DOI:
10.1039/C5RA16605E
(Paper)
RSC Adv., 2015,
5, 93194-93208
A novel benzimidazole derivative binds to the DNA minor groove and induces apoptosis in leukemic cells†
Received
17th August 2015
, Accepted 14th October 2015
First published on 14th October 2015
Abstract
DNA minor groove binders are an important class of chemotherapeutic agents. These small molecule inhibitors interfere with various cellular processes like DNA replication and transcription. Several benzimidazole derivatives showed affinity towards the DNA minor groove. In this study we show the synthesis and biological studies of a novel benzimidazole derivative (MH1), that inhibits topoisomerase II activity and in vitro transcription. UV-visible and fluorescence spectroscopic methods in conjunction with Hoechst displacement assay demonstrate that MH1 binds to DNA at the minor groove. Cytotoxic studies showed that leukemic cells are more sensitive to MH1 compared to cancer cells of epithelial origin. Further, we find that MH1 treatment leads to cell cycle arrest at G2/M, at early time points in Molt4 cells. Finally multiple cellular assays demonstrate that MH1 treatment leads to reduction in MMP, induction of apoptosis by activating CASPASE 9 and CASPASE 3. Thus our study shows MH1, a novel DNA minor groove binder, induces cytotoxicity efficiently in leukemic cells by activating the intrinsic pathway of apoptosis.
Introduction
Targeting DNA, the genetic material of a cell, is considered as one of the most attractive chemotherapeutic approaches and DNA interacting compounds are known to inhibit growth of cancer cells.1 Since the growth rate of cancer cells is high, it serves as an important strategy to target DNA molecules in order to interrupt replication, which is essential in each cell division of the cancer cell.2 Grooves in DNA are associated with several biological interactions inside the cell. The major groove which is wide and approximately 24 Å in width helps in the majority of protein DNA interactions, whereas the narrow minor groove which is only 10 Å in width, is an attractive target for small molecular inhibitors.3
Reversible interactions of small molecules with DNA have been majorly classified into three subgroups; intercalation, groove binding and electrostatic interactions.4 Studies on the minor groove of DNA interaction with noncovalent small molecules has resulted in emerging of many small molecule inhibitors as anticancer agents.5 Many natural products have also shown significant interactions with DNA minor groove.6 DNA interacting agents majorly affect replication and transcription activities inside the cell.7 Importantly, DNA minor groove binders are found to inhibit the topoisomerase activity and subsequent cell cycle arrest.8
Benzimidazole moiety is structurally related to purine bases and is found in a variety of natural products, such as vitamin B12. Benzimidazole nucleus is termed “master key” as it is an important core in many bioactive compounds. Different benzimidazole derivatives act at various targets within the cells to elicit an array of pharmacological properties.9 It is one of the most extensively studied class of heterocyclic compounds with wide range of biological activities such as anti-HIV,10 antioxidant,11 antifungal,12 antiviral,13 antibacterial,14 antimycobacterial15 and they are well known to possess antitumor/anticancer activity.16 Importantly, benzimidazole derivatives were found to interact with DNA17 and were also used in the medicinal field where the primary target was found to be DNA and DNA associated processes.18
In the present study, we have designed and synthesized novel 2,5 di and 2,5,7 tri substituted benzimidazole derivatives as shown in scheme 1 and scheme 2 (Fig. 1 and 2), and evaluated their anticancer activity against various cancer cell lines. MH1 was identified as the most potent small molecule inhibitor. MH1 exhibited DNA binding properties as determined by various biophysical assays, and inhibited both topoisomerase and transcription reactions. Further, ex vivo studies showed that novel benzimidazole derivative, MH1 treatment led to decrease in mitochondrial membrane potential and induced intrinsic pathway of apoptosis resulting in cytotoxicity in leukemic cells.
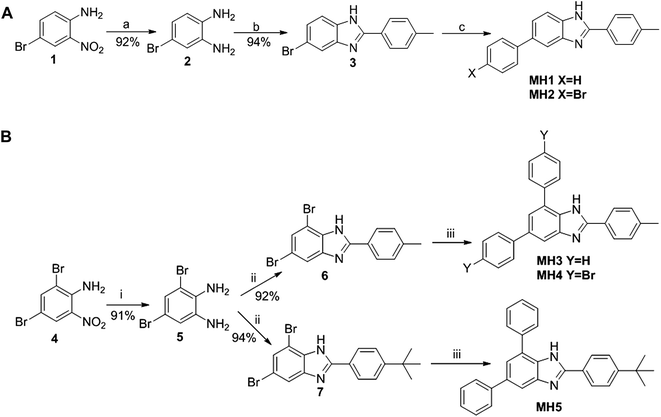 |
| Fig. 1 Synthetic schemes of compounds MH1–5. (A) synthesis of 2,5 disubstituted benzimidazole derivatives (MH1–2). (a) Zn/HCl, EtOH, reflux; (b) T3P®, p-tolualdehyde, ethylacetate, 0 °C-RT; (c) phenyl/4-bromo phenyl boronic acid, K2CO3, bis(triphenylphosphine)palladium(II) dichloride, H2O : EtOH : 1,4 dioxane (1 : 1 : 5), 130 °C. (B) synthesis of 2,5,7 trisubstituted benzimidazole derivatives (MH3–5): (i) Zn/HCl, EtOH, reflux; (ii) T3P®, p-tolualdehyde/4-tert-butylbenzaldehyde, ethylacetate, 0 °C-RT; (iii) phenyl/4-bromo phenyl boronic acid, K2CO3, bis(triphenylphosphine)palladium(II) dichloride, H2O : EtOH : 1,4 dioxane (1 : 1 : 5), 130 °C. | |
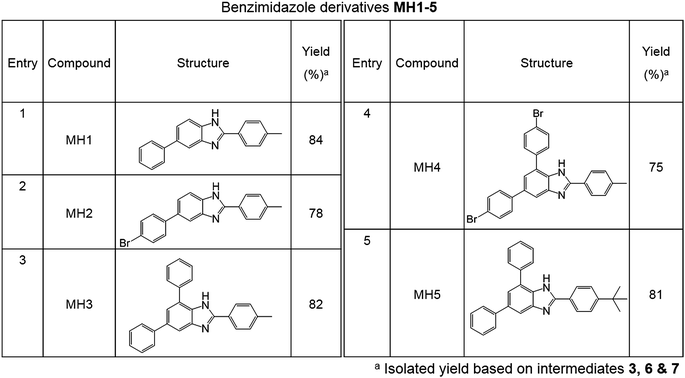 |
| Fig. 2 Chemical structure and yield of newly synthesized compounds. Chemical structure of different benzimidazole derivatives and their respective yield is indicated. | |
Results
Synthesis of novel benzimidazole derivatives
The general approach for the synthesis of 2,5-disubstituted (MH1 and 2) and 2,5,7-trisubstituted benzimidazoles (MH3–5) is outlined in scheme 1 and scheme 2, respectively (Fig. 1 and 2). As depicted in the schemes, different aryl substitution on C-5 and C-7 of benzimidazole backbone has been achieved by using an appropriate phenyl boronic acid. Thus, for the first time we describe direct tri aryl substitution on C-2, C-5 and C-7 positions of benzimidazole ring.
We have synthesized compounds MH1 and MH2 as shown in scheme 1. Nitro group reduction of 4-bromo-2-nitroaniline (1) with commercial zinc dust along with catalytic amount of HCl in ethanol under reflux condition obtained 4-bromo-1,2-diaminobenzene (2).
In continuation to our previous efforts19 we constructed benzimidazole ring using T3P® as a cyclodehydrating agent. T3P® mediated cyclocondensation of 4-bromo-1,2-diaminobenzene (2) with p-tolualdehyde in ethylacetate produced compound 3 with excellent yield. The substitution of aryl group at C-5 and C-7 of benzimidazole ring was effected with a Suzuki coupling protocol in both scheme 1 and scheme 2. Para alkyl substituted aryl boronic acids were used to make C–C bond using bis(triphenylphosphine)palladium(II) dichloride as a catalyst and potassium carbonate as a base under Suzuki reaction condition to obtain the title compounds, MH1–5.
MH1 effectively inhibits the proliferation of leukemic cells
Cytotoxic effect of newly synthesised benzimidazole derivatives were tested on different cancer cell lines using MTT assay. Results showed that among the five benzimidazole derivatives studied, MH1 showed maximum cytotoxicity in all the tested cell lines (Fig. 3A). MH1 exhibited an IC50 of 5.5, 8.9, 13.83 and 13.3 μM in leukemic cell lines, Molt4, Nalm6, REH and K562, respectively. However, MH1 was less effective, when tested in MCF7, HeLa and EAC cells, compared to leukemic cells (Fig. 3A). Hence our results suggest that leukemic cells are more sensitive to MH1 compared to cancers of epithelial origin. Sensitivity of MH1 was further evaluated at varying concentrations (0–20 μM), for 48 and 72 h in Molt4, Nalm6, REH and K562 cells. Highest concentration of DMSO treated cells served as vehicle control for the study. Results showed that among the leukemic cells tested, Molt4 exhibited maximum sensitivity to MH1, both in a dose and time dependent manner (Fig. 3B). Thus, Molt4 was selected for further studies.
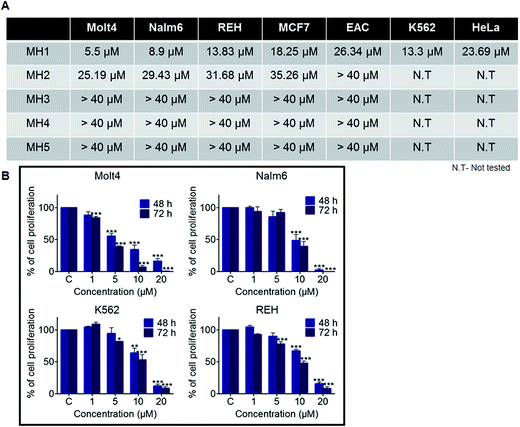 |
| Fig. 3 Cytotoxic effect of novel benzimidazole derivatives on different cancer cell lines. Molt4, Nalm6, REH, MCF7, EAC, K562, and HeLa cells were treated with different concentrations (0–40 μM) of the compounds (MH1, MH2, MH3, MH4 or MH5) for 48 or 72 h. MTT assay was performed for evaluating the effect of compounds on cell proliferation. (A) IC50 values for above compounds at 48 h as determined by MTT assay in different cancer cell lines. (B) Bar diagram (mean ± SEM) showing effect of MH1 (0, 1, 5, 10 and 20 μM) on proliferation of different leukemic cell lines (Molt4, Nalm6, K562 and REH, respectively) at 48 and 72 h of treatment. Experiment was repeated a minimum of three times with good consensus. | |
MH1 induces G2/M arrest and results in accumulation of SubG1 cells
Based on the above results, we wondered whether MH1 treatment could induce cell cycle arrest in Molt4 and K562 cells. In order to test this, Molt4 and K562 cells were treated with increasing concentrations of MH1 (1, 5, 10 and 20 μM for 48 h). PI staining followed by flow cytometry analysis, revealed a concentration dependent accumulation of SubG1 phase cells in both Molt4 and K562 cells (Fig. 4A–D). Interestingly, we also observed a G2/M arrest at a lesser extent in both the cell lines tested, particularly at 5–10 μM (Fig. 4B and D).
