DOI:
10.1039/C4RA10606G
(Paper)
RSC Adv., 2015,
5, 21445-21454
EGFR/HER-2 inhibitors: synthesis, biological evaluation and 3D-QSAR analysis of dihydropyridine-containing thiazolinone derivatives†
Received
24th September 2014
, Accepted 21st January 2015
First published on 21st January 2015
Abstract
A series of dihydropyridine containing thiazolinone derivatives (4a–4r) have been designed, synthesized and their biological activities evaluated as potential EGFR and HER-2 kinase inhibitors and in tumor cell antiproliferation. The synthesized compounds were analyzed by 1H-NMR and MS. In addition, compound 4m was scrutinized by X-ray structure analysis. Among them, compound 4r displayed the most potent inhibitory activity (IC50 = 0.099 μM for EGFR and IC50 = 3.26 μM for HER-2). Antiproliferative assay results indicated that compound 4r owned high antiproliferative activity against B16–F10, HeLa and MCF-7 in vitro, with IC50 values of 0.09 μM, 0.29 μM, and 0.56 μM, respectively. Docking simulations were further performed to position compound 4r into the EGFR active site to determine the probable binding mode. 3D-QSAR models were built for reasonable design of EGFR/HER-2 inhibitors in the present and the future.
1. Introduction
As research moves along, cancer chemotherapy ushers in a new period of vigorous development: molecular-targeted therapeutics have emerged which are highly selective and less toxic compared to traditional drugs.1,2 STI571 exemplifies the successful development of these novel anticancer drugs which are those targeting aberrantly expressed oncogenic growth factor receptors involved in proliferative signal transduction pathways in cancer cells.3,4 Receptor protein tyrosine kinases (RPTKs) play a fundamental role in signal transduction pathways that mediate malignant cell transformation. Epidermal growth factor receptor kinase (EGFR), a transmembrane PTK that is activated by ligand-induced dimerization, plays a key role in the formation and development of many types of solid tumors, including head and neck, lung, breast, bladder, prostate, and kidney cancers. Therefore, EGFR tyrosine kinase represents an attractive target for the development of novel anticancer agents.5,6 EGFR and the homologous HER-2 kinases, which are members of the type 1 or ErbB/HER receptor tyrosine kinase (RTK) family, have emerged as the most viable anticancer molecular targets in this family of four members, EGFR (HER-1/ErbB-1), HER-2 (ErbB-2/neu), HER-3 (ErbB-3), and HER-4 (ErbB-4).2,7,8 Among them, EGFR and HER-2 are the hottest targets in current cancer research and their overexpression or abnormal activation often cause cell malignant transformations, such as non-small cell lung cancer (NSCLC), prostate, breast, stomach, colon, and ovarian cancers.9,10 Compounds that inhibit the kinase activity of EGFR and/or HER-2 after binding of its ATP binding site are of potential interest as new therapeutic antitumor agents.11 Several EGFR inhibitors, the first-generation EGFR inhibitors gefitinib (Iressa™)12 and erlotinib (Tarceva™),13 have been approved by the US FDA for treatment of patients with NSCLC. And lapatinib as a dual reversible EGFR/HER-2 inhibitor was approved for breast cancer therapy.12 Two candidate drugs, afatinib14 and dacomitinib,15 which could bond with a unique cysteine 797 residue located at the lip of the ATP binding cleft,13 as the most promising second generation EGFR kinase inhibitors, have been being evaluated in a respective phase III study16 (Fig. 1).
