DOI:
10.1039/C5RA09594H
(Paper)
RSC Adv., 2015,
5, 74753-74763
Insulating oxide film formation with acid catalyzed hydrolysis of alkoxide precursors in supercritical fluid carbon dioxide†
Received
21st May 2015
, Accepted 18th August 2015
First published on 19th August 2015
Abstract
Insulating oxide films can be produced by hydrolysis of metal alkoxide precursors in the presence of an acid catalyst in supercritical fluid carbon dioxide (sc-CO2). Using tetraethylorthosilicate (TEOS) as a precursor and acetic acid (HAc) as a catalyst, uniform SiO2 films can be formed on surfaces of different substrates according to the reaction Si(OCH2CH3)4 + 2H2O → SiO2 + 4CH3CH2OH. The quality of the SiO2 film is controlled by the rate of hydrolysis of TEOS which is determined by the amount of water available in the system. In our sc-CO2 reaction system, water involved in the TEOS hydrolysis is generated by the in situ esterification process CH3COOH + C2H5OH → CH3COOC2H5 + H2O. In the absence of acetic acid, the reaction proceeds very slowly. The acid catalyzed reaction probably involves proton coordination to the oxygen atoms of TEOS molecules that facilitates the hydrolysis. The acid-catalyzed hydrolysis reaction produces dense SiO2 films instead of porous SiO2 films formed by water added hydrolysis of TEOS in sc-CO2. Formation of SiO2 films via hydrolysis in sc-CO2 is more rapid compared to the traditional hydrolysis reaction at room temperature. In general, metal alkoxide hydrolysis reactions carried out in a closed sc-CO2 system is not affected by moisture in air compared with traditional open-air hydrolysis systems. Using sc-CO2 as a reaction medium also eliminates undesirable organic solvents utilized in traditional alkoxide hydrolysis reactions.
1. Introduction
Stability of the optical properties of nanoparticle arrays is important for optical device fabrication and for their durability. For example, without protection, PbS quantum dot (QD) arrays would degrade by oxidation over long periods of time. In addition, techniques for making uniform and pinhole free insulator oxide films are important for fabricating devices where electrical isolation is a key factor. Stouwdam et al.1 grew an ∼1 nm shell of PbS on a PbSe core to stabilize it with respect to oxidation. However, the observed shifts and reduction in photoluminescence (PL) signals upon air exposure indicated that the PbSe core was not effectively protected by the PbS shell from oxidation. Ihly and co-workers2 used room-temperature atomic layer deposition (ALD) to infill the internal pore network of PbS QD films with amorphous alumina and then cap the films with a thin alumina (Al2O3) coating, to prevent QD oxidation. This infilling method can produce QD films with greatly enhanced photo thermal stability. Nanu et al.3 applied atomic layer chemical vapor deposition (AL-CVD) to deposit CuInS2 inside the pores of nanostructured TiO2, in which it acquires a nanometer scaled interpenetrating network between TiO2 and CuInS2. Pourret and Sionnest4 applied ZnO to infiltrate CdSe QD films using ALD at 100 °C and increase film conductivity and carrier mobility. These important findings regarding short term and long term changes in the morphology, chemical state, and optical properties of PbS QDs illustrate the necessity of additional technological advances in post-deposition coating and treatment methods so as to protect the QDs and maintain their stability. Recently, our group has explored the formation of silicon oxide films in supercritical fluid carbon dioxide (sc-CO2) by hydrolysis of a silicon alkoxide precursor in the presence of an acid catalyst.5,6 With tetraethylorthosilicate (TEOS) as a precursor and acetic acid (HAc) as a catalyst, thin silica films on semiconductor substrates were formed in sc-CO2. The optical properties of nanoparticle arrays coated with thin SiO2 films in sc-CO2 are used as an example to illustrate one of the applications of this technique.
CO2 is widely used for supercritical fluid studies because of its moderate critical constants, non-flammable nature, low cost, and available in relatively pure form. Above the critical point, CO2 becomes a fluid, which has both gas-like diffusivity and liquid-like density. The solvating strength can be tuned by adjusting temperature and pressure. The advantages of utilizing sc-CO2 as a green solvent for nanotechnology applications are given in recent reports5–9 on direct sol–gel processing of making SiO2 films and in a review10 on applications of sc-CO2 in materials processing and synthesis. The solvents used in traditional TEOS hydrolysis reactions usually are alcohols, THF, acetone, etc. The sc-CO2 technology not only eliminates traditional toxic organic solvents, but also increases reaction rates kinetically. The closed high pressure supercritical fluid system allows precisely control of water content or moisture of the system which is difficult to achieve in open air reaction systems. This report describes conditions of formation of silicon dioxide films with reasonable thickness in sc-CO2 using TEOS and HAc. Parameters of the SiO2 film formation including amount of catalyst, presence of water, molar ratio of HAc/TEOS, reaction time, temperature and pressure of sc-CO2, were evaluated. The properties of the resulting material that are of interest to coating applications including morphology and composition are presented in this paper. Formation of SiO2 protective coating on nanoparticle arrays in sc-CO2 and related spectroscopic studies are also presented.
2. Experimental
2.1 Chemicals
Tetraethylorthosilicate (TEOS), reagent grade 98% was purchased from Sigma-Aldrich, and acetic acid (HAc) (glacial, ACS reagent, w/w, min 99.7%) was purchased from Fischer Scientific.
Hexanes, toluene, ethanol, methanol, hydrogen tetrachloroaurate(III) trihydrate (99.99%), NaCNBH3 (95%), and sodium bis(2-ethylhexyl)sulfosuccinate (AOT, 98%) were purchased from Aldrich and used as received. Chromium (Cr) coated glass was made by sputtering in our laboratory. A CdSe(core)/ZnS(shell) nanoparticle solution was obtained from one of our collaborators Professor Jian Xu's research group in Pennsylvania State University. Instrument-grade carbon dioxide (purity: 99.99%) was obtained from Airgas, USA (http://www.airgas.com). Purified water was produced by means of distillation.
