only be favorable for retaining the properties of pristine graphene, but also to easily reduce GO during the process of forming Co3O4. Raman spectroscopy as a versatile tool, was applied to efficiently evaluate the quality of the exfoliated graphene. As shown in Fig. 1b, our GO exhibits a weak D band and sharp 2D band, which suggest a low defect density.43 The 2D band also reflects the thickness of GO that is a very sharp and symmetric for a single layer, and gets broader for multi-layers. AFM statistical results in Fig. 1d also indicate our GO was mostly single layer with an apparent thickness of ∼0.87 nm.
In order to synthesize Co3O4 NPs in one-step, and reduce GO simultaneously, cobalt(III) acetylacetonate (Co(acac)3) was used as the precursor, while urea was applied as a reducing agent for the reaction. In the initial reaction stage, Co(acac)3 was chemically adsorbed onto GO sheets through chemical bonding with oxygen functional groups (RO) of GO, as shown in eqn (1).
|
Co(acac)3 (l) + RO-graphene (s) → Co(acac)3⋯RO-graphene (s)
| (1) |
Urea played a key role for obtaining rGO/Co3O4 composite and considerable studies have been done to investigate the role of urea during the precipitation process. Li et al.44 reported that, urea, after decomposition at about 70 °C, to produce NH3 and CO2, and followed by hydrolysis of NH3 and CO2 to generate OH− and CO32− which will slowly deposit metal ion. Li et al.45 also reported similar results. The main reactions are shown as follows:
|
CO(NH2)2 (s) + H2O (l) → 2NH3 (g) + CO2 (g)
| (2) |
|
CO2 (g) + H2O (l) → CO32− (l) + 2H+ (l)
| (3) |
|
NH3 (g) + H2O (l) → NH4+ (l) + OH− (l)
| (4) |
Recently, urea also has been demonstrated to be an effective environmentally friendly reducing agent for preparing graphene.46 Co3O4 spinel phase finally formed, as equation shows below:
|
3Co3+ (l) + 2OH− (l) + 3CO32− (l) → Co3O4 (s) + 3CO2 (g) + H2O (l)
| (5) |
The experimental parameters as well as thermodynamic and kinetic control on the reaction between metal salts and urea for the formation of 3D architectures are well-known factors influencing the nucleation and crystal growth. In our experiment, homogenously grown Co3O4 NPs on mildly oxidized GO sheets as well as the reduction of GO were achieved by controlling the hydrolysis rate of Co(acac)3 via adjusting the ethanol/H2O ratio, concentration of urea, and reaction temperature. GO in this study was partly reduced by growing Co3O4 nanoparticles on the graphene sheets in terms of chemical binding with oxygen-containing groups. Meanwhile, urea as an efficient reducing agent, could also remove oxygen-containing groups from GO. Nitrogen was also introduced into the carbon lattices to fill the defects created by the oxidation process.
Generally, the morphology of the material reflects its internal structure. As shown by the SEM and TEM images of Co3O4 and rGO/Co3O4 in Fig. 2, respectively, it is easy to observe a cauliflower-like nanostructure for rGO/Co3O4, compared with the cube-like morphology obtained for pure Co3O4. The difference is mainly attributed to the presence of graphene. Graphene layers are made up of carbon atoms, linked by sp2 bonds in a hexagonal lattice network. Graphene up on oxidation, forms oxygen functional groups on the carbon atoms. When we in situ synthesize Co3O4 NPs on graphene layers, leads to Co(acac)3 first chemically adsorbed onto GO sheets. Thus, the Co3O4 NPs were formed and grown along with oxygen functional groups after decomposition of urea. The morphology of these small crystals, grown on graphene is similar to a hexagonal shape. Finally, a cauliflower-like morphology was formed when these nanocrystals interconnected with each other. This result is consistent with what we observed for single Co3O4 NP in Fig. 2c.
The content of graphene for the best electrode material, 10 wt% rGO/Co3O4 (nominal ratio), was determined by thermogravimetric measurements (Fig. S2†), and was about 6.75 wt%. Both Co3O4 and graphene contribute to the electrode capacity.47,48 Co3O4 and Li ions follow the electrochemical reaction below:
|
Co3O4 (s) + 8Li+ + 8e− → 3Co (s) + 4Li2O (s)
| (6) |
where, one mole of Co
3O
4 reacts with eight moles of Li ions and theoretically delivers high capacity of 895.5 mA h g
−1.
25 In addition, a study by Wang's group showed that the theoretical capacity of graphene was 744 mA h g
−1 when Li ions were attached to both sides of the graphene.