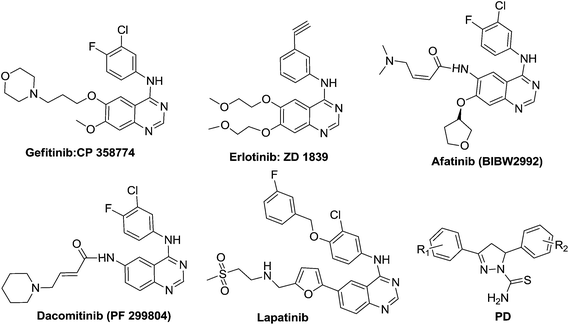 |
| Fig. 1 Selected EGFR/HER-2 inhibitors. | |
In previous studies, pyridine derivatives have been selected as pharmacological agents due to their biological activities and their potential applications.17 The application of this heterocycle is widespread due to its antiviral/antitumor,16,18–20 antibacterial,21,22 antiinflamatory,23 fungistatic,24 and anti-hyperglycemic activity.25
Furthermore, special attention has been placed recently on the use of pyrazoles for their anticancer properties. Recently, in our group, a series of novel pyrazole derivatives containing a thiourea skeleton (PD, Fig. 1) were discovered as potent anticancer agents targeting EGFR TK, and some of them had demonstrated potent antitumor activity.26,27
Thiazolinone and their derivatives have attracted continuing interest over the years because of their varied biological activities, such as anti-inflammatory, antimicrobial, antiproliferative, antiviral, anticonvulsant, antifungal, and antibacterial.28–32 In recent years, thiazolinone derivatives, with their antitumor activity, have become a new hot spot. For example, a thiazolinone-containing benzothiazole moiety has been shown to exert its anticancer activity on leukemia, melanoma, lung, colon, CNS, ovarian, renal, prostate and breast cancer cell lines by Havrylyuk et al.33 Besides these, many pyrazole or thiazolinone derivatives have also been reported with potent biological activities and low toxicities.34–36 Encouraged by these observations we designed and synthesized newer anticancer compound derivatives which contain pyridine, pyrazole and thiazolinone.
Herein, each of the dihydropyridine-containing thiazolinone and pyridine derivatives prepared has been tested for its biological activity as a potential EGFR and HER-2 kinase inhibitor and the results are reported in this paper.
2. Results and discussion
2.1. Chemistry
All novel dihydropyridine-containing thiazolinone (4a–4r) derivatives were synthesized following the synthetic pathway depicted in Scheme 1. The starting diversely-substituted chalcones (2a–2r) were synthesized using pyridinecarboxaldehyde and the substituted acetophenone in the presence of excess sodium hydroxide, using 40% sodium hydroxide as catalyst in water. Then the cyclization reaction of different chalcones with thiosemicarbazide in refluxing ethanol, in the presence of excess sodium hydroxide, afforded the formation of dihydropyridine derivatives containing the thiourea skeleton (3a–3r). Finally, the condensation of dihydropyridine derivatives containing the thiourea skeleton with the appropriate bromoacetic acid and using sodium acetate as an acid-binding agent, stirring at 80 °C for 8 h, resulted in the formation of dihydropyridine-containing thiazolinone derivatives (4a–4r). All of the target compounds gave satisfactory analytical and spectroscopic data, which were in accordance with their depicted structures by 1H NMR, MS.
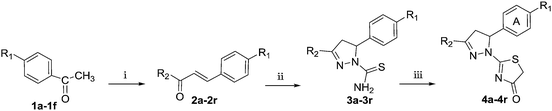 |
| Scheme 1 Reagents and conditions: (i) 1.0 equiv. pyridinecarboxaldehyde, 40% NaOH, ethanol, 0 °C, 2 h, 62.6–78.2%; (ii) 1.0 equiv. thiosemicarbazide, KOH, ethanol, reflux; 12 h, 65.2–72.3%; (iii) bromoacetic acid, acetic anhydride, sodium acetate, acetic acid, 80 °C, 8 h, 47.2–72.8%. | |
2.2. Crystal structure of compound 4m
Among these compounds, the crystal structure of compound 4m was determined by X-ray diffraction analysis. The crystallographic data for the structural analysis are presented in Table 1 and Fig. 2.