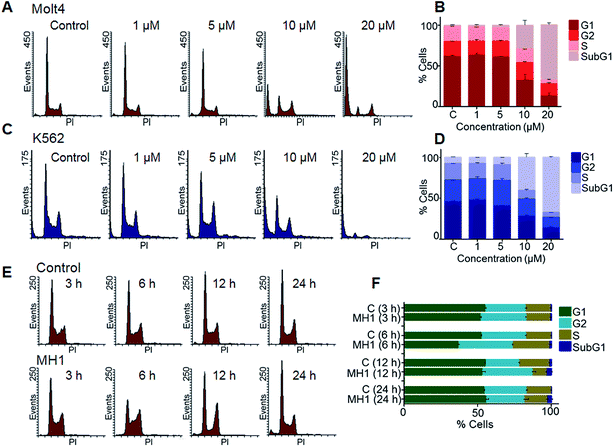 |
| Fig. 4 Cell cycle analysis of Molt4 and K562 cells following treatment with MH1. Molt4 and K562 cells were treated with different concentrations of MH1 (0, 1, 5, 10 and 20 μM for 48 h), fixed and analysed by flow cytometry after staining with propidium iodide. (A and C) Histogram showing effect of MH1 on Molt4 and K562 cells at 48 h. (B and D) Bar diagram (n = 2) showing percentage of cells in different cell cycle phases at 48 h of treatment with different concentrations of MH1 on Molt4 and K562. (E and F) Histogram showing effect of MH1 (10 μM) on cell cycle progression in Molt4 cells at different time points (3, 6, 12 and 24 h) and respective bar diagram showing cell cycle arrest (n = 3). | |
Interestingly the treatment of MH1 (10 μM) in Molt4 cells showed a prominent cell cycle arrest at G2/M phase at both 6 and 12 h time points (Fig. 4E and F). Thus our data suggests that MH1 could induce G2/M arrest at initial time points and increase SubG1 phase cell population at later time points, thereby inhibiting the proliferation of leukemic cells.
Apoptosis is induced in MH1 treated cells
Mode of cell death following MH1 treatment was analysed in Molt4 cells using annexin-FITC/PI staining. Movement of phosphatidylserine from inner membrane surface to outer membrane surface following cellular damage is an indicator of apoptosis, and can be studied following double-staining with annexin-FITC and propidium iodide (PI). Treatment of increasing concentrations of MH1 (48 h) resulted in significant increase in early and late apoptotic cells (Fig. 5A). Necrotic cells were either absent or minimal after treatment with MH1 (Fig. 5B). Besides, confocal microscopic analysis confirmed the staining of annexin-FITC alone or both annexin-FITC and propidium iodide staining in MH1 treated Molt4 cells (Fig. 5C). Thus, MH1 treatment showed activation of apoptosis in Molt4 cells.
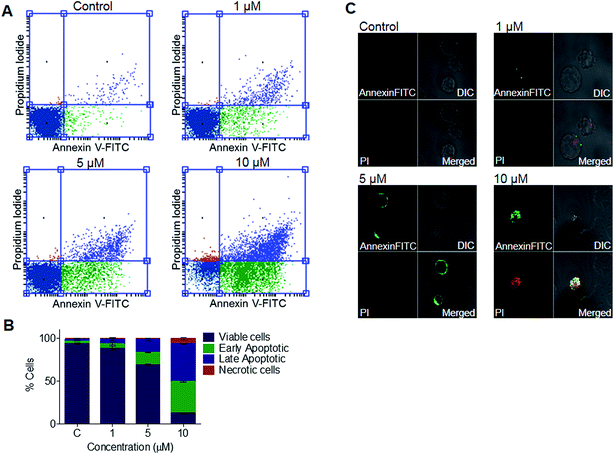 |
| Fig. 5 Evaluation of MH1 induced apoptosis by annexin-FITC/PI staining. Molt4 cells treated with MH1 (0, 1, 5 and 10 μM for 48 h) were double-stained with annexin-FITC/PI. (A) Dot plots showing apoptotic cells following treatment with MH1 on Molt4 cells. (B) Bar diagram (n = 2) representing distribution of early, late apoptotic and necrotic cell populations after treatment with MH1. (C) Confocal images of annexin-FITC/PI stained Molt4 cells, treated with MH1 (0, 1, 5 and 10 μM for 48 h). | |
Assessing the effect of MH1 on Molt4 cells using calcein-AM and ethidium homodimer staining
Calcein-AM and ethidium homodimer staining was used to analyse both live and dead cells, respectively in Molt4 cells following treatment with increasing concentrations of MH1 (0, 1, 5 and 10 μM for 48 h) (Fig. 6A). Results showed increase in cells stained with both calcein-AM and ethidium homodimer upon treatment with MH1 in concentration dependent manner (Fig. 6A and B). These results further confirm the cell death following treatment with MH1.
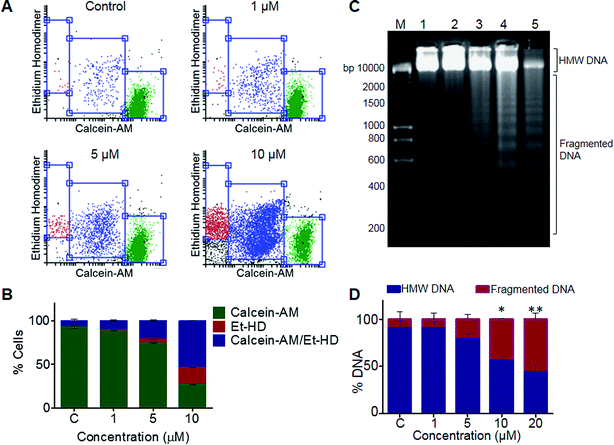 |
| Fig. 6 DNA fragmentation and live-dead cell assays following MH1 treatment in Molt4 cells. (A and B) MH1 treated (0, 1, 5 and 10 μM for 48 h) Molt4 cells were stained with calcein-AM and ethidium homodimer and analysed by flow cytometry. Dot plot represents MH1 treated Molt4 cells (A). Bar diagram (n = 2) showing percentage of calcein-AM alone (live cells) or ethidium homodimer (dead cells) alone or calcein-AM/ethidium homodimer stained Molt4 cells after treatment with MH1. (C and D) Agarose gel stained with ethidium bromide showing DNA ladder formation in Molt4 cells after treatment with MH1 (0, 1, 5, 10 and 20 μM for 48 h). ‘M’ indicates DNA ladder and bar diagram representing quantification of HMW (high molecular weight) DNA and fragmented DNA. | |
DNA fragmentation assay
DNA ladder formation is the hallmark of cellular apoptosis.20 MH1 treated Molt4 cells showed prominent DNA fragmentation at 48 h time point in a concentration dependent manner (Fig. 6C). Compared to the DMSO treated vehicle control cells, MH1 treated cells showed ladder formation from 5 μM onwards in a concentration dependent manner (Fig. 6C and D).
MH1 treatment lead to reduction in mitochondrial membrane potential
To check the effect of MH1 on mitochondrial membrane potential (MMP), JC-1 staining was performed. Interestingly, MH1 treated (0, 1, 5, 10 and 20 μM) Molt4 cells showed significant reduction in MMP in a concentration dependent manner. There were three different population of cells with altered MMP observed, having high, intermediate and low MMP. Following MH1 treatment, we observed a prominent shift of high MMP cells to intermediate or low MMP in a concentration dependent manner (Fig. 7A and B). Thus, our results suggest that apoptosis induced by MH1 on Molt4 cells could be due to mitochondrial apoptotic pathway.
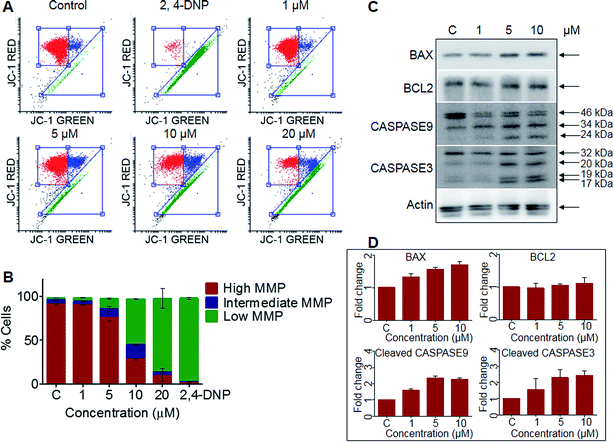 |
| Fig. 7 Evaluation of mitochondrial membrane potential and induction of apoptotic proteins in Molt4 cells following treatment with MH1. Molt4 cells were treated with different concentrations of MH1 for 48 h and measured for mitochondrial membrane potential by FACS and expression of apoptotic proteins by Western blot analysis. (A) Dot plot showing change in MMP following treatment with MH1 (0, 1, 5, 10 and 20 μM). 2,4-DNP treated Molt4 cells (48 h) served as positive control. (B) Bar diagram (n = 2) showing distribution of high, intermediate and low mitochondrial membrane potential in Molt4 cells after treatment with MH1. (C and D) Immunoblotting showing differential expression/activation of apoptotic markers BAX, BCL2, CASPASE9, CASPASE3 following treatment with MH1 in Molt4 cells (0, 1, 5 and 10 μM at 48 h), actin served as the loading control and respective quantifications are given in terms of fold change. | |
MH1 induces apoptosis through intrinsic pathway
Based on promising results observed from JC-1 staining assay we carried out Western blotting analysis for expression of apoptotic markers. Molt4 cells treated with different concentration of MH1 were subjected to immunoblotting analysis. Interestingly, we observed an up regulation of BAX expression, a protein involved in mitochondrial mediated apoptosis, following MH1 treatment (Fig. 7C). BCL2, an anti-apoptotic protein showed no significant difference in the expression in control and treated cases. However, distinct bands due to cleavage of both CASPASE9 and CASPASE3 were observed (Fig. 7C and D) following treatment with MH1. Hence, immunoblotting results suggest that MH1 treatment resulted in activation of intrinsic pathway of apoptosis in Molt4 cells.21
MH1 binds to minor groove of DNA
To test whether MH1 binds directly to DNA, UV-visible and fluorescence emission spectroscopic studies were carried out in presence of calf thymus (CT) DNA.22 UV-visible spectroscopy showed two characteristic absorption peaks for MH1 at 259 and 315 nm. Upon serial increase in the concentrations of CT-DNA, a characteristic decrease in the absorption at both 259 and 315 nm was observed, which can be directly correlated to the ability of MH1 to intercalate DNA or bind to groove of DNA (Fig. 8A).