49 Therefore, the theoretical capacity of 10 wt% rGO/Co
3O
4 is about 885 mA h g
−1 (744 mA h g
−1 × 0.0675 + 895.5 mA h g
−1 × 0.9325). However, the theoretical lithium capacity of graphene is still not confirmed yet, Pan
et al. reported a higher capacity over 744 mA h g
−1.
47 The theoretical capacity of rGO/Co
3O
4 is expected to be over 885 mA h g
−1.
Several factors were attributed to achieve high performance of rGO/Co3O4 for LIBs applications. A comparison indicates that the reversible capacity of the rGO/Co3O4 is much better than those previously reported (Table 1). High electron conductivity is one of the most important features for graphene used in this application that benefits the fast Li+ transfer. To achieve this goal, urea was used as a reductant for GO to eliminate the oxygen functional groups. Meanwhile, nitrogen was doped into the carbon lattice to reestablish the planar sp2-hybridized structure. Nitrogen was demonstrated to improve the electrochemical performances of graphene and graphene related materials tremendously.50 From XPS results shown in Fig. 4d, after careful fitting, the C–N bond at 285.9 eV was observed for rGO/Co3O4. The peak of the C–N bond has a relatively high intensity suggesting the presence of nitrogen in the carbon lattice. This result was also confirmed by the survey spectrum with the presence of a weak N peak. Another unique property of graphene is its inherent mechanical strength. Graphene sheets serve as two-dimensional supports for the homogenous growth of Co3O4 NPs, in favor of mechanical stress released during Li-ion insertion/extraction cycling. The graphene–Co3O4–graphene sandwich-like structure and the veins within cauliflower-like Co3O4 NPs observed from SEM and TEM, respectively, suggest that channels were formed within the Co3O4 NPs as well as between graphene and Co3O4 NPs, which could not only facilitate the fast Li+ transport but also provide a buffer space for the volume change of Co3O4 NPs during Li insertion and extraction cycling. Graphene sheets were also used to efficiently prevent the aggregation of Co3O4 NPs. Furthermore, the high surface area of rGO/Co3O4 derived from N2 adsorption (Table S1†) indicates more accessible active sites in the material. Meanwhile, the wide pore size distribution of Co3O4 NPs, provides a short diffusion length for Li+. All the above data explain the high capacity at a high current density, good capacity retention, long cycling life, and excellent rate capability of the rGO/Co3O4 anode.
Table 1 Summary of the electrochemical properties of Co3O4 and carbon composites
Electrode material |
Current density (mA g−1) |
Reversible capacity (mA h g−1) |
Cycle |
Reference |
Co3O4/graphene |
50 |
935 |
30 |
23 |
Co3O4/CNT |
100 |
781 |
60 |
55 |
Co3O4/C |
100 |
1060 |
100 |
56 |
Co3O4/graphene |
200 |
740 |
60 |
24 |
Co3O4/graphene |
200 |
778 |
42 |
25 |
Co3O4/CNT |
100 |
873 |
50 |
57 |
Co3O4/carbon aerogel |
50 |
779 |
50 |
58 |
Co3O4/C |
445 |
1026.9 |
50 |
59 |
Co3O4/graphene |
400 |
775.2 |
50 |
60 |
rGO/Co3O4 |
890 |
1297 |
600 |
This work |
Co3O4 nanocrystals, after a long time of cycling, became amorphous (Fig. S6†) indicating that Co3O4 NPs undergo significant changes in chemistry and structure during cycling. The result we investigated agrees well with a prior report that used identical-location TEM showing that the NiO nanostructures completely transformed after two cycles when carbon was added. This study demonstrates that high conductivity of graphene made the metal oxide structure amorphous which is the root cause for good reversible capacity.51,52