Table 1 Crystal data for compound 4m
Compound |
4m |
Empirical formula |
C17H14N4OS |
Formula weight |
322.8 |
Temperature (K) |
273(2) |
Crystal system |
Monoclinic |
Space group |
P![[1 with combining macron]](https://www.rsc.org/images/entities/char_0031_0304.gif) |
a (Å) |
7.7819(12) |
b (Å) |
10.0431(17) |
c (Å) |
10.0631(15) |
α (°) |
99.637(5) |
β (°) |
96.381(5) |
γ (°) |
99.610(5) |
V (Å) |
756.6(2) |
Z |
10 |
Dcalcd/g cm−3 |
1.4151(4) |
θ range (°) |
2.10–27.50 |
F(000) |
336.0 |
Reflections collected |
8193 (Rint = 0.0304) |
Data/restraints/parameters |
3371/0/208 |
Absorption coefficient (mm−1) |
0.710 |
R1; wR2 [I > 2σ(I)] |
0.0444/0.1041 |
R1; wR2 (all data) |
0.0686/0.1142 |
GOF |
1.042 |
Larg. peak/hole (e Å) |
0.28/−0.24 |
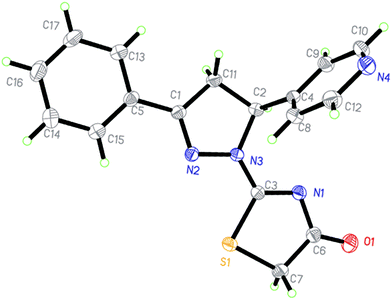 |
| Fig. 2 Crystal structure diagram of compound 4m. | |
2.3. Biological activity
2.3.1. Antiproliferation assay. In the present work, the eighteen newly synthesized derivatives 4a–4r were evaluated for their in vitro growth inhibitory activities against three cultured cell lines, B16–F10, HeLa and MCF-7, in comparison to the known anticancer drugs gefitinib and celecoxib, as reference drugs. The outcomes are summarized in Table 2. As shown in Table 2, the results reveal that most of the synthesized compounds exhibited significant anticancer activities, ranging from 0.09 μM to 8.29 μM, with regard to broad-spectrum antitumor activity. Especially with respect to MCF-7 cell proliferation activity, among them, compound 4f displayed the most potent antitumor activity in MCF-7, with an IC50 of 0.15 μM, compared to the positive control gefitinib (IC50 = 6.70 μM) and celecoxib (IC50 = 7.01 μM). More potent antitumor activity for MCF-7, together with virtual screening results, both indicated that the EGFR might be a potential target that these dihydropyridine-containing thiazolinone derivatives interact with.
Table 2 In vitro anticancer activities (IC50, μM) of compounds 4a–4r against human tumor cell lines
Compounds |
R1 |
R2 |
IC50a (μM) |
B16–F10b |
HeLab |
MCF-7b |
Biological activity was measured using an MTT assay. Values are the average of three independent experiments run in triplicate. Variation was generally 5–10%. Cancer cells kindly supplied by the State Key Laboratory of Pharmaceutical Biotechnology, Nanjing University. |
4a |
H |
 |
0.32 |
1.11 |
1.04 |
4b |
CH3 |
 |
1.88 |
2.39 |
1.96 |
4c |
OCH3 |
 |
4.12 |
5.17 |
3.55 |
4d |
F |
 |
3.75 |
5.21 |
3.71 |
4e |
Cl |
 |
3.24 |
5.68 |
2.55 |
4f |
Br |
 |
0.19 |
0.21 |
0.15 |
4g |
H |
 |
0.26 |
0.61 |
0.87 |
4h |
CH3 |
 |
1.22 |
1.95 |
0.83 |
4i |
OCH3 |
 |
3.41 |
5.19 |
2.64 |
4j |
F |
 |
2.64 |
5.12 |
2.09 |
4k |
Cl |
 |
2.14 |
3.13 |
2.65 |
4l |
Br |
 |
0.41 |
0.11 |
0.23 |
4m |
H |
 |
0.72 |
0.92 |
1.40 |
4n |
CH3 |
 |
2.02 |
3.08 |
1.57 |
4o |
OCH3 |
 |
5.52 |
8.29 |
3.76 |
4p |
F |
 |
3.52 |
5.21 |
2.47 |
4q |
Cl |
 |
3.37 |
5.03 |
2.73 |
4r |
Br |
 |
0.09 |
0.29 |
0.56 |
Gefitinib |
— |
— |
0.10 |
1.59 |
6.70 |
Celecoxib |
— |
— |
5.28 |
7.51 |
7.01 |
2.3.2. EGFR and HER-2 inhibitory activity. All compounds were tested for their EGFR inhibition to EGFR and HER-2 using a solid-phase ELISA assay, with erlotinib as the positive control drug. In this assay, the IC50 values of compounds possessing sufficiently potent anticancer activity are shown in Table 2. The results were compared with those provided by the known anticancer compound erlotinib under identical conditions, which showed that most of the synthesized compounds exhibited significant anticancer activities.The following structure–activity relationship (SAR) can be observed from the data of Table 3, EGFR and HER-2 activities of these compounds were tested against the standard clinically used inhibitor erlotinib. For the majority of the compounds, we found that compounds containing thiazolinone and dihydropyridine rings showed excellent inhibition of EGFR activities, displaying IC50 values between 0.099 and 19.62 μM. Compound 4o was the most active, having an IC50 value of 0.099 μM, whereas compound 4d was the least active, with an IC50 value of 19.62 μM.