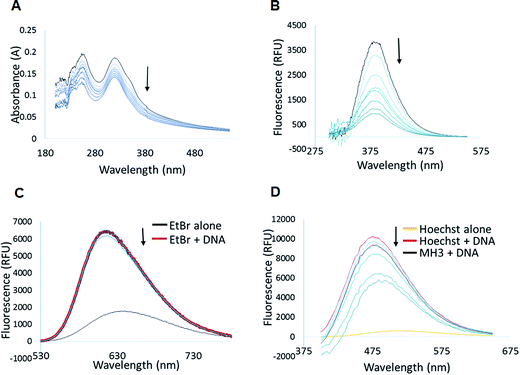 |
| Fig. 8 Evaluation of MH1–DNA interaction by UV-visible, fluorescent spectroscopy and DNA dye displacement assays. (A) MH1 was incubated with various concentrations of CT-DNA (0, 1, 5, 10, 25, 50, 75 and 100 μM) and absorbance was measured in UV-visible spectroscopy. The resulting spectra is shown as a function of wavelength. Arrow indicates increasing concentration of CT-DNA. (B) Fluorescence emission spectra of MH1 in presence of increasing concentrations of CT-DNA (0, 1, 5, 10, 25, 50, 75 and 100 μM). Arrow indicates increasing concentrations of CT-DNA. Fluorescence is expressed in RFU and is shown in Y-axis. (C) Ethidium bromide dye displacement assay showing effect of increasing concentrations of MH1 (0, 1, 5, 10, 25, 50 and 100 μM) on fluorescence emission spectra of ethidium bromide, when incubated with CT-DNA. Black spectral line indicates ethidium bromide alone, red spectral line is ethidium bromide along with DNA and blue spectral lines are increasing concentrations of MH1 (indicated by an arrow) along with ethidium bromide (1 μM) and CT-DNA. (D) Hoechst (33258) dye displacement assay showing effect of increasing concentrations of MH1 (0, 1, 5, 10, 25, 50 and 100 μM) on fluorescence emission spectra of Hoechst along with CT-DNA. Yellow spectral line indicates Hoechst alone, red spectral line indicates Hoechst and CT-DNA, black spectral line is Hoechst with CT-DNA in presence of MH3 (50 μM) and blue spectral lines indicate increasing concentrations of MH1 and marked by an arrow. | |
To confirm the binding interaction of MH1 with DNA, we took the advantage of fluorescent properties of MH1.23 MH1 exhibited a fluorescent excitation (259 nm) and emission maxima (315 nm and 385 nm). Interestingly, we observed that the fluorescence emission spectra of MH1 was decreased when incubated with increasing concentrations (0, 1, 5, 10, 25, 50, 75 and 100 μM) of CT-DNA, which confirmed above observation of binding of MH1 to DNA (Fig. 8B).
In order to test the mode of MH1 and DNA interaction, we performed DNA dye displacement assay, a test used for differentiating the intercalators and minor groove binders.24 We find that addition of MH1 (0, 1, 5, 10, 25, 50 and 100 μM) to Hoechst 33258–DNA complex decreased the characteristic emission spectra of Hoechst in a concentration dependent manner (Fig. 8D). However, MH3, a less potent compound with extra benzene ring at the seventh position on benzimidazole ring, did not induce significant change in emission spectrum (Fig. 8D), which suggested plausible MH1 interactions with minor groove of the DNA. It is possible that unlike MH1, the extra benzene ring at the seventh position on benzimidazole ring may cause a hindrance for binding to DNA. Besides, ethidium bromide dye displacement assay was also performed to check the intercalating properties of MH1. Interestingly, addition of MH1 did not change the emission spectra of ethidium bromide along with DNA confirming the observation that MH1 is not a DNA intercalator (Fig. 8C). However, there was a marginal topological alteration, when CT-DNA was added to MH1 and analysed using circular dichroism studies (Fig. 9).
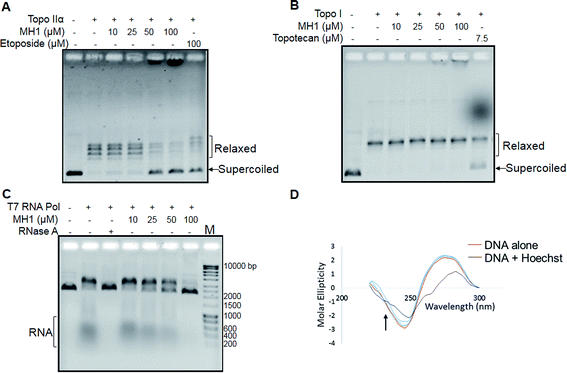 |
| Fig. 9 Evaluation of inhibitory effect of MH1 on topoisomerase action and transcription. MH1 inhibitory activity on human topoisomerase IIα and T7 RNA polymerase catalysed transcription was tested. (A) Human topoisomerase IIα activity was assayed in presence of increasing concentrations of MH1 (0, 10, 25, 50 and 100 μM) using supercoiled plasmid DNA (pBS-SK+, 37 °C for 30 min). Etoposide (100 μM) served as positive control for the reaction. Supercoiled and relaxed forms of the DNA are indicated. (B) Topoisomerase I assay in presence of MH1. Recombinant human topoisomerase I was tested for its activity on supercoiled plasmid DNA in presence of different concentrations of MH1 (0, 10, 25, 50 and 100 μM) for 30 min at 37 °C. Topotecan (7.5 μM) was used as a positive control. Positions of supercoiled and relaxed DNA are indicated. (C) In vitro transcription assay using T7 RNA polymerase was performed in presence of different concentrations of MH1 (0, 10, 25, 50 and 100 μM) for 30 min at 37 °C. To confirm RNA species (denoted in red bracket) formation, products were also treated with RNase A (50 μg ml−1). ‘M’ is molecular weight ladder. (D) Circular dichroism studies to check the topological changes in DNA in presence of MH1. CD analysis was carried out using CT-DNA (50 μM) in presence of increasing concentrations of MH1 (0, 10, 25, 50 and 100 μM). Hoechst 33258 (10 μM) was used as a positive control (black spectral line). DNA alone was presented in red spectral line. | |
MH1 interferes with some of the DNA protein transactions
Previous studies have shown that small molecules that bind DNA minor groove can affect topoisomerase action.25 We tested whether MH1 could interfere with human topoisomerase I and II. To test this supercoiled plasmid DNA (250 ng) was incubated with topoisomerases in presence of increasing concentrations of MH1 (10, 25, 50 and 100 μM) and the products were resolved on an agarose gel. Results showed that MH1 inhibited topoisomerase IIα mediated conversion of supercoiled DNA to circular form in a concentration dependent manner (Fig. 9A). Etoposide (100 μM) was used as the positive control. However, such an effect by MH1 was absent or minimal when topoisomerase I was used for the study (Fig. 9B).
We tested whether DNA binding ability of MH1 could result in inhibition of other cellular processes like transcription. Inhibitory effect of MH1 on in vitro transcription on a plasmid DNA by T7 polymerase was tested and the reaction products were visualized on an agarose gel. Addition of MH1 (10, 25, 50 and 100 μM) inhibited formation of RNA species during transcription in a concentration dependent manner (Fig. 9C). Hence our results indicate that DNA groove binding by MH1 could interfere with cellular processes drastically,26 thereby causing cell cycle arrest and apoptosis in cancer cells.
In order to have further insight on MH1 interaction with the minor groove of DNA, docking studies were carried out. Results suggested that MH1 can efficiently occupy the minor groove of DNA (Hoechst 33258 binding site) with a binding energy of −9.84 kcal mol−1 (Fig. 10A and B). Hydrogen bond Interactions of hydroxyl group of thymine and imidazole moiety of MH1 favoured the affinity of MH1 towards the DNA (Fig. 10C). Thus spectroscopic studies in conjunction with docking results suggested that MH1 binds to minor groove of DNA.
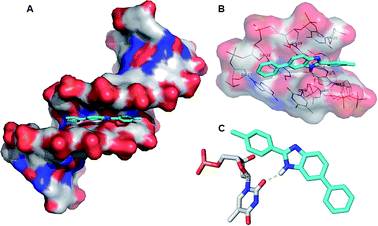 |
| Fig. 10 Molecular docking studies of MH1 with double stranded DNA. Ability of MH1 to bind at the minor groove of DNA was tested with the help of Autodock 4.2 docking software and plausible interactions are represented. (A) Docked orientation of MH1 in Hoechst binding pocket of double stranded DNA (MH1 is shown in cyan blue). (B) Interaction of MH1 at minor groove pocket of AT rich double-stranded DNA. (C) Hydrogen bond formation between imidazole moiety of MH1 and oxygen group of thymine residue inside the minor groove. | |
Discussion
In the current study, we have developed a facile, direct and efficient method for the synthesis of highly substituted benzimidazole derivatives. The present method is efficient for the chemo selective synthesis of various 2,5,7-tri-aryl substituted benzimidazole derivatives.