2.2 Synthesis and preparation of nanoparticles
Gold particles were prepared by reduction of gold (Au) ions suspended in AOT water-in-hexane microemulsions. Separate metal ion/reducing agent solutions were prepared by dissolving 0.0178 g of AOT in 2 mL of hexane and adding an aqueous solution of 7.2 μL (water/surfactant ratio, W = 10) of either the 1.2 M NaCNBH3 reducing agent or the 0.4 M Au3+ ion. The W value was manipulated by the amount of aqueous solution added. These micellar solutions were stirred for 1 h before reduction to equilibrate the reagents to the reaction temperature. The reaction was at an ambient temperature. The microemulsion containing the reducing agent was added dropwise over a time span of 60 seconds to the gold ion-containing microemulsion under vigorous stirring. Dodecanethiol (70 μL) was added to the reaction immediately after all of the reducing agent had been added to the solution. This solution was then allowed to stir at the reaction temperature for another hour. After this time, the gold particles were precipitated by adding a mixture of 6 mL of ethanol and 4 mL of methanol, followed by centrifugation. The supernatant was discarded, and the remaining particles were washed two more times with 6 mL of ethanol to remove AOT, spectator ions, and excess dodecanethiol. The particles were then re-suspended in 1 mL of toluene. All procedures were conducted on the benchtop without the need for inert environments. Detailed procedure can be found in the literature.11
2.3 Sample preparation, deposition and chemical reactions in sc-CO2
2.3.1 Preparations of Cr coated glass. The glass substrates (8 × 8 mm2) were sputtered and coated with Cr thin layer in a Denton DV 502 RF magnetron sputter chamber. The substrates were loaded into the sputter chamber and the system was pumped down to a base vacuum of 5 × 10−6 Torr. The chamber was then backfilled with Ar to a pressure of 7 × 10−3 Torr. The sputtering was initiated at a power of 250 W and a deposition rate of 1 Å s−1. Sputtering time was approximately 2.5 min with a thickness of 150 Å.
2.3.2 Deposition of nanoparticles and chemical reaction in sc-CO2.
2.3.2.1 Deposition of nanoparticles in sc-CO2. Supercritical carbon dioxide precipitation of the nanoparticles was carried out inside a 14 mL chamber of a stainless steel high-pressure apparatus. Temperature was controlled by a culture control incubator manufactured by Boekel Scientific Inc. and monitored by a thermocouple. An ISCO syringe pump was used to introduce CO2 and control the pressure. A small vial was placed in the cell containing a piece of Cr coated glass with 200 μL of the Au nanoparticle colloid synthesized previously. The apparatus was sealed and slowly charged with 60 atm of CO2 at room temperature over a period of 10 min. The chamber was then brought to a pressure of 70 atm. The entire apparatus was brought to 40 °C to bring the carbon dioxide in the reaction chamber into the supercritical state. During this heating, the pressure inside the chamber rose to roughly 140 atm. Then, the ISCO pump was used to raise the pressure up to a final pressure of 170 atm. During this period, the carbon dioxide mixes with the toluene, changing its polarity and causing precipitation of the nanoparticles. The high-pressure apparatus was left at this condition of 40 °C and 170 atm for 30 min to ensure an equilibrium state for the one-phase sc-CO2/toluene solution. Finally, the chamber was vented slowly (0.2 mL min−1) into a graduated cylinder placed in a fume hood removing toluene and CO2, and leaving the nanoparticles on the bottom of the substrate.
2.3.2.2 Hydrolysis reaction of TEOS in sc-CO2. After obtaining the nanoparticle layer on the substrate, the sample was ready for SiO2 film deposition. Samples for both room temperature and sc-CO2 reactions were prepared in a nitrogen glove box which was previously purged by N2 gas to remove moisture and air. TEOS (50 μL, 2.24 × 10−4 mol) and HAc (40 μL, 7.04 × 10−4 mol) were spiked separately into different mini glass beakers. Pieces of substrate were loaded on the top of platform and a stainless steel high pressure chamber cap was then immediately sealed. The reaction chamber was installed into sc-CO2 system, and then charged with CO2 from 60 atm to 70 atm, and heated from room temperature to 50 °C, which took about 1 hour. At this time, the pressure inside the chamber was approximately 160 atm. After that, CO2 was then charged again from 160 atm to 170 atm. The system was in the static mode for 2 hours, followed by depressurization with a flow rate of 0.1–0.3 mL min−1. The high pressure reaction chamber used in the experiments is illustrated in Fig. 1.
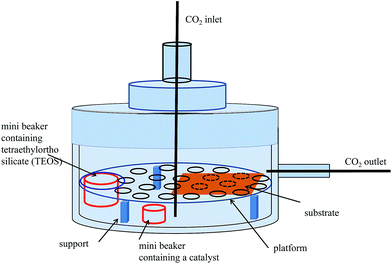 |
| Fig. 1 Supercritical fluid CO2 high pressure reaction chamber. | |
2.4 Instruments and characterizations
2.4.1 Cross section cutting, SEM and EDS measurements. Tescan LYRA-3 Model XMH FIB instrument was used for cross section cutting. A platinum (Pt) cap was deposited by chemical vapor deposition to protect the SiO2 layer from ion beam damage. Cross section cutting used a focused ion beam (FIB) with a 30 keV Ga to produce a few micrometers deep cross section, which includes the Pt protection layer, SiO2 layer, sample layer such as Au nanoparticle layer, and Cr coated glass layer or GaAs substrate.Furthermore, a FEI Sirion scanning electron microscope (SEM) instrument was used to measure the cross section and the image taking. SEM images were collected with a Sirion instrument manufactured by FEI, Inc. The following parameters were used for the SEM field emission gun (FEG): an accelerating voltage of 5 kV, a spot size of 3.0, and a working distance of ∼4.5 mm. During the measurement of the cross section of the films, dynamic focus and tilt correction were applied and specimen tilt was set at 45°.
Energy dispersive spectroscopy (EDS) was employed to measure elemental data using instrument of FEI Quanta ESEM II.
2.4.2 TEM measurements. Carbon coated copper grids purchased from Ted Pella were used to prepare SiO2 films in a close chamber from hydrolysis reaction of TEOS and to obtain TEM images. A Phillips CM 200 LaB6 (lanthanum hexaboride) cathode transmission electron microscope operating at 200 kV was used for both low and high resolution imaging.
2.4.3 ATR-FTIR, UV-Vis-NIR and XPS. Attenuated total reflectance (ATR) in conjunction with Fourier transform infrared (FTIR) spectroscopy was used to identify the Si–O–Si vibrational modes. Thermo Scientific Spectra Tech in Nicolet 6700 FTIR in conjunction with Omnic Thermo Electron Corporation Version 7.3 Software was used. ATR enables samples to be examined directly in the solid or liquid state without further preparation.UV-Vis-NIR spectra were obtained on a Cary 5000 Varian UV-Vis-NIR spectrophotometer scanning from 200 to 1600 nm.