Table 3 Inhibition activities of compounds 4a–4r to EGFR and HER-2
Compounds |
IC50a (μM) |
Compounds |
IC50a (μM) |
EGFRb |
HER-2b |
EGFRb |
HER-2b |
Errors were in the range (5–10%) of the reported values, from three different assays. Human recombinant enzymes, by the esterase assay (4-nitrophenylacetate as substrate). |
4a |
5.26 |
6.81 |
4k |
9.63 |
12.38 |
4b |
2.63 |
7.62 |
4l |
5.42 |
5.03 |
4c |
0.30 |
18.62 |
4m |
3.12 |
4.95 |
4d |
19.62 |
20.33 |
4n |
1.23 |
4.83 |
4e |
12.18 |
15.32 |
4o |
0.099 |
3.26 |
4f |
8.32 |
15.32 |
4p |
15.23 |
18.32 |
4g |
4.63 |
5.43 |
4q |
7.28 |
8.63 |
4h |
1.82 |
3.98 |
4r |
5.19 |
5.08 |
4i |
0.18 |
4.88 |
Erlotinib |
0.03 |
0.14 |
4j |
13.26 |
14.12 |
|
|
|
Based on the data obtained, we surveyed a variety of substituents at different positions on the pyridine and A-rings of these compounds 4a–4r (Scheme 1); they could be divided into two subunits: R1 (pyridine ring) and A-rings to discuss how the substituents of these compounds affect the EGFR inhibitory activities. First, we chose different pyridine rings as the research object, and the results showed that most compounds with a nitrogen atom on the 4-position of pyridine ring manifested more potent activities than compounds with a nitrogen atom on the 2-position and 3-position of pyridine ring, such as 4o (IC50 = 0.099 μM) > 4i (IC50 = 0.18 μM) > 4c (IC50 = 0.30 μM); 4q (IC50 = 7.28 μM) > 4k (IC50 = 9.63 μM) > 4e (IC50 = 12.18 μM). In addition, we studied the same pyridine ring and different substituents of the A-rings, and deduced that when the substituent on the para-position of the A-ring was a potent electron-donating group rather than an electron-withdrawing group, the compounds gained better activity. For example 4c (IC50 = 0.30 μM) > 4b (IC50 = 2.63 μM) > 4a (IC50 = 5.26 μM) > 4f (IC50 = 8.32 μM) > 4e (IC50 = 12.18 μM) > 4d (IC50 = 19.2 μM); 4o (IC50 = 0.099 μM) > 4n (IC50 = 1.23 μM) > 4m (IC50 = 3.12 μM) > 4r (IC50 = 5.19 μM) > 4q (IC50 = 7.28 μM) > 4p (IC50 = 15.23 μM). The inhibitory activities increased in the following order: OCH3 > CH3 > H > Br > Cl > F. For the given compounds, it was observed that the IC50 value for inhibition of EGFR kinase was higher than that observed with HER-2 kinase. However, they had the same trends. This was possibly attributed to the fact that a higher concentration of the purified EGFR kinase was used than of the HER-2 kinase in the enzyme assays. It is evident that there is also a reasonable correlation between the EGFR and HER-2 inhibitory activities; thus, this is not surprising in view of the high sequence homology of the catalytic domains of these two kinases.
From the above-mentioned analysis, it could be indicated that thiazolinone, pyridine ring and dihydropyridine rings in the dihydropyridine-containing thiazolinone derivatives might play important roles in the EGFR and HER-2 inhibitory activities.
In comparison, we found that those compounds with electron-donating groups on the A-ring (such as OCH3, CH3), exhibited more potent anticancer activities than those with electron-withdrawing substituents (such as F). From the above-mentioned analysis, it could be concluded that the compounds with a methoxy-substituted A-ring were found to be the most favorable for the anticancer activity.