Cytotoxicity results of newly synthesized compounds on different cancer cell lines showed prominent or moderate cytotoxicity, among all synthesized compounds. Among the molecules studied, MH1 was most promising molecule with notable IC50 values in all cancer cell lines tested (Fig. 3A). Interestingly, effect of MH1 was more pronounced in leukemic cell lines, especially, Molt4 cells showed highest sensitivity among all tested cell lines (Fig. 3B).
The overall structure of MH1 and MH2 is in a crescent shape which is characteristic of typical minor groove binding ligands. In addition, benzimidazole core moiety has the ability to form a hydrogen bond with nucleotides. Besides, these molecules possess sufficient conformational flexibility to further help in attaining the shape, which is significant enough to fit inside the DNA minor groove.17 As observed in the cytotoxicity assays (Fig. 3B), cancer cell lines showed higher sensitivity towards MH1 and MH2, compared to rest of the compounds, which possess an extra benzene ring. It appears that the extra benzene ring could diminish the solubility of the compound as well as binding to the DNA minor groove. This explains the reduced cytotoxicity exhibited by MH3–5 as compared to MH1–2.
MH1 treatment on Molt4 cells at 6 and 12 h resulted in G2/M arrest while at 48 h time point resulted in accumulation of SubG1 phase cells in a concentration dependent manner (Fig. 4). Further, annexin-FITC/PI staining of MH1 treated Molt4 cells showed induction of apoptosis both by flow cytometry and confocal microscopy (Fig. 5). Live dead assay using calcein-AM and ethidium homodimer staining corroborated the above findings (Fig. 6). DNA ladder formation in MH1 treated Molt4 cells further confirmed induction of apoptosis (Fig. 6C and D).
Interestingly, MH1 treatment resulted in lowering of mitochondrial membrane potential in Molt4 cells (Fig. 7A and B). Western blotting assays for apoptotic markers confirmed the involvement of mitochondrial machinery in activating intrinsic pathway of apoptosis (Fig. 7C and D).
Importantly, MH1 interacts with DNA (Fig. 8A and B), by binding to the minor groove of double-stranded DNA, as confirmed by DNA dye displacement assays and docking studies (Fig. 8C and D and 10). Finally, MH1 inhibited human topoisomerase IIα activity in vitro in a concentration dependent manner (Fig. 9A). Being an important topoisomerase inside cells,27 it's inhibition resulted in G2/M arrest which was prominent at 6 and 12 h of MH1 treatment in Molt4 cells. However, MH1 did not interfere with topoisomerase I activity (Fig. 9B). Besides topoisomerases, interaction of MH1 with DNA resulted in inhibition of in vitro transcription explaining the mechanism of MH1 action.28 Thus our study identifies a novel benzimidazole derivative, MH1 capable of binding to minor groove of DNA, thereby inhibiting crucial cellular processes and inducing intrinsic apoptotic pathway in leukemic cells leading to subsequent cell death.
Several benzimidazole derivatives were known to target DNA and associated processes.1b Consistent with this, the primary focus of the present study showed that novel benzimidazole derivative, MH1 interacts with the minor groove of the DNA and inhibits several cellular processes. These observations open up several new avenues for further in depth studies.
Previously, certain benzimidazole derivatives were shown to interact specifically to G-quadruplex DNA structures.29 Besides, G-quadruplex structures were shown to be one of the major reasons for DNA breakage during chromosomal translocations30 and hence cancer progression. The important property of benzimidazole derivatives to bind G-quadruplex structure showed its significant role in inhibition of telomerase enzyme activity,31 which is overexpressed in majority of cancers.32
Several known topoisomerase inhibitors like etoposide or doxorubicin are well studied for their ability to induce DNA damage and provoking DNA repair pathways and check point pathways,27 however some benzimidazole derivatives induced cell cycle arrest as well as topoisomerase inhibition without causing DNA damage.33 Therefore, further studies are required to address how these benzimidazole derivatives regulate signalling pathways which are important for cell cycle arrest and induction of apoptosis.
Materials and methods
Chemistry
The progress of all reactions was monitored by TLC, which was performed on 2.0–5.0 cm aluminum sheets precoated with silica gel 60 F 254 to a thickness of 0.25 mm (Merck) using UV light for visualization. 1H NMR and 13C NMR spectra were recorded on an Agilent-Varian NMR spectrometer operating at 400 and 100 MHz, respectively (ESI Fig. 1a to n†). Chemical shifts are given in δ values (ppm) using tetramethylsilane as the internal standard. Mass spectra were recorded using high resolution mass spectrometer (HRMS). Infrared spectra were recorded in KBr on Shimadzu FT-IR model 8300 spectrophotometer. Chromatographic separations were carried out on 60
:
120 silica gel.
4-Bromo-1,2-diaminobenzene (2). 4-Bromo-2-nitro-aniline (5 mmol) and zinc dust (10 mmol) with catalytic amount of HCl was refluxed in ethanol (10 mL) for 3 h. The reaction mixture was filtered through Celite and filtrate was evaporated under reduced pressure, the resulting residue was dissolved in ethylacetate and washed with water followed by brine solution. The organic layer was dried over anhydrous Na2SO4 and evaporated under vacuo to obtain a desired amino product 2 as white solid.34
5-Bromo-2-p-tolyl-1H-benzimidazole (3). T3P® (1.5 mmol, 50% solution in ethylacetate) was added to the solution of 4-bromo-1,2-diaminobenzene (1.0 mmol) and p-tolualdehyde (1.0 mmol) in ethylacetate at 0 °C and the resulting reaction mixture was stirred at room temperature for 2 h. The reaction mixture was diluted with water (20 mL) and neutralized with 10% NaHCO3 solution and the product was extracted with ethyl acetate (10 mL × 2), the combined organic layer was washed with water followed by brine solution. The organic phase was dried over anhydrous Na2SO4 and evaporated under reduced pressure to obtain a crude product, which was purified by column chromatography using ethyl acetate and hexane as an eluent to get the desired product 3 as a pale yellow solid. 1H NMR (400 MHz, CDCl3): δ 8.32–8.30 (d, J = 8.0 Hz, 2H, ArH), 7.79 (br s, 1H, ArH), 7.38–7.36 (d, J = 7.6 Hz, 1H, ArH), 7.33–7.30 (dd, J = 8.4, 1.4 Hz, 1H, ArH), 7.29–7.27 (d, J = 7.8 Hz, 2H, ArH), 2.21 (s, 3H, Ar-CH3).
3,5-Dibromobenzene-1,2-diamine (5). 2,4-Dibromo-6-nitroaniline (5 mmol) and zinc dust (10 mmol) with catalytic amount of HCl was refluxed in ethanol (10 mL) for 4 h. The reaction mixture was filtered through Celite and filtrate was evaporated under reduced pressure, the resulting residue was dissolved in ethylacetate and washed with water followed by brine solution. The organic layer was dried over anhydrous Na2SO4 and evaporated under vacuo to obtain 3,5-dibromobenzene-1,2-diamine (5) as a pale brown solid.
5,7-Dibromo-2-p-tolyl-1H-benzimidazole (6) & 5,7-dibromo-2-(4-tert-butylphenyl)-1H-benzimidazole (7). Compounds 6 and 7 were synthesized as described earlier for the synthesis of 3 using p-tolualdehyde and 4-tert-butylbenzaldehyde as an aldehyde component to construct benzimidazole ring, respectively. 5,7-Dibromo-2-p-tolyl-1H-benzimidazole (6); pale brown solid; 1H NMR (400 MHz, CDCl3): δ 8.30–8.28 (d, J = 8.0 Hz, 2H, ArH), 7.81 (s, 1H, ArH), 7.64 (s, 1H, ArH), 7.31–7.29 (d, J = 8.2 Hz, 2H, ArH), 2.23 (s, 3H, Ar-CH3). 5,7-Dibromo-2-(4-tert-butylphenyl)-1H-benzimidazole (7); dark brown solid; 1H NMR (400 MHz, CDCl3): δ 8.29–8.27 (d, J = 8.2 Hz, 2H, ArH), 7.80 (s, 1H, ArH), 7.62 (s, 1H, ArH), 7.33–7.31 (d, J = 8.0 Hz, 2H, ArH), 1.51 (s, 9H, t-butyl-CH3).
General procedure for the synthesis of title compounds MH1–5
Corresponding bromo compounds (3, 6 & 7) (1.0 mmol) were taken in water
:
ethanol
:
1,4 dioxane (6 mL) mixture in 1
:
1
:
5 ratio, potassium carbonate (3.0 mmol) and phenyl/4-bromo phenyl boronic acid (1.1 mmol) were added to the reaction mixture and degassed with nitrogen gas for 15 min with stirring at room temperature. Bis(triphenylphosphine)palladium(II) dichloride (0.1 mmol) catalyst was added to the mixture and heated for 30 min at 130 °C in seal tube. The reaction mixture was filtered through Celite and concentrated under reduced pressure, the resulting residue was diluted with ethylacetate and washed with water followed by brine solution. The organic layer was dried over anhydrous Na2SO4 and concentrated under vacuo to give the crude product which was purified by column chromatography using hexane
:
ethylacetate as an eluent to get the title compounds (MH1–5).
5-Phenyl-2-p-tolyl-1H-benzimidazole (MH1). Yield: 84%; off white solid; m.p. 182–184 °C, IR γmax (KBr) 3350, 3154, 3123, 3087, 3030, 2980, 1380 cm−1; 1H NMR (400 MHz, CDCl3); δ 8.00–7.98 (d, J = 8.0 Hz, 2H, ArH), 7.78 (s, 1H, ArH), 7.68–7.66 (d, J = 8.0 Hz, 1H, ArH), 7.62–7.59 (m, 2H, ArH), 7.51–7.49 (dd, J = 1.6 Hz, 1H, ArH), 7.45–7.41 (m, 2H, ArH), 7.35–7.30 (m, 1H, ArH), 7.29–7.26 (m, 2H, ArH), 2.40 (s, 3H, Ar-CH3). 13C NMR (100 MHz, CDCl3); δ 152.5, 141.7, 140.6, 136.5, 129.8, 128.7, 127.3, 126.9, 126.8, 126.5, 122.6, 120.8, 117.9, 21.4. HRMS (ESI) m/z calcd for C20H16N2Na [M + Na]+ 307.3544, found 307.3563.