The amount of graphene in the composite greatly affects the performance of the electrodes and was systematically studied in search of the best LIB material. For 2.5 wt%, the battery shows poor cycling performance, consistent with that of pure Co3O4, suggesting a lack of graphene sheets in the composite to support excess Co3O4 NPs and leads to the aggregation of Co3O4 NPs during the cycling process. This results in a large irreversible capacity loss and poor cycling stability. After increasing the amount of graphene sheets to 5 wt%, the electrode shows the highest capacity (∼1700 mA h g−1) in the first 50 cycles, which may be due to the larger effective surface and grain boundary area of Co3O4 nanoparticles improved by additional graphene sheets. The following fast capacity degradation may also attribute to the low amount of graphene sheets in the composite. In contrast, batteries with 10, 15, and 30 wt% of graphene, show consistent and stable cycling performances, but slight degradation of reversible capacity which increasing amounts of graphene, indicating that an excess amount of graphene may increase the diffusion length for Li+. A previous study of carbon nanofiber–Co3O4 composites attributes the extra capacity to the formation/dissolution of the film, large effective surface, and grain boundary area of Co3O4 NPs, which may offer extra sites for Li+.42,53 In our work, the increasing capacity of rGO/Co3O4 with cycling may also result from the gradual exfoliation of graphene layers to accommodate more sites and a low energy barrier for Li+ insertion and extraction.54
5 Conclusions
In summary, we have successfully grown cauliflower-like Co3O4 NPs on rGO sheets using a newly designed facile one-step microwave-assisted hydrothermal method. The composites with various concentrations of GO have been systematically studied as anode materials for LIBs. The optimized rGO/Co3O4 composite shows a high surface area 222 m2 g−1 and a wide pore size distribution ranging from microporous to macroporous, which favors Li+ transfer. Co3O4 NPs alone made by this method shows poor performance in a lithium ion battery. However, when combined with graphene, the Co3O4 composites exhibit a surprisingly high reversible capacity of ∼1300 mA h g−1 at a high rate of 1C, long cycling life of over 600 cycles, and excellent rate capability. The increased capacity for rGO/Co3O4 may be derived from the gradually expanded and exfoliated interlayers of rGO. The Co3O4 crystal structure changed to an amorphous material after long term insertion–deinsertion of Li+. The good quality of rGO may be responsible for the highly reversible capacity retention of rGO/Co3O4 over extended cycling. This study shows a facile synthesis and improved electrode performance of rGO/Co3O4, which will provide a new avenue for the development of the next generation of energy transfer and storage devices.
Acknowledgements
S. L. S. acknowledges support from the U.S. Department of Energy, Office of Basic Energy Sciences, Division of Chemical, Biological and Geological Sciences (grant DE-FG02-86ER13622A000). We thank the Institute of Materials Science, University of Connecticut, for access to the SEM, HRTEM, and XPS instruments. We thank Dr Frank Galasso for help in revising the manuscript and Dr Heng Zhang for helpful discussion in XPS.
Notes and references
- P. G. Bruce, S. A. Freunberger, L. J. Hardwick and J.-M. Tarascon, Nat. Mater., 2012, 11, 19–30 CrossRef CAS PubMed.
- B. Dunn, H. Kamath and J.-M. Tarascon, Science, 2011, 334, 928–935 CrossRef CAS PubMed.
- A. S. Aricò, P. Bruce, B. Scrosati, J.-M. Tarascon and W. van Schalkwijk, Nat. Mater., 2005, 4, 366–377 CrossRef PubMed.
- M. Armand and J.-M. Tarascon, Nature, 2008, 451, 652–657 CrossRef CAS PubMed.
- S. W. Lee, N. Yabuuchi, B. M. Gallant, S. Chen, B. Kim, P. T. Hammond and Y. Shao-Horn, Nat. Nanotechnol., 2010, 5, 531–537 CrossRef CAS PubMed.
- A. Magasinski, P. Dixon, B. Hertzberg, A. Kvit, J. Ayala and G. Yushin, Nat. Mater., 2010, 9, 353–358 CrossRef CAS PubMed.
- P. G. Bruce, B. Scrosati and J. Tarascon, Angew. Chem., Int. Ed., 2008, 47, 2930–2946 CrossRef CAS PubMed.
- J. Liang, X.-Y. Yu, H. Zhou, H. Bin Wu, S. Ding and X. W. D. Lou, Angew. Chem., Int. Ed., 2014, 126, 13017–13021 CrossRef.
- J. Chen, L. Xu, W. Li and X. Gou, Adv. Mater., 2005, 17, 582–586 CrossRef CAS.
- J. Wang, N. Yang, H. Tang, Z. Dong, Q. Jin, M. Yang, D. Kisailus, H. Zhao, Z. Tang and D. Wang, Angew. Chem., Int. Ed., 2013, 52, 6417–6420 CrossRef CAS PubMed.
- Y. Ren, Z. Liu, F. Pourpoint, A. R. Armstrong, C. P. Grey and P. G. Bruce, Angew. Chem., Int. Ed., 2012, 51, 2164–2167 CrossRef CAS PubMed.
- Y. Zou and Y. Wang, Nanoscale, 2011, 3, 2615–2620 RSC.
- H. Wang, L.-F. Cui, Y. Yang, H. Sanchez Casalongue, J. T. Robinson, Y. Liang, Y. Cui and H. Dai, J. Am. Chem. Soc., 2010, 132, 13978–13980 CrossRef CAS PubMed.