The X-ray photoelectron spectroscopy (XPS) spectra were acquired on a Perkin-Elmer Phi 5400 system, equipped with a nonmonochromatic magnesium X-ray source and a hemispherical sector photoelectron energy analyzer, with the extraction lens accepting signal from a 1.1 mm diameter area. XPS is a surface analysis technique, which only measures near surface layers. If the SiO2 film thickness exceeds 5–10 nm, XPS photoelectrons emitted from nanoparticles beneath the SiO2 film cannot be detected. The thickness of the SiO2 films fabricated by us in this study is 30 nm or greater. We have performed XPS and it only shows the information about the SiO2 film. The XPS spectra are included in the ESI† of this paper.
3. Results and discussion
3.1 Chemistry of TEOS hydrolysis reaction
3.1.1 Effect of water. A traditional method of making SiO2 film is by hydrolysis of TEOS with water,12,13 according to the following reaction: |
Si(OCH2CH3)4 + 2H2O → SiO2 + 4CH3CH2OH
| (1) |
Preliminary benchtop experiments were carried out in our laboratory in a close system with TEOS and water placed separately next to a carbon coated copper grid with the presence of acetic acid catalyst. The hydrolysis reaction was efficient in the presence of water, even at room temperature, based upon our EDS and SEM information. However, the SiO2 films generated from TEOS by this method are porous in the entirety of the SiO2 layer. Fig. 2a shows TEM images of SiO2 film layer from the reaction of the TEOS and a small amount (a few microliters) of water in the presence of a catalyst (HAc). Even this small amount of excess water can cause the hydrolysis to go too fast according to Reaction (1) and results in a porous low bulk density and high particulate aerogel structure. High resolution 29Si NMR studies14 showed the kinetics of hydrolysis and condensation reactions could be remarkably accelerated with addition of high water concentrations. In a closed high pressure sc-CO2 system, one source of water is from CO2 which has a purity of 99.99%. This source of water is very small. The main source of water for the hydrolysis is from in situ esterification reaction between HAc and the hydrolysis product C2H5OH and condensation reactions shown in Reactions (2–4).
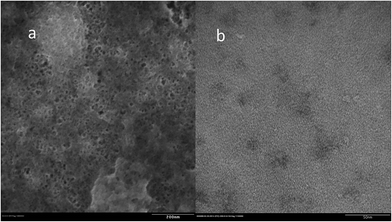 |
| Fig. 2 TEM images of the SiO2 layer from the hydrolysis reaction of TEOS (a) with addition of water and (b) without addition of water. | |
If no water was is added to the system, the water required for TEOS hydrolysis may come from in situ water generation15 from the esterification reaction
|
CH3COOH + C2H5OH → CH3COOC2H5 + H2O
| (2) |
and the condensation reactions.
|
Si–OH + Si–OH → Si–O–Si + H2O
| (3) |
|
Si–OH + Si–OC2H5 → Si–O–Si + C2H5OH
| (4) |
These reactions could moderate the hydrolysis rate. Uniform SiO2 films were produced (Fig. 2b) on TEM grids when using only TEOS and acetic acid without additional water. In the sc-CO2 system, water may also be obtained from CO2 medium, which usually contains 1 × 10−2 mol fraction of water at 50 °C and 200 atm, a common supercritical fluid experimental condition.16 Therefore, the water moisture from in situ generation and from the CO2 phase is enough for the TEOS hydrolysis to form decent SiO2 films.
Restricting the availability of water and moderating the hydrolysis rate can significantly improve the SiO2 film quality. Water generated in situ through the esterification and condensation reactions, thus, provides a better alternative for the formation of high quality insulating silica films from alkoxide precursors (Fig. 2b).
3.1.2 Effect of catalyst. In the absence of the acetic acid catalyst at room temperature, the TEOS hydrolysis reaction is extremely slow, as revealed by our EDS measurements. Pope and Mackenzie17 reported that TEOS gelation time will take 1000 hours at room temperature if there is no catalyst. The authors also pointed out when acetic acid was applied, the TEOS gelation time could shorten to 72 hours.17 Acetic acid is very soluble in CO2,7 approximately 10% of acetic acid by mol can be dissolved in sc-CO2 at 40 °C (313 K) and 89 atm (9 MPa) and is an in situ water producing reactant in the esterification reaction (Reaction (2)). Our benchtop experiments showed in the presence of HAc catalyst, the TEOS took more than 72 hours (3 to 4 days) to complete the hydrolysis reaction.More importantly, sc-CO2 is a good solvent for metal alkoxides. In addition, CO2 molecules, which are linearly symmetric, are known to interact with carbonyl groups and bridged acetate groups in a Lewis acid and Lewis-base bonding mode, thus the intermediates and colloidal particles with these CO2-philic moieties can be stabilized in sc-CO2.9,18
The higher acid/alkoxide ratio increases the rate of TEOS hydrolysis reaction.19 In our experiments, as observed from the cross sections of SEM images, however, if an excess amount of acid was added in the catalytic hydrolysis of alkoxide, the pre-deposited nanoparticle layer, e.g., Au nanoparticle layer, could be damaged showing some porous structures. Moreover, when an excess amount of acid was used in the acid catalyzed hydrolysis, GaAs substrate surfaces started to peel, generating flakes. This was observed at molar ratio of HAc/TEOS ≥ 7.8, for example: HAc = 1.049 × 10−4 mol and TEOS = 1.34 × 10−5 mol. On the other hand, if the moisture in CO2 and catalyst are totally absent, the hydrolysis reaction would be extremely slow.
Fig. 3 shows TEM images of SiO2 films obtained from a room temperature hydrolysis reaction and both films in Fig. 3a and b appear to be uniform. SiO2 films formation in carbon coated copper grids were acquired at a fixed amount of TEOS (1.34 × 10−5 mol, 3 μL) and different amount of HAc [3.5 × 10−5 mol (2 μL) and 7.0 × 10−5 mol (4 μL)]. The hydrolysis reaction was undertaken at room temperature in 25 mL glass containers in which TEM grids were pre-loaded. The glass containers were left unattended for 5 days.