5-(4-Bromophenyl)-2-p-tolyl-1H-benzimidazole (MH2). Yield: 78%; brown solid; m.p. 184–186 °C IR γmax (KBr) 3362, 3164, 3127, 3048, 2980, 1397, 550 cm−1; 1H NMR (400 MHz, CDCl3); δ 7.97–7.92 (m, 2H, ArH), 7.75–7.68 (m, 1H, ArH), 7.67–7.64 (m, 2H, ArH), 7.58–7.56 (d, J = 9.6 Hz, 1H, ArH), 7.53–7.51 (dd, J = 1.6 Hz, 1H, ArH), 7.48–7.43 (m, 1H, ArH), 7.38–7.32 (m, 2H, ArH), 7.29–7.26 (m, 1H, ArH), 2.42 (s, 3H, Ar-CH3). 13C NMR (100 MHz, CDCl3); δ 152.1, 141.9, 140.8, 136.4, 130.2, 129.9, 128.3, 127.9, 126.7, 126.1, 123.1, 122.8, 121.5, 117.7, 21.3. HRMS (ESI) m/z calcd for C20H15BrN2Na [M + Na]+ 386.2505, found 386.2531.
5,7-Diphenyl-2-p-tolyl-1H-benzimidazole (MH3). Yield: 82%; pale brown solid; m.p. 166–168 °C, IR γmax (KBr) 3352, 3159, 3124, 3041, 2983, 1382 cm−1; 1H NMR (400 MHz, CDCl3); δ 8.24–8.22 (d, J = 6.8 Hz, 2H, ArH), 7.91–7.89 (d, J = 8.0 Hz, 1H, ArH), 7.85 (s, 1H, ArH), 7.75 (s, 1H, ArH), 7.66–7.64 (d, J = 7.6 Hz, 2H, ArH), 7.59–7.56 (m, 1H, ArH), 7.53–7.50 (t, J = 6.4 Hz, 3H, ArH), 7.48–7.34 (m, 3H, ArH), 7.26–7.24 (t, J = 4.0 Hz, 2H, ArH), 2.36 (s, 3H, Ar-CH3). 13C NMR (100 MHz, CDCl3); δ 152.3, 135.8, 135.6, 134.7, 133.5, 132.6, 130.0, 129.8, 129.1, 128.8, 128.7, 128.5, 128.4, 128.2, 127.9, 127.4, 127.0, 126.7, 122.8, 22.7. HRMS (ESI) m/z calcd for C26H20N2Na [M + Na]+ 383.4504, found 383.4523.
5,7-Bis(4-bromophenyl)-2-p-tolyl-1H-benzimidazole (MH4). Yield: 75%; dark brown solid; m.p. 91–93 °C, IR γmax (KBr) 3401, 3213, 3178, 3102, 3032, 2997, 1396, 550, 542 cm−1; 1H NMR (400 MHz, CDCl3); δ 7.99–7.90 (m, 2H, ArH), 7.71–7.69 (d, J = 7.2 Hz, 3H, ArH), 7.56–7.50 (m, 4H, ArH), 7.49–7.45 (m, 2H, ArH), 7.37–7.33 (m, 3H, ArH), 2.16 (s, 3H, Ar-CH3). 13C NMR (100 MHz, CDCl3); δ 152.6, 141.7, 140.5, 136.5, 132.0, 131.98, 131.93, 129.86, 129.81, 128.97, 128.90, 128.65, 128.60, 128.0, 127.5, 127.4, 126.7, 126.6, 21.4. HRMS (ESI) m/z calcd for C26H18Br2N2Na [M + Na]+ 541.2425, found 541.2442.
2-(4-tert-Butylphenyl)-5,7-diphenyl-1H-benzimidazole (MH5). Yield: 81%; off white solid; m.p. 198–200 °C, IR γmax (KBr) 3399, 3202, 3165, 3114, 2997, 2984, 1395, 1387 cm−1; 1H NMR (400 MHz, CDCl3); δ 7.97–7.87 (m, 4H, ArH), 7.80–7.70 (m, 3H, ArH), 7.68–7.63 (m, 2H, ArH), 7.62–7.51 (m, 4H, ArH), 7.31–7.28 (m, 3H, ArH), 1.25 (s, 9H, t-butyl-CH3). 13C NMR (100 MHz, CDCl3); δ 152.5, 135.5, 134.7, 133.5, 132.4, 130.0, 129.4, 129.2, 128.8, 128.7, 128.5, 128.1, 127.9, 127.8, 127.6, 127.0, 126.7, 124.6, 31.1, 30.9. HRMS (ESI) m/z calcd for C29H26N2Na [M + Na]+ 425.5301, found 425.5312.
Biology
Chemicals and reagents. All the chemicals used in the present study were of analytical grade and purchased from Sigma-Aldrich, USA. Human topoisomerase I and IIα were purchased from TopoGEN.Inc, USA. DNA modifying enzymes were from New England Biolabs (USA). Antibodies were from Santacruz Biotechnology or Invitrogen (USA).
Cell culture. Human cancer cell lines, Molt4 (acute lymphoblastic leukemia), K562 (chronic myelogenous leukemia), EAC (mouse breast cancer), MCF7 (human breast cancer) and HeLa (human cervical cancer) were purchased from National Centre for Cell Science, Pune, India and Nalm6 (B-cell leukemia) and REH (B-cell leukemia) were a kind gift from Dr M. R. Lieber, USA. Cells were cultured in RPMI1640, MEM or DMEM (Sera Lab, UK) containing 10% FBS (Gibco BRL, USA), 100 U of penicillin G ml−1 and 100 μg of streptomycin per ml (Sigma-Aldrich, USA) at 37 °C in a humidified atmosphere containing 5% CO2.
MTT assay. Cytotoxic effect of newly synthesized benzimidazole derivatives was studied using MTT assay.35 Briefly, leukemic cell lines were seeded in a 24 well plate at a density of 50
000 cells per ml and treated with different concentrations (0–40 μM) of synthesized compounds for 48 or 72 h. 100 μl of cell suspension from each sample was mixed with 10 μl of MTT (5 mg ml−1) in 96 well plate, incubated for 2–3 h, formazone crystals were solubilized in 67 μl of solution containing 10% SDS and 50% DMF (30–60 min at 37 °C) and readings were acquired at 570 nm using Biorad iMARK (USA) plate reader. Adherent cell lines (MCF7, HeLa and EAC), were seeded directly in 96 well plate at the density of 5000 cells per well and incubated with different concentrations (0–40 μM) of compounds and subjected to MTT assay as described above. Experiments were repeated a minimum of two times with good agreement, each with duplicate reactions and bar diagram is presented with error bars. IC50 values were determined using GraphPad software prism 5.1.
Cell cycle analysis. To study the effect of MH1 on cell cycle progression in Molt4 and K562 cells, cells were seeded at a density of 50
000 cells per ml, and treated with different concentrations of MH1 (0, 1, 5, 10 and 20 μM) for 48 h as described previously.36 Besides, Molt4 cells were treated with MH1 (10 μM) for different time points (3, 6, 12 and 24 h). DMSO treated cells were served as a vehicle control. For cell cycle analysis, following MH1 treatment, cells were harvested, washed in 1x PBS and fixed with 80% chilled ethanol. Fixed cells were subjected to RNase A treatment (50 μg ml−1, at 37 °C for 4–12 h). Cells were stained with propidium iodide (10 μg ml−1) and analysed in BD FACSVerse™. Minimum 10
000 cells were acquired and analysed in Flowing software (version 2.5). Experiments were repeated a minimum of two independent times and presented as histogram and % of cells in different phases of cell cycle are presented as bar diagram with error bars.
Annexin-FITC/PI staining. Induction of apoptosis following MH1 treatment in Molt4 cells was studied using annexin-FITC/PI staining kit (Santacruz, USA).37 Briefly, different concentrations of MH1 (0, 1, 5 and 10 μM) were incubated with Molt4 cells (50
000 cells per ml) for 48 h. Cells were harvested, suspended in binding buffer containing calcium along with annexin-FITC (0.2 mg ml−1) and propidium iodide (50 ng ml−1) for 20 min at room temperature and was analysed on BD FACSVerse™. Minimum 10
000 cells were acquired per sample and analysed in Flowing software (version 2.5). DMSO treated cells were used as a vehicle control. Experiments were repeated a minimum of two times and results were presented as dot plots. % Cells in early, late apoptosis and necrosis are presented as a bar diagram with error bars.For confocal microscopy, annexin-FITC/PI stained cells were fixed using 2% paraformaldehyde for 15 min at room temperature. Cells were washed with 1x PBS and mounted on slides using DABCO. Images were captured using inverted confocal laser scanning microscope (Ziess LSM 510 MK4, Germany).
Live dead cell assay. Effect of MH1 on Molt4 cell viability was also analysed by performing live dead cell assay using calcein-AM and ethidium homodimer staining.38 Briefly, Molt4 cells were incubated with increasing concentrations of MH1 (0, 1, 5 and 10 μM) for 48 h. Cells were harvested, washed in 1x PBS and incubated with calcein-AM (100 nM) and ethidium homodimer (8 μM) for 20 min at 37 °C and analysed in BD FACSVerse™ (10
000 cells per sample). DMSO treated cells were used as a vehicle control. Data are presented as dot plots. Cells stained with calcein-AM alone (live cells) or ethidium homodimer alone (dead cells) or both are presented as a bar diagram with error bars.
DNA fragmentation assay. DNA fragmentation during apoptotic induction by MH1 on Molt4 cells was studied using DNA fragmentation assay.20b Briefly, Molt4 cells were incubated with increasing concentrations of MH1 (0, 1, 5, 10 and 20 μM for 48 h), harvested, washed in PBS and lysed in lysis buffer (100 mM NaCl, 10 mM Tris pH 8.0, 0.25% Triton X-100, 1 mM EDTA and 100 μg ml−1 proteinase K for 4 h at 55 °C). Following RNase treatment (50 μg ml−1, for 1 h at 37° C), and deproteinization (phenol: chloroform extraction), DNA was precipitated, washed with 70% ethanol, dried and dissolved in TE. DNA concentration was estimated, and equal concentration was loaded on 2% agarose gel and electrophoresed at 50 V for 3 h. DNA hyper ladder I was used as a marker to assess the length of the DNA. Quantification of HMW (high molecular weight) DNA and fragmented DNA was carried out using Multi Gauge V3.0 software and presented as a bar diagram with error bars (n = 2).