- X. Wang, X. L. Wu, Y. G. Guo, Y. Zhong, X. Cao, Y. Ma and J. Yao, Adv. Funct. Mater., 2010, 20, 1680–1686 CrossRef CAS.
- S. Stankovich, D. A. Dikin, G. H. B. Dommett, K. M. Kohlhaas, E. J. Zimney, E. A. Stach, R. D. Piner, S. T. Nguyen and R. S. Ruoff, Nature, 2006, 442, 282–286 CrossRef CAS PubMed.
- F. Bonaccorso, L. Colombo, G. Yu, M. Stoller, V. Tozzini, A. C. Ferrari, R. S. Ruoff and V. Pellegrini, Science, 2015, 347, 1246501 CrossRef PubMed.
- A. Ambrosi, C. K. Chua, A. Bonanni and M. Pumera, Chem. Rev., 2014, 114, 7150–7188 CrossRef CAS PubMed.
- A. K. Geim and K. S. Novoselov, Nat. Mater., 2007, 6, 183–191 CrossRef CAS PubMed.
- D. Li and R. B. Kaner, Science, 2008, 320, 1170–1171 CrossRef CAS PubMed.
- Y. Zhu, S. Murali, W. Cai, X. Li, J. W. Suk, J. R. Potts and R. S. Ruoff, Adv. Mater., 2010, 22, 3906–3924 CrossRef CAS PubMed.
- J. William, S. Hummers and R. E. Offeman, J. Am. Chem. Soc., 1958, 80, 1339 CrossRef.
- Y. Liang, Y. Li, H. Wang, J. Zhou, J. Wang, T. Regier and H. Dai, Nat. Mater., 2011, 10, 780–786 CrossRef CAS PubMed.
- Z. Wu, W. Ren, L. Wen, L. Gao, J. Zhao, Z. Chen, G. Zhou, F. Li and H. Cheng, ACS Nano, 2010, 4, 3187–3194 CrossRef CAS PubMed.
- B. Li, H. Cao, J. Shao, G. Li, M. Qu and G. Yin, Inorg. Chem., 2011, 50, 1628–1632 CrossRef CAS PubMed.
- H. Kim, D. H. Seo, S. W. Kim, J. Kim and K. Kang, Carbon, 2011, 49, 326–332 CrossRef CAS.
- W. L. Yao, J. L. Wang, J. Yang and G. D. Du, J. Power Sources, 2008, 176, 369–372 CrossRef CAS.
- G. A. Tompsett, W. C. Conner and K. S. Yngvesson, ChemPhysChem, 2006, 7, 296–319 CrossRef CAS PubMed.
- M. Baghbanzadeh, L. Carbone, P. D. Cozzoli and C. O. Kappe, Angew. Chem., Int. Ed., 2011, 50, 11312–11359 CrossRef CAS PubMed.
- X. Hu, J. C. Yu, J. Gong, Q. Li and G. Li, Adv. Mater., 2007, 19, 2324–2329 CrossRef CAS.
- H. Huang, S. Sithambaram, C. H. Chen, C. Kingondu Kithongo, L. Xu, A. Iyer, H. F. Garces and S. L. Suib, Chem. Mater., 2010, 22, 3664–3669 CrossRef CAS.
- L. Xu, Y. S. Ding, C. H. Chen, L. Zhao, C. Rimkus, R. Joesten and S. L. Suib, Chem. Mater., 2008, 20, 308–316 CrossRef CAS.
- S. Eigler and A. Hirsch, Angew. Chem., Int. Ed., 2014, 53, 7720–7738 CrossRef CAS PubMed.
- S. Eigler, M. Enzelberger-Heim, S. Grimm, P. Hofmann, W. Kroener, A. Geworski, C. Dotzer, M. Röckert, J. Xiao, C. Papp, O. Lytken, H. P. Steinrück, P. Müller and A. Hirsch, Adv. Mater., 2013, 25, 3583–3587 CrossRef CAS PubMed.
- M. S. Dresselhaus, A. Jorio, M. Hofmann, G. Dresselhaus and R. Saito, Nano Lett., 2010, 10, 751–758 CrossRef CAS PubMed.
- H. Wang, J. T. Robinson, X. Li and H. Dai, J. Am. Chem. Soc., 2009, 131, 9910–9911 CrossRef CAS PubMed.
- C. Chen, W. Cai, M. Long, B. Zhou, Y. Wu, D. Wu and Y. Feng, ACS Nano, 2010, 4, 6425–6432 CrossRef CAS PubMed.
- R. J. Rani, J. Oh, J. Park, J. Lim, B. Park, K. Kim, S.-J. Kim and S. C. Jun, Nanoscale, 2013, 5, 5620–5627 RSC.