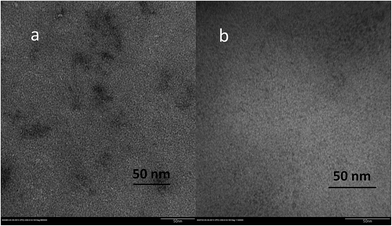 |
| Fig. 3 TEM images of SiO2 film formation (a) TEOS (1.34 × 10−5 mol, 3 μL) + HAc (3.5 × 10−5 mol, 2 μL), magnification = 88 000×, (b) TEOS (1.34 × 10−5 mol, 3 μL) + HAc (7.0 × 10−5 mol, 4 μL), magnification = 115 000×. | |
EDS spectral results are also supportive of the resulting products which show O
:
Si ratio around 2
:
1, at a fixed TEOS of 1.34 × 10−5 mol (3 μL) and acetic acid varied from 3.5 × 10−5 mol (2 μL) to 1.75 × 10−4 mol (10 μL) in a 25 mL-glass container (Fig. 4). In this experiment, GaAs substrate was employed. Although the SiO2 formula is represented with only one silicon atom and two oxygen atoms, it is actually a giant covalent molecular lattice with each silicon atom attached to four oxygen atoms and each oxygen atom attached to two silicon atoms. The simplest formula is SiO2. When 3.5 × 10−5 mol (2 μL) of HAc were added, the Si and O peaks in EDS spectra were barely observable, indicating the hydrolysis reaction was just initiated. The atomic ratios showed a consistent trend of O
:
Si = 2
:
1 when more HAc were added to the system sequentially, suggesting that SiO2 film was formed (Fig. 4).
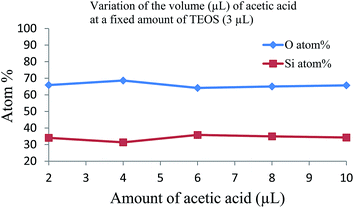 |
| Fig. 4 Percentage of atomic numbers of O: Si in EDS spectra, after the hydrolysis reaction on GaAs substrate. | |
Overall, in this study, water moisture and catalyst are two critical factors to lead the TEOS hydrolysis reaction. However, the amount of water required needs to be well controlled, as is the amount of catalyst.
3.2 SiO2 film formation in sc-CO2 with the TEOS hydrolysis reaction
3.2.1 Advantages of conducting hydrolysis reaction in sc-CO2. The TEOS hydrolysis reactions belong to the category of sol–gel processes. Recent developments in sol–gel processes have used either organic solvents or sc-CO2 fluid for synthesizing a number of metal oxides.8,9,19–21 Supercritical CO2 is a good solvent for alkoxides and short chain organic acids (e.g., HCOOH and CH3COOH).19 The reaction temperature in sc-CO2 phase must be greater than its critical temperature (31 °C). A commonly used temperature in sc-CO2 is ≥40 °C, while organic solvents used in sol–gel processes are in room temperature. Thus, sc-CO2 process would generally accelerate chemical reaction rates relative to room temperature reactions.The advantages of utilizing sc-CO2 as a medium for depositing materials in nanostructures are well recognized in the literature.22–25 The ability of sc-CO2 to dissolve certain solutes and penetrate into small holes plus easy separation of solute from the fluid by manipulating temperature and pressure are not achievable by liquid or gas phase deposition methods. Several previous studies reported formation of SiO2 films in sc-CO2 by hydrolysis of TESO and water. For example, mesoporous silica films were synthesized by Vogt et al.26 using TEOS and water in preformed polymeric templates using either gaseous CO2 or supercritical CO2 as the reaction medium. In their studies, introduction of the precursor was either through vapor pressure (utilizing the vapor pressure of TEOS in gaseous CO2) or through sc-CO2 (dissolving TEOS in sc-CO2). They found that reaction in sc-CO2 produced uniform ordered porous films, as compared to reaction in compressed CO2 gas.26 The solubilities of organic compounds in CO2 typically increase considerably at a temperature greater than 31 °C (the critical temperature, Tc) when the pressure is raised above the critical pressure (Pc = 72.8 atm).27 A decrease of TEOS solubility in the gaseous CO2 phase would reduce the reaction rate in the formation of the templated film.26 The authors also found that in the process of synthesis of mesoporous silica films, the pore sizes were doubled when the synthesis conditions changed from gaseous to supercritical fluid CO2.26 Ghosh and co-workers28,29 also conducted similar experiments, i.e. SiO2 film formation via hydrolysis of TEOS, using CO2 under gaseous (68 atm, 45 °C), liquid (170 atm, 25 °C) and supercritical fluid (102–170 atm, 45 °C) conditions. In their experiments, the density of sc-CO2 (0.544–0.779 g mL−1) is much higher than that of gaseous CO2 (0.178 g mL−1). They found the pore diameters for SiO2 thin films templated with 3 different surfactants in sc-CO2 were larger than that in gaseous CO2. Pore expansion in the mesoporous silica films increases with CO2 processing pressures, as well as with surfactant's tail length and branch. This is attributed to the solvating strength of the CO2-philic fluorinated tail of the surfactants in sc-CO2; while in gas phase, due to the low density of the gaseous CO2, the distance between CO2 and precursor molecules are much larger, resulting in a low reaction rate.
Controlling water moisture which determines reaction rate is a key factor to produce good SiO2 films. In our acid catalyzed hydrolysis, the water required in the hydrolysis is mainly acquired from in situ esterification and condensation reactions plus a trace amount from the CO2 phase.
In our experimental conditions, the amounts of TEOS (2.245 × 10−4 mol) and HAc (7.04 × 10−4 mol) dissolved in sc-CO2 fluid at temperature 50 °C are higher than their amount present in the gas phase based on the known vapor pressures calculated from the Ideal Gas Law. In addition, solvation strength of sc-CO2 can be tuned by manipulating pressure to enhance the solubility of precursors in sc-CO2. Under our sc-CO2 experimental conditions, the amounts of TEOS and HAc dissolved in the fluid phase are at least 10 times greater than the concentrations they can attain in gaseous CO2 phase based on their known vapor pressure data at 50 °C. Apparently, sc-CO2 has advantages over gas CO2 to be used as a reaction medium for higher concentration precursors.