Measurement of mitochondrial membrane potential assay. To test the involvement of mitochondrial machinery in induction of apoptosis, JC-1 staining was carried out.39 Briefly, Molt4 cells treated with different concentrations of MH1 (0, 1, 5, 10 and 20 μM) were harvested at 48 h, incubated with JC-1 dye (5,5′,6,6′-tetrachloro-1,1,3,3′-tetraethylbenzimidazolcarbocyanamide iodide; 2 μM; Calbiochem, USA) for 30 min at 37 °C, washed and immediately analysed in BD FACSVerse™. Cells treated with 2,4-DNP was used as a positive control, and cells treated with DMSO alone was served as a vehicle control. Data are presented as dot plots, percentage cells with high, intermediate and low mitochondrial membrane potential (MMP) are presented in bar diagram with error bars based on minimum of two experiments.
Western blot analysis. Effect of MH1 on the expression of apoptotic proteins was analysed on Molt4 cells following MH1 treatment by Western blot analysis. Cell lysate was prepared after 48 h of MH1 treatment (0, 1, 5 and 10 μM) using RIPA buffer method as described previously.40 ∼30 μg of protein from each sample was resolved on a SDS-PAGE (12%), transferred to PVDF membrane (Millipore, USA) and probed with respective primary, biotinylated secondary and streptavidin HRP antibodies. The primary antibodies used were BAX, BCL2, and Actin (from Invitrogen, USA); CASPASE9 and CASPASE3 (from Santacruz, USA). Membranes were developed using chemiluminescent reagents (Bio-Rad, USA) and imaged were acquired using gel documentation system (LAS 3000, Fuji, Japan). Actin served as loading control. Quantification of the Western blots were carried out using Multi Gauge V3.0 software and presented as a bar diagram in fold changes with respect to the control with error bars (n = 2).
Molecular docking studies. 2D structure of MH1 was sketched in ChemBioDraw Ultra 14.0. Explicit hydrogen were added and converted into 3D structure using Open Babel 2.3.2
41 (http://www.openbabel.org). 3D crystal structure of double stranded DNA bound with Hoechst 33258 at minor groove was retrieved from protein databank (PDB ID: 127D, http://www.rcsb.org/pdb). Molecular docking studies were carried out with Autodock4.2 program.42 Briefly, ligand was removed from the DNA PDB structure and PDBQT files were generated for DNA molecule and MH1. Grid files were created keeping the grid centres 15, 21.23 and 8.45 on X, Y and Z dimensions, respectively (npts = 60 for X, Y and Z, spacing = 0.375). Rigid docking was carried out using Lamarckian genetic algorithm (GA run was kept 25 with long search parameter). Best pose was selected according to lower binding energy and presented in the final image. Images were created with PyMOL (http://www.pymol.org/).
MH1–DNA interaction studies. DNA interaction studies were carried out using calf thymus (CT) DNA (Sigma) dissolved in 20 mM Tris–HCl, pH 7.4 with 15 mM NaCl and 0.5 mM EDTA (all the assays were performed using same buffer). Concentration of CT-DNA was determined by UV-spectroscopy using the extinction coefficient 12
824 M−1 cm−1.
UV-Vis spectroscopy. MH1–DNA interaction studies were carried out using UV-Vis spectrometer (Perkin-Elmer, Lambda 35).43 Briefly, MH1 (10 μM) was scanned (200 nm to 550 nm) with different concentration of CT-DNA (0, 1, 5, 10, 25, 50, 75 and 100 μM) with a scan speed of 240 nm min−1, three cycles keeping the scan intervals of 0.5 nm and 2 nm slit width. Resulting absorbance (A) was plotted with the function of wavelength.
Fluorescence emission spectrum measurement. Fluorescence emission studies44 of MH1–DNA interactions were carried out in 96 well plate microplate reader Infinite M200PRO (Tecan Group Ltd., Switzerland). Briefly, 1 μM MH1 was added with the different concentration of CT-DNA (0, 1, 5, 10, 25, 50, 75 and 100 μM) and scanned for the emission spectra from 300 to 550 nm at 259 nm excitation range (instrument settings: z-position – 20
000 μm, gain-100, integration time – 20 μs and number of flashes-25 with 1 nm step). Resulting fluorescence intensity (RFU) is plotted as the function of wavelength.
DNA dye displacement assay. To check the mode of MH1 binding to DNA, we have carried out DNA dye displacement assay using Hoechst 33258 or ethidium bromide dye.45 Briefly, 20 μM CT-DNA along with 5 μM Hoechst 33258 was incubated with different concentration of MH1 (0, 1, 5, 10, 25, 50 and 100 μM) for 10 min at room temperature, emission spectra was scanned from 400 to 650 nm at excitation of 352 nm. 50 μM MH3 was also used to check its ability to displace the Hoechst as it showed poor cytotoxicity ex vivo. Fluorescence intensity from compound alone was also measured and used for the subsequent subtraction in the final graph. Similarly, ethidium bromide (1 μM) displacement assay was also carried out (emission range from 530 to 780 at 510 nm excitation) and emission spectra was plotted as described previously.
Circular dichroism. In order to check the DNA topological changes upon binding with MH1, circular dichroism studies were performed.46 Calf thymus DNA (50 μM) was incubated with different concentrations of MH1 (0, 10, 25, 50 and 100 μM) and circular dichroism (CD) spectra were recorded at room temperature from 220 to 300 nm with five cycles for every sample using a JASCO J-810 spectropolarimeter (scan speed of 100 nm min−1). Buffer with compound alone was subtracted from corresponding spectra and final graph was presented. Hoechst 33258 (10 μM) was used as a positive control.
Topoisomerase assay. Ability of MH1 to inhibit topoisomerase activity was checked using recombinant human topoisomerase I and human topoisomerase II α (TopoGEN.Inc, USA). Supercoiled plasmid DNA (pBS-SK+) was isolated using GenElute plasmid miniprep kit (Sigma), according to the manufactures instructions. ∼250 ng of plasmid DNA was incubated with different concentrations of MH1 (0, 10, 25, 50 and 100 μM, at RT for 5 min; equal amount of DMSO was added in controls). To this, topoisomerase enzyme (2 units) was added in the supplied reaction buffer (30 min incubation at 37 °C). Reactions were terminated by addition of stop buffer containing 5% sarkosyl, 0.125% bromophenol blue, 25% glycerol and resolved on 0.8% agarose gel (40 V, 4 h). Following ethidium bromide post staining, gel images were captured. Etoposide (100 μM) and Topotecan (7.5 μM) were served as a positive control for topoisomerase II α and topoisomerase I, respectively. Experiments were carried out a minimum of two independent times.
In vitro transcription assay. To have an insight on other DNA related activities, we performed in vitro transcription assay using T7 RNA polymerase (NEB, USA).47 Briefly, 500 ng of pBS-SK+ plasmid DNA was incubated with different concentrations of MH1 (0, 10, 25, 50 and 100 μM) at room temperature for 5 min (equal amount of DMSO was added in the control reactions), in presence T7 RNA polymerase (5 units). Reactions were incubated for 30 min at 37 °C, terminated and further processed as described above in “topoisomerase assay”. RNase A (50 μg ml−1) was used to confirm the synthesised RNA species.
Statistical analysis. The error bars were expressed as mean ± SEM. Statistical analysis was performed using One-way ANOVA followed by Dunnett test and significance was calculated after comparing each value with control using GraphPad software prism 5.1. The values were considered as statistically significant, if the p-value was equal to or less than 0.05 (0.05*, 0.005**, 0.0005***).
Conflict of interest
Authors disclose that there is no conflict of interest.
Acknowledgements
We thank Dr M. Nambiar and other members of SCR laboratory for discussions and comments on the manuscript. We thank NMR, Confocal and FACS facility at IISc for their help. This work was supported by grants from DST (F.NO.SR/SO/HS-006/2010 (G) Dated 29.08.2011) to KSR and SCR, IISc-DBT partnership programme [DBT/BF/PR/INS/2011-12/IISc] to SCR. MH is supported by Junior Research Fellowship from DST-PURSE program.
References
-
(a) M. D. Moen, K. McKeage, G. L. Plosker and M. A. Siddiqui, Imatinib: a review of its use in chronic myeloid leukaemia, Drugs, 2007, 67(2), 299–320 CrossRef CAS PubMed;
(b) A. Paul and S. Bhattacharya, Chemistry and biology of DNA-binding small molecules, Curr. Sci., 2012, 102(2) CAS.
-
(a) D. Henderson and L. H. Hurley, Molecular struggle for transcriptional control, Nat. Med., 1995, 1(6), 525–527 CrossRef CAS PubMed;
(b) L. H. Hurley, DNA and its associated processes as targets for cancer therapy, Nat. Rev. Cancer, 2002, 2(3), 188–200 CrossRef CAS PubMed;
(c) D. A. Anthoney and C. J. Twelves, DNA: still a target worth aiming at? A review of new DNA-interactive agents, Am. J. PharmacoGenomics, 2001, 1(1), 67–81 CrossRef CAS PubMed.
- X. Cai, P. J. Jr Gray and D. D. von Hoff, DNA minor groove binders: back in the groove, Cancer Treat. Rev., 2009, 35(5), 437–450 CrossRef CAS PubMed.
-
(a) J. W. Lown, DNA recognition by lexitropsins, minor groove binding agents, J. Mol. Recognit., 1994, 7(2), 79–88 CrossRef CAS PubMed;
(b) B. S. Reddy, S. M. Sondhi and J. W. Lown, Synthetic DNA minor groove-binding drugs, Pharmacol. Ther., 1999, 84(1), 1–111 CrossRef CAS PubMed.