- S. Stankovich, D. A. Dikin, R. D. Piner, K. A. Kohlhaas, A. Kleinhammes, Y. Jia, Y. Wu, S. T. Nguyen and R. S. Ruoff, Carbon, 2007, 45, 1558–1565 CrossRef CAS.
- L. Shen and C. Wang, Electrochim. Acta, 2014, 133, 16–22 CrossRef CAS.
- Z. Wu, W. Ren, L. Wen, L. Gao, J. Zhao, Z. Chen, G. Zhou, F. Li and H. Cheng, ACS Nano, 2010, 4, 3187–3194 CrossRef CAS PubMed.
- G. X. Wang, Y. Chen, K. Konstantinov, M. Lindsay, H. K. Liu and S. X. Dou, J. Power Sources, 2002, 109, 142–147 CrossRef CAS.
- S. Laruelle, S. Grugeon, P. Poizot, M. Dollé, L. Dupont and J.-M. Tarascon, J. Electrochem. Soc., 2002, 149, A627 CrossRef CAS.
- J. Lu, J. Yang, J. Wang, A. Lim, S. Wang and K. P. Loh, ACS Nano, 2009, 3, 2367–2375 CrossRef CAS PubMed.
- B. Li, Y. Xie, C. Wu, Z. Li and J. Zhang, Mater. Chem. Phys., 2006, 99, 479–486 CrossRef CAS.
- C. C. Li, X. M. Yin, T. H. Wang and H. C. Zeng, Chem. Mater., 2009, 21, 4984–4992 CrossRef CAS.
- Z. Lei, L. Lu and X. S. Zhao, Energy Environ. Sci., 2012, 5, 6391–6399 CAS.
- D. Pan, S. Wang, B. Zhao, M. Wu, H. Zhang, Y. Wang and Z. Jiao, Chem. Mater., 2009, 21, 3136–3142 CrossRef CAS.
- Y. Liu and X. Zhang, Electrochim. Acta, 2009, 54, 4180–4185 CrossRef CAS.
- G. Wang, B. Wang, X. Wang, J. Park, S. Dou, H. Ahn and K. Kim, J. Mater. Chem., 2009, 19, 8378 RSC.
- P. Chen, T. Y. Xiao, Y. H. Qian, S. S. Li and S. H. Yu, Adv. Mater., 2013, 25, 3192–3196 CrossRef CAS PubMed.
- N. S. Spinner, A. Palmieri, N. Beauregard, L. Zhang, J. Campanella and W. E. Mustain, J. Power Sources, 2015, 276, 46–53 CrossRef CAS.
- N. Spinner, L. Zhang and W. E. Mustain, J. Mater. Chem. A, 2014, 2, 1627 CAS.
- S. Grugeon, S. Laruelle, L. Dupont and J. M. Tarascon, Solid State Sci., 2003, 5, 895–904 CrossRef CAS.
- J. Xiao, X. Wang, X. Q. Yang, S. Xun, G. Liu, P. K. Koech, J. Liu and J. P. Lemmon, Adv. Funct. Mater., 2011, 21, 2840–2846 CrossRef CAS.
- D. Gu, W. Li, F. Wang, H. Bongard, B. Spliethoff, W. Schmidt, C. Weidenthaler, Y. Xia, D. Zhao and F. Schüth, Angew. Chem., Int. Ed., 2015, 54, 7060–7064 CrossRef CAS PubMed.
- L. Wang, Y. Zheng, X. Wang, S. Chen, F. Xu, L. Zuo, J. Wu, L. Sun, Z. Li, H. Hou and Y. Song, ACS Appl. Mater. Interfaces, 2014, 6, 7117–7125 CAS.
- S. M. Abbas, S. T. Hussain, S. Ali, N. Ahmad, N. Ali and K. S. Munawar, Electrochim. Acta, 2013, 105, 481–488 CrossRef CAS.
- F. Hao, Z. Zhang and L. Yin, ACS Appl. Mater. Interfaces, 2013, 5, 8337–8344 CAS.
- X. Liu, S. W. Or, C. Jin, Y. Lv, W. Li, C. Feng, F. Xiao and Y. Sun, Electrochim. Acta, 2013, 100, 140–146 CrossRef CAS.
- D. Li, D. Q. Shi, Z. X. Chen, H. K. Liu, D. Z. Jia and Z. P. Guo, RSC Adv., 2013, 3, 5003–5008 RSC.
Footnote |
† Electronic supplementary information (ESI) available. See DOI: 10.1039/c6ra03790a |
|
This journal is © The Royal Society of Chemistry 2016 |
Click here to see how this site uses Cookies. View our privacy policy here.