3.2.2 Hydrolysis of TEOS in sc-CO2 and SiO2 film formation. In a high-pressure chamber, the alkoxide precursors are transformed into sols through condensation reaction in sc-CO2. These sols can form aerogel via supercritical CO2 drying, in which the interface between the liquid and vapor phases is eliminated. Sui and Charpentier carried out TEOS hydrolysis experiments in a 100 mL autoclave equipped with ATR-FTIR in sc-CO2.19 The volumes of TEOS and HAc added were both 2 mL, calculated from the initial concentrations of TEOS and HAc, and the volume of high pressure system. The total reaction times undertaken were 6 h, under the temperature of 40–60 °C and pressure of 88.4–204 atm. At 50 °C and 88.4–204 atm, after 200 min (3.3 h), TEOS hydrolysis conversion reached a plateau, suggesting the hydrolysis reaction conversions were complete.19 Based upon information from the literature, in our experiments, the conditions used were as follows: temperature of 50 °C, pressure of 170 atm, and the hydrolysis reaction in sc-CO2 in 2 hours, which should be appropriate to complete the conversions of hydrolysis reactions when the volumes of TEOS and HAc used were in the level of microliters. The completion of the reaction could also be confirmed by lack of acetic acid odor when the reaction chamber was opened after the reactions. We chose 50 °C for the hydrolysis reaction, because above this temperature nanoparticle might undergo changes during the SiO2 coating process.Charpentier and co-workers8 synthesized and formed silica aerogel particles by a sol–gel route in sc-CO2. They noticed that increasing the concentration of acid and addition of extra water led to an acceleration of the reaction. By slowing down the rate of the reactions, precipitation and agglomeration of particles could be minimized. Fig. 5 shows SiO2 film formation on GaAs substrate in sc-CO2. The reason to use GaAs substrate is due to the facts that it will have less interference with background information related to SiO2 and it is a commonly used semiconductor material for many applications. Fig. 5a and b show EDS spectrum and SEM image of blank GaAs substrate. After hydrolysis reaction in sc-CO2, SiO2 film was formed as evidenced by O and Si peaks in EDS spectrum (Fig. 5c) and SEM image (Fig. 5d). In this reaction, there is no exterior water added. In the presence of acetic acid as a catalyst, a smooth silicon dioxide film with reasonable thickness can be formed in supercritical fluid CO2 at temperatures at 50 °C and 170 atm (Fig. 5d).
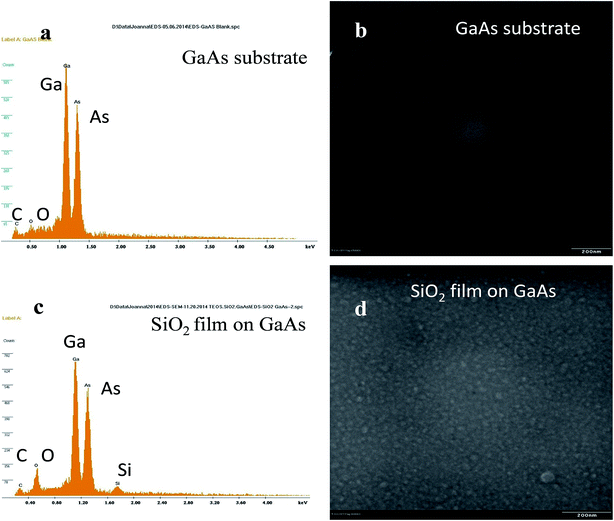 |
| Fig. 5 SiO2 film formation on GaAs substrate in sc-CO2. (a) EDS spectrum of GaAs substrate, (b) SEM image of GaAs substrate, (c) EDS spectrum of SiO2 film on GaAs substrate, and (d) SEM image of SiO2 film on the surface of GaAs substrate. | |
The reaction kinetics of sol–gel reactions in sc-CO2 depends on pressure, in addition to reaction temperature and reactant concentration. The conversion of TEOS was studied as a function of temperature and pressure in sc-CO2 using in situ FTIR and a chemometrics modeling technique.19 It was found that a higher temperature and low pressure would facilitate the conversion in the ranges of 40 °C to 60 °C (313 K–333 K) and 88 atm to 204 atm (1300 psi–3000 psi), respectively.19 The effect of the temperature on the kinetics is consistent with Arrhenius equation k = Ae−Ea/(RT) (k: rate constant, T: absolute temperature, A: the pre-exponential factor, Ea: the activation energy, R: the universal gas constant). A higher local concentration of acetic acid with TEOS will increase esterification reaction rate, thus enhancing the hydrolysis reaction rate.
Attenuated total reflectance (ATR) is a sampling technique used in conjunction with FTIR spectroscopy which enables samples to be examined directly in the solid or liquid state without further preparation. A sample fabricated on GaAs substrate with 30 μL TEOS and 30 μL of HAc, through the hydrolysis reactions of TEOS in sc-CO2 at 50 °C, 170 atm and 2 hours was measured by ATR-FTIR spectra, which revealed a characteristic Si–O–Si peak located clearly at 1066.1 cm−1, and supported the SiO2 film formation, as shown in Fig. 6.
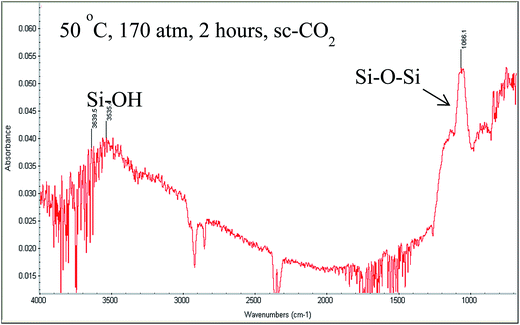 |
| Fig. 6 ATR-FTIR spectrum of SiO2 film fabricated on GaAs substrate with 30 μL TEOS and 30 μL HAc in sc-CO2 at 50 °C and 170 atm. Si–O–Si vibrational mode is located near 1066 cm−1. | |
3.3 SiO2 film thickness
Tescan LYRA-3 Model XMH FIB instrument was used for cross section cutting. After cutting, a FEI Sirion SEM instrument was used to measure the cross section and the imaging. The cross sections of the films include Cr coated glass layer or GaAs substrate, with a nanoparticle layer, SiO2 film layer, and Pt protective layer. The reason to use Cr coated glass as a substrate is to meet a requirement for the SEM measurements and to let charges be drained with less imaging interferences. For the purpose of the study of SiO2 film formation with acid catalyzed hydrolysis of TEOS in sc-CO2, the SiO2 film deposited directly on the substrates without the nanoparticle layer will be discussed in this section.