-
(a) J. Mann, A. Baron, Y. Opoku-Boahen, E. Johansson, G. Parkinson, L. R. Kelland and S. Neidle, A new class of symmetric bisbenzimidazole-based DNA minor groove-binding agents showing antitumor activity, J. Med. Chem., 2001, 44(2), 138–144 CrossRef CAS PubMed;
(b) M. H. Helal, Z. A. Al-Mudaris, M. H. Al-Douh, H. Osman, H. A. Wahab, B. O. Alnajjar, H. H. Abdallah and A. M. Abdul Majid, Diaminobenzene schiff base, a novel class of DNA minor groove binder, Int. J. Oncol., 2012, 41(2), 504–510 CAS;
(c) Z. A. Al-Mudaris, A. S. Majid, D. Ji, B. A. Al-Mudarris, S. H. Chen, P. H. Liang, H. Osman, S. K. J. Din and A. M. Abdul Majid, Conjugation of benzylvanillin and benzimidazole structure improves DNA binding with enhanced antileukemic properties, PLoS One, 2013, 8(11), e80983 Search PubMed;
(d) A. Vidal, C. Munoz, M. J. Guillen, J. Moreto, S. Puertas, M. Martinez-Iniesta, A. Figueras, L. Padulles, F. J. Garcia-Rodriguez, M. Berdiel-Acer, M. A. Pujana, R. Salazar, M. Gil-Martin, L. Marti, J. Ponce, D. G. Mollevi, G. Capella, E. Condom, F. Vinals, D. Huertas, C. Cuevas, M. Esteller, P. Aviles and A. Villanueva, Lurbinectedin (PM01183), a new DNA minor groove binder, inhibits growth of orthotopic primary graft of cisplatin-resistant epithelial ovarian cancer, Clin. Cancer Res., 2012, 18(19), 5399–5411 CrossRef CAS PubMed;
(e) A. Fedier, C. Fowst, J. Tursi, C. Geroni, U. Haller, S. Marchini and D. Fink, Brostallicin (PNU-166196)–a new DNA minor groove binder that retains sensitivity in DNA mismatch repair-deficient tumour cells, Br. J. Cancer, 2003, 89(8), 1559–1565 CrossRef CAS PubMed;
(f) S. Neidle, DNA minor-groove recognition by small molecules, Nat. Prod. Rep., 2001, 18(3), 291–309 RSC.
- P. L. Hamilton and D. P. Arya, Natural product DNA major groove binders, Nat. Prod. Rep., 2012, 29(2), 134–143 RSC.
- R. M. Jr Brosh, J. K. Karow, E. J. White, N. D. Shaw, I. D. Hickson and V. A. Bohr, Potent inhibition of Werner and bloom helicases by DNA minor groove binding drugs, Nucleic Acids Res., 2000, 28(12), 2420–2430 CrossRef.
-
(a) T. A. Beerman, M. M. McHugh, R. Sigmund, J. W. Lown, K. E. Rao and Y. Bathini, Effects of analogs of the DNA minor groove binder Hoechst 33258 on topoisomerase II and I mediated activities, Biochim. Biophys. Acta, 1992, 1131(1), 53–61 CrossRef CAS PubMed;
(b) J. M. Woynarowski, M. McHugh, R. D. Sigmund and T. A. Beerman, Modulation of topoisomerase II catalytic activity by DNA minor groove binding agents distamycin, Hoechst 33258, and 4′,6-diamidine-2-phenylindole, Mol. Pharmacol., 1989, 35(2), 177–182 CAS.
-
(a) L. Garuti, M. Roberti and G. Bottegoni, Benzimidazole derivatives as kinase inhibitors, Curr. Med. Chem., 2014, 21(20), 2284–2298 CrossRef CAS PubMed;
(b) S. P. Velagapudi, S. J. Seedhouse, J. French and M. D. Disney, Defining the RNA internal loops preferred by benzimidazole derivatives via 2D combinatorial screening and computational analysis, J. Am. Chem. Soc., 2011, 133(26), 10111–10118 CrossRef CAS PubMed.
- S. Demirayak, U. Abu Mohsen and A. Cagri Karaburun, Synthesis and anticancer and anti-HIV testing of some pyrazino[1,2-a]benzimidazole derivatives, Eur. J. Med. Chem., 2002, 37(3), 255–260 CrossRef CAS PubMed.
- G. Ayhan-Kilcigil, C. Kus, T. Coban, B. Can-Eke and M. Iscan, Synthesis and antioxidant properties of novel benzimidazole derivatives, J. Enzyme Inhib. Med. Chem., 2004, 19(2), 129–135 CrossRef CAS PubMed.
- D. Sharma, B. Narasimhan, P. Kumar, V. Judge, R. Narang, E. de Clercq and J. Balzarini, Synthesis, antimicrobial and antiviral activity of substituted benzimidazoles, J. Enzyme Inhib. Med. Chem., 2009, 24(5), 1161–1168 CrossRef CAS PubMed.
- V. C. Emery and A. F. Hassan-Walker, Focus on new drugs in development against human cytomegalovirus, Drugs, 2002, 62(13), 1853–1858 CrossRef CAS PubMed.
- B. V. Kumar, S. D. Vaidya, R. V. Kumar, S. B. Bhirud and R. B. Mane, Synthesis and anti-bacterial activity of some novel 2-(6-fluorochroman-2-yl)-1-alkyl/acyl/aroyl-1H-benzimidazoles, Eur. J. Med. Chem., 2006, 41(5), 599–604 CrossRef CAS PubMed.
-
(a) Z. Kazimierczuk, M. Andrzejewska, J. Kaustova and V. Klimesova, Synthesis and antimycobacterial activity of 2-substituted halogenobenzimidazoles, Eur. J. Med. Chem., 2005, 40(2), 203–208 CrossRef CAS PubMed;
(b) S. A. Stanley, S. S. Grant, T. Kawate, N. Iwase, M. Shimizu, C. Wivagg, M. Silvis, E. Kazyanskaya, J. Aquadro, A. Golas, M. Fitzgerald, H. Dai, L. Zhang and D. T. Hung, Identification of novel inhibitors of M. tuberculosis growth using whole cell based high-throughput screening, ACS Chem. Biol., 2012, 7(8), 1377–1384 CrossRef CAS PubMed.
-
(a) T. D. Penning, G. D. Zhu, V. B. Gandhi, J. Gong, X. Liu, Y. Shi, V. Klinghofer, E. F. Johnson, C. K. Donawho, D. J. Frost, V. Bontcheva-Diaz, J. J. Bouska, D. J. Osterling, A. M. Olson, K. C. Marsh, Y. Luo and V. L. Giranda, Discovery of the poly(ADP-ribose) polymerase (PARP) inhibitor 2-[(R)-2-methylpyrrolidin-2-yl]-1H-benzimidazole-4-carboxamide (ABT-888) for the treatment of cancer, J. Med. Chem., 2009, 52(2), 514–523 CrossRef CAS PubMed;
(b) D. Hao, J. D. Rizzo, S. Stringer, R. V. Moore, J. Marty, D. L. Dexter, G. L. Mangold, J. B. Camden, D. D. von Hoff and S. D. Weitman, Preclinical antitumor activity and pharmacokinetics of methyl-2-benzimidazolecarbamate (FB642), Invest. New Drugs, 2002, 20(3), 261–270 CrossRef CAS PubMed;
(c) H. M. Refaat, Synthesis and anticancer activity of some novel 2-substituted benzimidazole derivatives, Eur. J. Med. Chem., 2010, 45(7), 2949–2956 CrossRef CAS PubMed;
(d) R. Abonia, E. Cortes, B. Insuasty, J. Quiroga, M. Nogueras and J. Cobo, Synthesis of novel 1,2,5-trisubstituted benzimidazoles as potential antitumor agents, Eur. J. Med. Chem., 2011, 46(9), 4062–4070 CrossRef CAS PubMed.
- P. Chaudhuri, B. Ganguly and S. Bhattacharya, An experimental and computational analysis on the differential role of the positional isomers of symmetric bis-2-(pyridyl)-1H-benzimidazoles as DNA binding agents, J. Org. Chem., 2007, 72(6), 1912–1923 CrossRef CAS PubMed.
- S. Bhattacharya and P. Chaudhuri, Medical implications of benzimidazole derivatives as drugs designed for targeting DNA and DNA associated processes, Curr. Med. Chem., 2008, 15(18), 1762–1777 CrossRef CAS PubMed.
-
(a) G. M. Raghavendra, A. B. Ramesha, C. N. Revanna, K. N. Nandeesh, K. Mantelingu and K. S. Rangappa, One-pot tandem approach for the synthesis of benzimidazoles and benzothiazoles from alcohols, Tetrahedron Lett., 2011, 52(43), 5571–5574 CrossRef CAS;
(b) K. S. Sharath Kumar, T. R. Swaroop, K. B. Harsha, K. H. Narasimhamurthy and K. S. Rangappa, T3P®-DMSO mediated one pot cascade protocol for the synthesis of 4-thiazolidinones from alcohols, Tetrahedron Lett., 2012, 53(42), 5619–5623 CrossRef CAS.
-
(a) J. A. Collins, C. A. Schandi, K. K. Young, J. Vesely and M. C. Willingham, Major DNA fragmentation is a late event in apoptosis, J. Histochem. Cytochem., 1997, 45(7), 923–934 CrossRef CAS PubMed;
(b) S. Kumar, M. Hegde, V. Gopalakrishnan, V. K. Renuka, S. A. Ramareddy, E. de Clercq, D. Schols, A. K. Gudibabande Narasimhamurthy, S. C. Raghavan and S. S. Karki, 2-(4-Chlorobenzyl)-6-arylimidazo[2,1-b][1,3,4]thiadiazoles: synthesis, cytotoxic activity and mechanism of action, Eur. J. Med. Chem., 2014, 84, 687–697 CrossRef CAS PubMed.
- E. Gottlieb, S. M. Armour, M. H. Harris and C. B. Thompson, Mitochondrial membrane potential regulates matrix configuration and cytochrome c release during apoptosis, Cell Death Differ., 2003, 10(6), 709–717 CrossRef CAS PubMed.
- L. C. Tu, C. S. Chen, I. C. Hsiao, J. W. Chern, C. H. Lin, Y. C. Shen and S. F. Yeh, The beta-carboline analog Mana-Hox causes mitotic aberration by interacting with DNA, Chem. Biol., 2005, 12(12), 1317–1324 CrossRef CAS PubMed.
- N. Shahabadi and M. Maghsudi, Multi-spectroscopic and molecular modeling studies on the interaction of antihypertensive drug; methyldopa with calf thymus DNA, Mol. BioSyst., 2014, 10(2), 338–347 RSC.