2.3.3. Cytotoxicity test. All the target compounds were evaluated for their toxicity against human kidney epithelial cells 293T with the median cytotoxic concentration (CC50) data of tested compounds obtained by MTT assay. As displayed in Table 4, these compounds were tested at multiple doses to study the viability of macrophages. As shown in Table 4, the 18 compounds demonstrated almost no cytotoxic activities in vitro against human kidney epithelial cells 293T.
Table 4 The median cytotoxic concentration (CC50) data of all compounds
Compounds |
CC50a, μM |
Compounds |
CC50a, μM |
The cytotoxicity of each compound is expressed as the concentration of compound that reduced cell viability to 50% (CC50). |
4a |
46.25 |
4k |
43.28 |
4b |
52.76 |
4l |
53.44 |
4c |
49.24 |
4m |
49.21 |
4d |
50.03 |
4n |
52.36 |
4e |
46.36 |
4o |
63.23 |
4f |
53.28 |
4p |
43.28 |
4g |
52.78 |
4q |
55.64 |
4h |
46.24 |
4r |
57.38 |
4i |
61.42 |
Celecoxib |
53.46 |
4j |
57.62 |
|
|
2.4. Molecular docking
To gain better understanding of the potency of the 18 compounds, we examined the interactions of these compounds with EGFR by molecular docking, which was performed by simulation of the 18 compounds into the ATP binding site in EGFR. The protein structure of the EGFR was downloaded from the PDB (1M17.pdb)37 and was preprocessed using the Schrodinger Protein Preparation Guide, hydrogens were added to the structure, H-bonds within the protein were optimized, and the protein was minimized to an rmsd of 0.3 Å. A 9.9 Å sphere of water molecules was added around the ligand and a short (3 ps) dynamics run was carried out, followed by several cycles of minimization using Quanta/CHARMm. The entire protein–ligand–water complex was allowed to move during calculations.38 The predicted binding interaction energy was used as the criterion for ranking. The estimated interaction energy of other synthesized compounds ranged from −41.89 kcal mol−1 to −31.20 kcal mol−1. The selected compounds 4o and 4g had an estimated binding free energy of −41.89 kcal mol−1 and −40.44 kcal mol−1, respectively. The binding models of compounds 4o and 4g and EGFR are depicted in Fig. 3. The amino acid residues which had interaction with EGFR are labeled. In the binding mode, compound 4o was nicely bound to the ATP binding site of EGFR by hydrophobic interaction and binding was stabilized by a π–cation interaction (4o A: Lys 721). Besides, compound 4g was also nicely bound to the ATP binding site of EGFR by hydrophobic interaction and binding was stabilized by one hydrogen bond (angle Lys 721:HE1 4g:O = 94.45°, distance = 2.46 Å), and a π–cation interaction (4g A: Lys 721). This molecular docking results, along with the biological assay data, suggested that compounds 4o and 4g were potential inhibitors of EGFR.
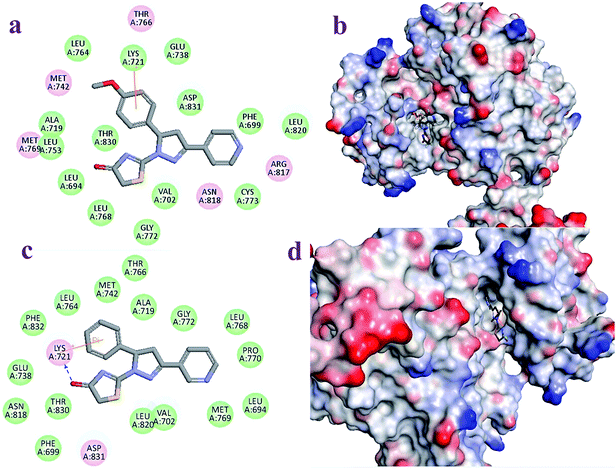 |
| Fig. 3 Docking of compounds 4o and 4g in the ATP binding site of EGFR. Left: 2D models of the interaction between compounds 4o and 4g and the ATP binding site. Right: 3D models of the interaction between compounds 4o and 4g and the ATP binding site, respectively. | |
2.5. 3D-QSAR model39
In order to acquire a systematic SAR profile on dihydropyridine-containing thiazolinone derivatives (4a–4r) as antitumor agents and to explore the more powerful and selective dual EGFR inhibitors, a 3D-QSAR model was built to choose activity conformation of the designed moleculars and reasonably evaluate the designed molecules by using the corresponding pIC50 values which were converted from the obtained IC50 (μM) values of EGFR inhibition and performed using the built-in QSAR software of DS 3.5 (Discovery Studio 3.5, Accelrys, Co. Ltd.). The way of this transformation was derived from an online calculator developed from an Indian medicinal chemistry lab (http://www.sanjeevslab.org/tools-IC50.html). The training and test set was divided by the random diverse molecules method of DS 3.5, in which the test set accounts for 22.22% while the training set was set to 77.78%. The training set composes 14 agents and 4 agents consisted the relative test set. The success of this model depended on the docking study and the reliability of the previous study into the activities data.