Preliminary experiments of SiO2 deposition via TEOS hydrolysis was carried out using benchtop experiments at room temperature. Fig. 7a shows the cross sections of SiO2 films generated from TEOS hydrolysis, on the top of GaAs substrates, with 3 μL of TEOS (1.34 × 10−5 mol) and 6 μL of acetic acid (1.05 × 10−4 mol) in a close system. The average thickness of the films was around 81 nm. In addition, Cr coated glass substrate was also used to check silica film layer via TEOS hydrolysis in sc-CO2 and the average thickness of the cross section of SiO2 film was approximately 385 nm (Fig. 7b), under a condition of 2.24 × 10−4 mol (50 μL) TEOS, 7.04 × 10−4 (40 μL) HAc, and sc-CO2 at 50 °C, 170 atm.
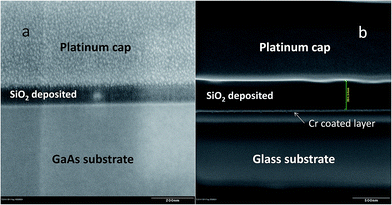 |
| Fig. 7 Cross sections of SEM images of SiO2 film generated from TEOS hydrolysis (a) on the top of GaAs substrates, in a benchtop experiment, and magnification is 100 000×, and (b) on the top of Cr coated glass substrate, under a condition of 50 μL TEOS, 40 μL HAc and sc-CO2, and magnification is 35 000×. | |
One way to control the SiO2 film thickness is by controlling the amount of catalyst addition. A set of benchtop experiments was conducted in a nitrogen glove box. 25 mL glass vials were used as sealed systems. The amount of TEOS was fixed at 1.34 × 10−5 mol (3 μL), and the amount of acetic acid was changing from 3.5 × 10−5 mol (2 μL) to 1.75 × 10−4 mol (10 μL). GaAs wafers were used as substrates. The benchtop reactions took approximately 4–5 days to complete at room temperature. When 3.5 × 10−5 mol (2 μL) of acetic acid were used, the thickness of the cross section of the SiO2 layer was around 29 nm. As the amount of HAc was doubled (4 μL), the thickness of the silica layer was almost doubled, 45 nm, as shown in Fig. 8. When the amount of HAc added reached a certain level (6 μL), it was shown that the thickness of the silica film approach a maximum (81 nm), and the plot showed a pseudo-linear relationship at this point. If acetic acid continued to increase to 8 μL or 10 μL, then a slight decline of the thickness from the curve was observed (Fig. 8). The average cross sections of the film thickness are 29, 45, 81, 73, and 73 nm for the images of Fig. 8a–e, respectively.
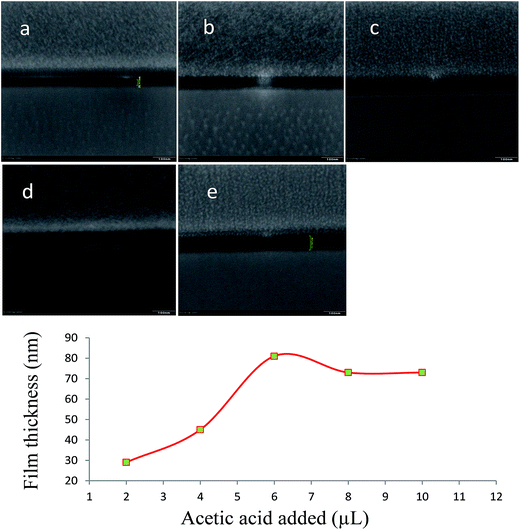 |
| Fig. 8 SEM images of SiO2 film thickness measurements. At a fixed TEOS amount (1.34 × 10−5 mol, 3 μL), variation of the amount of acetic acid from (a) 3.5 × 10−5 mol (2 μL), (b) 7.0 × 10−5 mol (4 μL), (c) 1.05 × 10−4 mol (6 μL), (d) 1.4 × 10−4 mol (8 μL), to (e) 1.75 × 10−4 mol (10 μL). The bottom curve shows SiO2 film thickness changes with the amount of acetic acid added. The magnifications in the cross sections of SEM images are all in 120 000×. | |
3.4 Spectroscopy studies of SiO2 protective coating on the surface of nanoparticles
A piece of Cr coated glass (Cr layer thickness: 15 nm) was used as a substrate to deposit Au nanoparticles in supercritical fluid CO2. Cr coated glass substrates were used instead of GaAs because (1) the sample can be characterized by UV-Vis spectroscopy and (2) it allows for a charge drainage for the EDS/SEM measurements. The deposition was carried out in 40 °C and 170 atm. The deposited Au sample was then measured by an UV-Vis spectroscopy in which spectra indicated a big hump around 450 nm from the background of Cr coated glass (not shown) and a maximum absorbance wavelength from Au nanoparticles at 561 nm, as shown in Fig. 9. This Au sample was then deposited with SiO2 film via TEOS hydrolysis reaction in the same reaction medium, i.e., supercritical fluid CO2. Due to a thin coating of SiO2 (approximately ∼390 nm), the UV-Vis spectrum is still able to provide Au nanoparticle information, i.e., a maximum absorbance wavelength at 561 nm, except raising the entire background slightly (Fig. 9). The Au deposited sample was also measured by EDS spectra before (Fig. 9a) and after (Fig. 9b) TEOS hydrolysis reaction in sc-CO2. In Fig. 9a for Au nanoparticle deposited SiO2 film, around 1.70 and 2.18 keV, there are two Au peaks, and the height of the former is almost not noticeable compared with the latter. The major peak is located at 2.18 keV. The peak of oxygen (O) around 0.5 keV is almost indistinguishable. After the TEOS hydrolysis reaction in supercritical fluid CO2, as shown in Fig. 9b, the Au peaks at 1.70 and 2.18 keV have been changed to approximately 1
:
1 ratio and the O peak at 0.5 keV was significantly increased compared with the carbon (C) peak at 0.28 keV located nearby. Si peak was nearly superimposed with that of the first Au peak at 1.70 keV as shown in Fig. 9b. We assume the Au peaks won't be changed with time. The net value of atomic ratio for Si at 1.70 keV and O at 0.50 keV is 1
:
2, according to a quantitative EDS result, indicating the SiO2 passivation layer was produced on the top of Au array without interfering Au film spectroscopic measurements.