- W. C. Tse and D. L. Boger, A fluorescent intercalator displacement assay for establishing DNA binding selectivity and affinity, Current Protocols in Nucleic Acids Chemistry, 2005, ch. 8, unit 8.5, DOI:10.1002/0471142700.nc0805s20, PubMed PMID: 18428943.
-
(a) D. S. Pilch, Z. Xu, Q. Sun, E. J. Lavoie, L. F. Liu, N. E. Geacintov and K. J. Breslauer, Characterizing the DNA binding modes of a topoisomerase I-poisoning terbenzimidazole: evidence for both intercalative and minor groove binding properties, Drug Des. Discovery, 1996, 13(3–4), 115–133 CAS;
(b) J. Portugal, Berenil acts as a poison of eukaryotic topoisomerase II, FEBS Lett., 1994, 344(2–3), 136–138 CrossRef CAS PubMed.
- P. Majumder, A. Banerjee, J. Shandilya, P. Senapati, S. Chatterjee, T. K. Kundu and D. Dasgupta, Minor groove binder distamycin remodels chromatin but inhibits transcription, PLoS One, 2013, 8(2), e57693 CAS.
- J. L. Nitiss, Targeting DNA topoisomerase II in cancer chemotherapy, Nat. Rev. Cancer, 2009, 9(5), 338–350 CrossRef CAS PubMed.
- M. Zihlif, D. R. Catchpoole, B. W. Stewart and L. P. Wakelin, Effects of DNA minor groove binding agents on global gene expression, Cancer Genomics Proteomics, 2010, 7(6), 323–330 CAS.
-
(a) B. Maji and S. Bhattacharya, Advances in the molecular design of potential anticancer agents via targeting of human telomeric DNA, Chem. Commun., 2014, 50(49), 6422–6438 RSC;
(b) B. Maji and S. Bhattacharya, Molecular design of synthetic benzimidazoles for the switchover of the duplex to G-quadruplex DNA recognition, Chimia, 2013, 67(1–2), 39–43 CrossRef CAS PubMed.
-
(a) M. Nambiar, M. Srivastava, V. Gopalakrishnan, S. K. Sankaran and S. C. Raghavan, G-quadruplex structures formed at the HOX11 breakpoint region contribute to its fragility during t(10;14) translocation in T-cell leukemia, Mol. Cell. Biol., 2013, 33(21), 4266–4281 CrossRef CAS PubMed;
(b) M. Nambiar, G. Goldsmith, B. T. Moorthy, M. R. Lieber, M. V. Joshi, B. Choudhary, R. V. Hosur and S. C. Raghavan, Formation of a G-quadruplex at the BCL2 major breakpoint region of the t(14;18) translocation in follicular lymphoma, Nucleic Acids Res., 2011, 39(3), 936–948 CrossRef CAS PubMed;
(c) V. K. Katapadi, M. Nambiar and S. C. Raghavan, Potential G-quadruplex formation at breakpoint regions of chromosomal translocations in cancer may explain their fragility, Genomics, 2012, 100(2), 72–80 CrossRef CAS PubMed.
-
(a) S. Bhattacharya, P. Chaudhuri, A. K. Jain and A. Paul, Symmetrical bisbenzimidazoles with benzenediyl spacer: the role of the shape of the ligand on the stabilization and structural alterations in telomeric G-quadruplex DNA and telomerase inhibition, Bioconjugate Chem., 2010, 21(7), 1148–1159 CrossRef CAS PubMed;
(b) A. K. Jain, V. V. Reddy, A. Paul, K. Muniyappa and S. Bhattacharya, Synthesis and evaluation of a novel class of G-quadruplex-stabilizing small molecules based on the 1,3-phenylene-bis(piperazinyl benzimidazole) system, Biochemistry, 2009, 48(45), 10693–10704 CrossRef CAS PubMed;
(c) A. K. Jain, A. Paul, B. Maji, K. Muniyappa and S. Bhattacharya, Dimeric 1,3-phenylene-bis(piperazinyl benzimidazole)s: synthesis and structure-activity investigations on their binding with human telomeric G-quadruplex DNA and telomerase inhibition properties, J. Med. Chem., 2012, 55(7), 2981–2993 CrossRef CAS PubMed.
- T. R. Cech, Life at the End of the Chromosome: Telomeres and Telomerase, Angew. Chem., Int. Ed. Engl., 2000, 39(1), 34–43 CrossRef CAS.
- S. O. Kim, K. Sakchaisri, N. R. Thimmegowda, N. K. Soung, J. H. Jang, Y. S. Kim, K. S. Lee, Y. T. Kwon, Y. Asami, J. S. Ahn, R. L. Erikson and B. Y. Kim, STK295900, a dual inhibitor of topoisomerase 1 and 2, induces G(2) arrest in the absence of DNA damage, PLoS One, 2013, 8(1), e53908 CAS.
- M. Rangarajan, J. S. Kim, S. P. Sim, A. Liu, L. F. Liu and E. J. Lavoie, Topoisomerase I inhibition and cytotoxicity of 5-bromo- and 5-phenylterbenzimidazoles, Bioorg. Med. Chem., 2000, 8(11), 2591–2600 CrossRef CAS PubMed.
- M. Hegde, S. S. Karki, E. Thomas, S. Kumar, K. Panjamurthy, S. R. Ranganatha, K. S. Rangappa, B. Choudhary and S. C. Raghavan, Novel levamisole derivative induces extrinsic pathway of apoptosis in cancer cells and inhibits tumor progression in mice, PLoS One, 2012, 7(9), e43632 CAS.
-
(a) M. Srivastava, M. Hegde, K. K. Chiruvella, J. Koroth, S. Bhattacharya, B. Choudhary and S. C. Raghavan, Sapodilla plum (Achras sapota) induces apoptosis in cancer cell lines and inhibits tumor progression in mice, Sci. Rep., 2014, 4, 6147 CrossRef CAS PubMed;
(b) C. V. Kavitha, M. Nambiar, C. S. Ananda Kumar, B. Choudhary, K. Muniyappa, K. S. Rangappa and S. C. Raghavan, Novel derivatives of spirohydantoin induce growth inhibition followed by apoptosis in leukemia cells, Biochem. Pharmacol., 2009, 77(3), 348–363 CrossRef CAS PubMed.
- K. K. Chiruvella, V. Kari, B. Choudhary, M. Nambiar, R. G. Ghanta and S. C. Raghavan, Methyl angolensate, a natural tetranortriterpenoid induces intrinsic apoptotic
pathway in leukemic cells, FEBS Lett., 2008, 582(29), 4066–4076 CrossRef CAS PubMed.
- A. Kummrow, M. Frankowski, N. Bock, C. Werner, T. Dziekan and J. Neukammer, Quantitative assessment of cell viability based on flow cytometry and microscopy, Cytometry, Part A, 2013, 83(2), 197–204 CrossRef CAS PubMed.
- S. Kumar, V. Gopalakrishnan, M. Hegde, V. Rana, S. S. Dhepe, S. A. Ramareddy, A. Leoni, A. Locatelli, R. Morigi, M. Rambaldi, M. Srivastava, S. C. Raghavan and S. S. Karki, Synthesis and antiproliferative activity of imidazo[2,1-b][1,3,4]thiadiazole derivatives, Bioorg. Med. Chem. Lett., 2014, 24(19), 4682–4688 CrossRef CAS PubMed.
- R. R. Somasagara, M. Hegde, K. K. Chiruvella, A. Musini, B. Choudhary and S. C. Raghavan, Extracts of strawberry fruits induce intrinsic pathway of apoptosis in breast cancer cells and inhibits tumor progression in mice, PLoS One, 2012, 7(10), e47021 CAS.
- N. M. O'Boyle, M. Banck, C. A. James, C. Morley, T. Vandermeersch and G. R. Hutchison, Open babel: an open chemical toolbox, J. Cheminf., 2011, 3, 33 Search PubMed.
- G. M. Morris, R. Huey, W. Lindstrom, M. F. Sanner, R. K. Belew, D. S. Goodsell and A. J. Olson, AutoDock4 and AutoDockTools4: automated docking with selective receptor flexibility, J. Comput. Chem., 2009, 30(16), 2785–2791 CrossRef CAS PubMed.
- M. S. Shahabuddin, M. Gopal and S. C. Raghavan, Intercalating, cytotoxic, antitumour activity of 8-chloro and 4-morpholinopyrimido [4′,5′:4,5]thieno(2,3-b)quinolines, J. Photochem. Photobiol., B, 2009, 94(1), 13–19 CrossRef CAS PubMed.
- X. B. Fu, D. D. Liu, Y. Lin, W. Hu, Z. W. Mao and X. Y. Le, Water-soluble DNA minor groove binders as potential chemotherapeutic agents: synthesis, characterization, DNA binding and cleavage, antioxidation, cytotoxicity and HSA interactions, Dalton Trans., 2014, 43(23), 8721–8737 RSC.
-
(a) B. C. Baguley, W. A. Denny, G. J. Atwell and B. F. Cain, Potential antitumor agents. 34. Quantitative relationships between DNA binding and molecular structure for 9-anilinoacridines substituted in the anilino ring, J. Med. Chem., 1981, 24(2), 170–177 CrossRef CAS PubMed;
(b) Y. Matsuba, H. Edatsugi, I. Mita, A. Matsunaga and O. Nakanishi, A novel synthetic DNA minor groove binder, MS-247: antitumor activity and cytotoxic mechanism, Cancer Chemother. Pharmacol., 2000, 46(1), 1–9 CrossRef CAS PubMed.
- M. Srivastava, M. Nambiar, S. Sharma, S. S. Karki, G. Goldsmith, M. Hegde, S. Kumar, M. Pandey, R. K. Singh, P. Ray, R. Natarajan, M. Kelkar, A. De, B. Choudhary and S. C. Raghavan, An inhibitor of nonhomologous end-joining abrogates double-strand break repair and impedes cancer progression, Cell, 2012, 151(7), 1474–1487 CrossRef CAS PubMed.
- K. Yu, F. Chedin, C. L. Hsieh, T. E. Wilson and M. R. Lieber, R-loops at immunoglobulin class switch regions in the chromosomes of stimulated B cells, Nat. Immunol., 2003, 4(5), 442–451 CrossRef CAS PubMed.
Footnote |
† Electronic supplementary information (ESI) available. See DOI: 10.1039/c5ra16605e |
|
This journal is © The Royal Society of Chemistry 2015 |