In the default situation, the alignment conformation of each molecule possessed the lowest CDOCKER_INTERACTION_ENERGY among the eighteen docked poses. The 3D-QSAR model generated from DS 3.5, defined the critical regions (steric or electrostatic) affecting the binding affinity. It was a PLS model set up with 400 independent variables (conventional R2 = 0.99). The graphical relationship of observed and predicted values is illustrated in Fig. 4a, in which the plot of the observed IC50 versus the predicted values showed that this model could be used in prediction of activity for dihydropyridine-containing thiazolinone derivatives (4a–4r).
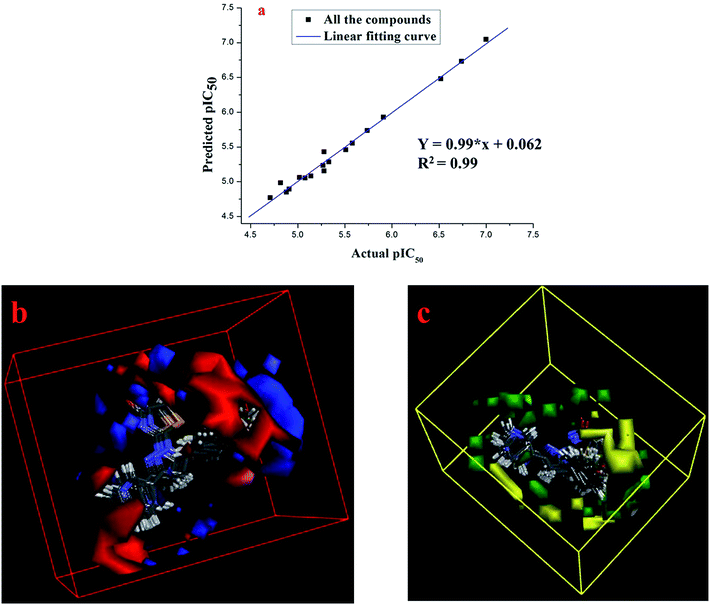 |
| Fig. 4 (a) Using a linear fitting curve to compare the predicted pIC50 values with those of experiment. (b) Isosurface of the 3D-QSAR model coefficients on electrostatic potential grids. The blue triangle mesh represents positive electrostatic potential and the red area represents negative electrostatic potential. (c) Isosurface of the 3D-QSAR model coefficients on van der Waals grids. The green triangle mesh representation indicates positive coefficients; the yellow triangle mesh indicates negative coefficients. | |
A contour plot of the electrostatic field region favorable (in blue) or unfavorable (red) for anticancer activity based on the EGFR protein target is shown in Fig. 4b, while energy grids corresponding to the favorable (in green) or unfavorable (yellow) steric effects for the EGFR affinity are shown in Fig. 4c. It was widely accepted that a better inhibitor based on the 3D-QSAR model should have strong van der Waals attraction in the green areas and a polar group in the blue electrostatic potential areas (which were dominant close to the skeleton). As shown in these two pictures, this promising model would provide a guideline to design and optimize more effective EGFR inhibitors based on the dihydropyridine-containing thiazolinone derivatives (4a–4r) and pave the way for further studies in the future.