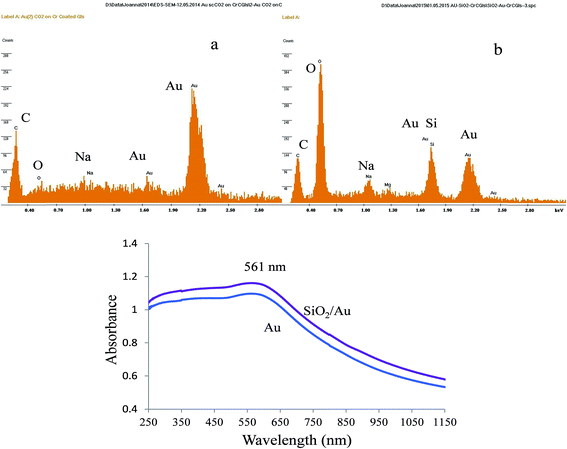 |
| Fig. 9 EDS spectra of (a) Au film deposited on Cr coated glass in sc-CO2, and (b) SiO2 thin film covered on the top of Au layer surface in sc-CO2. UV-Vis spectra show spectral information of Au deposited on Cr coated glass and SiO2 coated on the top of Au nanoparticle layer on Cr coated glass substrate. | |
A similar experiment was also carried out using CdSe(core)/ZnS(shell) nanoparticles. The CdSe/ZnS nanoparticle array deposited on the Cr coated glass substrate has a maximal UV-Vis absorbance around 583 nm (Fig. 10). After the UV-Vis measurement, the sample was then taken for TEOS hydrolysis reaction in sc-CO2 and then measured by UV-Vis spectroscopy again. Fig. 10 shows the film has an identical maximal absorbance at 583 nm, except the background of the SiO2 coated CdSe/ZnS film is somewhat higher. The sample was also measured by EDS before (Fig. 10a) and after (Fig. 10b) the TEOS hydrolysis reaction in sc-CO2. It was found that there were some oxygen and sodium existing in the original sample (Fig. 10a), which probably came from the starting nanomaterials based upon EDS elemental analysis. After TEOS hydrolysis reaction in sc-CO2, the EDS spectrum of the film (Fig. 10b) shows Si peak at 1.7 keV and O peak at 0.5 keV, accordingly. The oxygen peak from SiO2 overlapped with the original oxygen peak from the CdSe/ZnS sample. However, after subtracting this part, the net atomic ratio of O to Si in the SiO2/CdSe film sample was 2
:
1, suggesting the SiO2 film was coated on the top of CdSe/ZnS film.
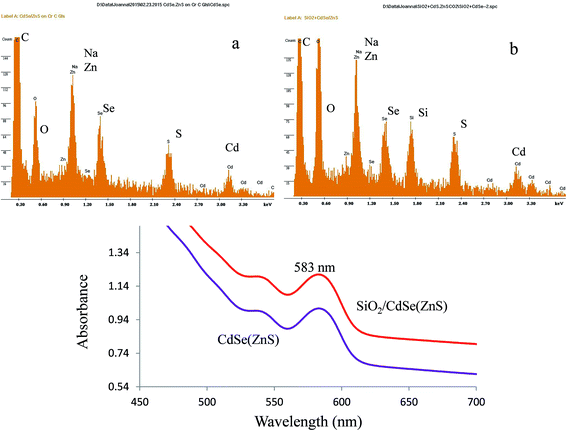 |
| Fig. 10 EDS spectra of (a) CdSe/ZnS (core/shell) nanoparticle film deposited on Cr coated glass in sc-CO2, and (b) SiO2 thin film covered on top of the CdSe/ZnS layer in sc-CO2. UV-Vis spectra of CdSe/ZnS nanoparticles deposited on Cr coated glass and SiO2 coated on top of the CdSe/ZnS nanoparticle layer completed in sc-CO2. | |
The results showed that nanoparticle array can be deposited on Cr coated glass in supercritical fluid CO2. TEOS hydrolysis reaction produced a thin passivation silica coating in the same reaction medium on the top of nanoparticle layer, which can be successfully measured by spectroscopy without intervention. This study shows that SiO2 films formed in sc-CO2 provide an alternative approach to protect nanomaterials from oxidation and ripening/sintering and to maintain the stabilities of nanomaterials and nanostructured devices.
4. Conclusion
Formation of SiO2 films by acetic acid catalyzed hydrolysis of TEOS in supercritical fluid CO2 was studied. In this process, no extra water, organic solvents or other additives were required. Parameters such as amount of catalyst, water, molar ratio of HAc/TEOS, reaction time, temperature and pressure of sc-CO2 were evaluated. The high pressure supercritical fluid CO2 sealed system restricts the exterior water moisture, thus reducing the hydrolysis reaction rate. A trace amount of water generated from in situ esterification and condensation reactions and from CO2 tanks produces a good quality SiO2 film. Uniform SiO2 films with variable thicknesses can be formed by adjusting relative amounts of TEOS and HAc. Spectra of the nanoparticle layer with SiO2 protective coating can be measured by UV-Vis spectroscopy without any interference. EDS measurements provided quantitative information regarding the atomic ratio of O to Si in the protective coating. Further exploration for the research of the formation of SiO2 protective coating on nanoparticle layers in sc-CO2 and related spectroscopic studies are currently underway.
Acknowledgements
This work is supported by Air Force TOPS IV contract program (FA8650-11-D-5800). We are grateful for the discussion of XPS film studies with Howard E. Smith in Air Force Research Laboratories.
References
- J. W. Stouwdam, J. N. Shan and F. C. J. M. van Veggel, Photostability of colloidal PbSe and PbSe/PbS core/shell nanocrystals in solution and in the solid state, J. Phys. Chem. C, 2007, 111, 1086 CAS.
- R. Ihly, J. Tolentino, Y. Liu, M. Gibbs and M. Law, The photothermal stability of PbS quantum dot solids, ACS Nano, 2011, 5, 8175 CrossRef CAS.
- M. Nanu, J. Schoonman and A. Goossens, Inorganic nanocomposites of n-and p-type semiconductors: a new type of three-dimensional solar cell, Adv. Mater., 2004, 16, 453 CrossRef CAS PubMed.