3. Conclusions
In summary, we have designed and synthesized a novel series of dihydropyridine-containing thiazolinone derivatives (4a–4r) which were tested for their anticancer activities against B16–F10, HeLa and MCF-7 and for their EGFR/HER-2 inhibitory activities. Most of them exhibited potent anticancer and EGFR/HER-2 inhibitory activities, with IC50 values ranging from 0.099–19.62 μM and 3.26–20.33 μM respectively. These compounds showed a very interesting profile for the inhibition of EGFR. Most of them exhibited EGFR inhibitory activities and almost no toxicity towards 293T. Among them, compound 4o showed the most potent EGFR inhibition activities (IC50 = 0.099 μM for EGFR and IC50 = 3.26 μM for HER-2). Docking simulations were performed to obtain probable binding models and poses. After analysis of the binding models of compounds 4o and 4g with EGFR, it was found that a hydrogen bond and a π–cation interaction with the protein residues in the ATP binding site might play a crucial role in their EGFR inhibition and antiproliferative activities. Finally, QSAR models were built with previous activity data and binding conformations to begin our work in this paper as well as to provide a reliable tool for the reasonable design and synthesis of potent tyrosine kinase inhibitors.
4. Experiments
4.1. Materials and measurements
All chemicals and reagents used in the current study were analytical grade. Thin layer chromatography (TLC) was performed on silica gel plates with a fluorescent indicator. All analytical samples were homogeneous on TLC in at least two different solvent systems. Melting points (uncorrected) were determined on an X-4 MP apparatus (Taike Corp., Beijing, China). All the 1H NMR spectra were recorded on a Bruker DPX 300 model spectrometer in CDCl3 and chemical shifts (δ) were reported as parts per million (ppm). ESI-MS spectra were recorded on a Mariner System 5304 mass spectrometer.
4.2. Synthesis
4.2.1. General synthetic procedure of chalcones (2a–2r). Equimolar portions of the appropriate pyridinecarboxaldehyde (20 mmol) and substituted acetophenone (20 mmol) were dissolved in approximately 80 mL of ethanol. The mixture was allowed to stir for several minutes at 0 °C to dissolve. Subsequently added was a 10 mmol aliquot of 40% aqueous sodium hydroxide solution dropwise to the reaction flask via a self-equalizing addition funnel. The reaction solution was allowed to stir at 0 °C for approximately 2 h. Afterwards, the precipitate was collected by suction filtration, washed with cold ethanol (30 mL) three times and the purified chalcones (2a–2r) were acquired.
4.2.2. General synthetic procedure of pyrazole derivatives (3a–3r). A mixture of chalcone (2a–2r, 10 mmol), thiosemicarbazide (10 mmol), and KOH (10 mmol) was added in a 150 mL flask, then refluxed in ethanol (75 mL) for 12 h. After cooling, the solution was poured into ice-water and stirred for a few minutes. The precipitate formed was collected, washed three times with distilled water, and dried under vacuum. The crude products were purified by recrystallization with ethanol and washed with ice-water (25 mL) three times to give a pure product (3a–3r).
4.2.3. General synthetic procedure of dihydropyridine-containing thiazolinone derivatives (4a–4r). A mixture of compound 3a–3r (5 mmol), bromoacetic acid (5 mmol), acetic anhydride (5 mmol), and sodium acetate (5 mmol) was dissolved in ethanol (30 mL) and stirred at 80 °C for 6–8 h. After cooling, the solution was poured into ice-water and stirred for a few minutes. The precipitate formed was collected, washed three times with distilled water, and dried under vacuum. The crude products were purified by recrystallization with ethanol–methylene dichloride (1
:
1), and washed with ice-water (25 mL) three times to give a pure product (4a–4r).
4.2.3.1. 2-(5-Phenyl-3-(pyridin-2-yl)-4,5-dihydro-1H-pyrazol-1-yl)thiazol-4(5H)-one (4a). Green solid. Yield 63%, m.p. 189–191 °C; 1H NMR (CDCl3, 300 MHz); δ: 8.55 (d, J = 3.2 Hz, 2H, Ar); 7.81 (d, J = 6.5 Hz, 2H, Ar); 7.70 (t, J = 6.9 Hz, 1H, Ar); 7.48 (d, J = 7.1 Hz, 4H, Ar); 5.91 (q, J = 9.1 Hz, 1H, CH); 3.95 (q, J = 8.3 Hz, 1H, CH); 3.87 (s, 2H, CH2); 3.79 (q, J = 9.2 Hz, 1H, CH). ESI-MS: 323.3 (C17H14N4OS [M + H]+).