- A. Pourret, P. G. Sionnest and J. W. Elan, Atomic layer deposition of ZnO in quantum dot thin films, Adv. Mater., 2001, 21, 232 CrossRef PubMed.
- C. M. Wai, H. Ohde and S. J. Kramer, Micron Technology, Inc., Idaho Research Foundation, Formation of insulator oxide films with acid or base catalyzed hydrolysis of alkoxides in supercritical carbon dioxide, US Patent No. 8,241,708 B2, 2012.
- J. S. Wang, C. M. Wai and G. J. Brown, Formation of insulator oxide films with acetic acid catalyzed hydrolysis of alkoxides in supercritical fluid CO2, Abstract of Papers, ACS 248th national conference in San Francisco, CA, Aug 10-15, 2014, COLL-162.
- P. A. Charpentier, X. S. Li and R. H. Sui, Study of the sol-gel reaction mechanism in supercritical CO2 for the formation of SiO2 nanocomposites, Langmuir, 2009, 25, 3748 CrossRef CAS PubMed.
- R. Sui, A. S. Rizkalla and P. A. Charpentier, Synthesis and formation of silica aerogel particles by a novel Sol-Gel route in supercritical carbon dioxide, J. Phys. Chem. B, 2004, 108, 11886 CrossRef CAS.
- R. Sui and P. Charpentier, Synthesis of metal oxide nanostructures by direct sol-gel chemistry in supercritical fluid, Chem. Rev., 2012, 112, 3057 CrossRef CAS PubMed.
- X. Zhang, S. Heinonen and E. Levänen, Applications of supercritical carbon dioxide in materials processing and synthesis, RSC Adv., 2014, 4, 61137 RSC.
- A. B. Smetana, J. S. Wang, J. J. Boeckl, G. J. Brown and M. Wai, Fine-tuning size of gold nanoparticles by cooling during reverse micelle synthesis, Langmuir, 2007, 23, 10429 CrossRef CAS PubMed.
- A. M. Buckley and M. Greenblatt, The sol-gel preparation of silica gels, J. Chem. Educ., 1994, 71, 599 CrossRef CAS.
- B. Karmakar, G. De and D. Ganguli, Dense silica microspheres from organic and inorganic acid hydrolysis of TEOS, J. Non-Cryst. Solids, 2000, 272, 119 CrossRef CAS.
- J. C. Pouxviel, J. P. Boilot, J. C. Beloeil and J. Y. Lalleman, NMR study of the sol/gel Polymerization, J. Non-Cryst. Solids, 1987, 89, 345 CrossRef CAS.
- S. S. Jada, Study of tetraethyl orthosilicate hydrolysis by in situ generation of water, J. Am. Ceram. Soc., 1987, 70, C-298 CrossRef CAS PubMed.
- A. N. Sabirzyanov, A. P. Il'in, A. P. Akhunov and F. M. Gumerov, Solubility of water in supercritical carbon dioxide, High Temp., 2002, 40, 203 CrossRef.
- E. J. A. Pope and J. D. Mackenzie, Sol–gel processing of silica: II. The role of the catalyst, J. Non-Cryst. Solids, 1986, 87, 185 CrossRef CAS.
- R. Sui, J. M. H. Lo and P. A. Charpentier, Infrared and computational studies on interactions of carbon dioxide and titania nanoparticles with acetate groups, J. Phys. Chem. C, 2009, 113, 21022 CAS.
- R. Sui, A. S. Rizkalla and P. A. Charpentier, Kinetic study on the sol-gel reaction in supercritical CO2 by using in situ ATR-FTIR spectrometry, Cryst. Growth Des., 2008, 8, 3024 CAS.
- R. Sui, A. S. Rizkalla and P. A. Charpentier, Formation of titania nanofibers: a direct sol-gel route in supercritical CO2, Langmuir, 2005, 21, 6150 CrossRef CAS PubMed.
- R. Sui, A. S. Rizkalla and P. A. Charpentier, Direct synthesis of zirconia Aerogel nanoarchitecture in supercritical CO2, Langmuir, 2006, 22, 4390 CrossRef CAS PubMed.
- A. B. Smetana, J. S. Wang, J. J. Boeckl, G. J. Brown and C. M. Wai, Deposition of ordered arrays of gold and platinum nanoparticles with an adjustable article size and interparticle spacing using supercritical
CO2, J. Phys. Chem. C, 2008, 112, 2294 CAS.
- J. S. Wang, A. B. Smetana, J. J. Boeckl, G. J. Brown and C. M. Wai, Depositing ordered arrays of metal sulfide nanoparticles in nanostructures using supercritical fluid carbondioxide, Langmuir, 2010, 26, 1117 CrossRef CAS PubMed.
- J. S. Wang, B. Ullrich, G. J. Brown and C. M. Wai, Morphology and energy transfer in PbS quantum dot arrays formed with supercritical fluid deposition, Mater. Chem. Phys., 2013, 141, 195 CrossRef CAS PubMed.
- J. S. Wang, G. J. Brown, W. C. Hung and C. M. Wai, Supercritical fluid deposition of uniform PbS nanoparticle films for energy-transfer studies, ChemPhysChem, 2012, 13, 2068 CrossRef CAS PubMed.
- X. Li and B. D. Vogt, Carbon dioxide mediated synthesis of mesoporous silica films: tuning properties using pressure, Chem. Mater., 2008, 20, 3229 CrossRef CAS.
- K. P. Johnson, D. H. Ziger and C. A. Eckert, Solubilities of hydrocarbon solids in supercritical fluids. The augmented van der Waals treatment, Ind. Eng. Chem. Fundam., 1982, 21, 191 Search PubMed.
- K. Ghosh, S. M. Vyas, H. J. Lehmler, S. E. Rankin and B. L. Knutson, Tailoring porous silica films through supercritical carbon dioxide processing of fluorinated surfactant templates, J. Phys. Chem. B, 2007, 111, 363 CrossRef CAS PubMed.
- K. Ghosh, H. J. Lehmler, S. E. Rankin and B. L. Knutson, Supercritical carbon dioxide processing of fluorinated surfactant templated mesoporous silica thin films, Langmuir, 2005, 21, 61459 Search PubMed.
Footnote |
† Electronic supplementary information (ESI) available. See DOI: 10.1039/c5ra09594h |
|
This journal is © The Royal Society of Chemistry 2015 |