4.2.3.2. 2-(3-(Pyridin-2-yl)-5-(p-tolyl)-4,5-dihydro-1H-pyrazol-1-yl)thiazol-4(5H)-one (4b). Yellow solid. Yield 53%, m.p. 147–149 °C; 1H NMR (CDCl3, 300 MHz); δ: 8.56 (d, J = 5.3 Hz, 2H, Ar); 7.71 (d, J = 6.8 Hz, 2H, Ar); 7.47 (t, J = 2.0 Hz, 1H, Ar); 7.26 (d, J = 9.1 Hz, 3H, Ar); 5.91 (q, J = 6.2 Hz, 1H, CH); 3.87 (q, J = 8.2 Hz, 1H, CH); 3.74 (s, 2H, CH2); 3.61 (q, J = 5.1 Hz, 1H, CH); 2.42 (s, 3H, CH3). ESI-MS: 337.2 (C18H16N4OS [M + H]+).
4.2.3.3. 2-(5-(4-Methoxyphenyl)-3-(pyridin-2-yl)-4,5-dihydro-1H-pyrazol-1-yl)thiazol-4(5H)-one (4c). Yellow solid. Yield 62%, m.p. 154–156 °C; 1H NMR (CDCl3, 300 MHz); δ: 8.60 (d, J = 6.3 Hz, 2H, Ar); 7.78 (d, J = 9.9 Hz, 2H, Ar); 7.56 (t, J = 2.9 Hz, 1H, Ar); 6.92 (d, J = 5.1 Hz, 3H, Ar); 5.83 (q, J = 4.1 Hz, 1H, CH); 3.93 (q, J = 5.3 Hz, 1H, CH); 3.88 (d, J = 4.1 Hz, 3H, OCH3); 3.86 (s, 2H, CH2); 3.79 (q, J = 6.2 Hz, 1H, CH). ESI-MS: 353.1 (C18H16N4O2S [M + H]+).
4.2.3.4. 2-(5-(4-Fluorophenyl)-3-(pyridin-2-yl)-4,5-dihydro-1H-pyrazol-1-yl)thiazol-4(5H)-one (4d). Yellow solid. Yield 67%, m.p. 162–164 °C; 1H NMR (CDCl3, 300 MHz); δ: 8.67 (d, J = 4.5 Hz, 2H, Ar); 7.85 (d, J = 3.2 Hz, 2H, Ar); 7.69 (t, J = 7.9 Hz, 1H, Ar); 7.28 (d, J = 3.4 Hz, 3H, Ar); 5.89 (q, J = 4.7 Hz, 1H, CH); 3.93 (q, J = 4.8 Hz, 1H, CH); 3.88 (s, 2H, CH2); 3.80 (q, J = 5.1 Hz, 1H, CH). ESI-MS: 341.21 (C17H13FN4OS [M + H]+).
4.2.3.5. 2-(5-(4-Chlorophenyl)-3-(pyridin-2-yl)-4,5-dihydro-1H-pyrazol-1-yl)thiazol-4(5H)-one (4e). Yellow solid. Yield 57%, m.p. 142–144 °C; 1H NMR (CDCl3, 300 MHz); δ: 8.54 (d, J = 6.2 Hz, 2H, Ar); 7.78 (d, J = 7.3 Hz, 2H, Ar); 7.73 (t, J = 8.1 Hz, 1H, Ar); 7.36 (d, J = 6.4 Hz, 3H, Ar); 5.86 (q, J = 6.7 Hz, 1H, CH); 3.99 (q, J = 5.3 Hz, 1H, CH); 3.92 (s, 2H, CH2); 3.84 (q, J = 8.2 Hz, 1H, CH). ESI-MS: 358.0 (C17H13ClN4OS [M + H]+).
4.2.3.6. 2-(5-(4-Bromophenyl)-3-(pyridin-2-yl)-4,5-dihydro-1H-pyrazol-1-yl)thiazol-4(5H)-one (4f). Yellow solid. Yield 51%, m.p. 183–186 °C; 1H NMR (CDCl3, 300 MHz); δ: 8.48 (d, J = 3.3 Hz, 2H, Ar); 7.73 (d, J = 7.6 Hz, 2H, Ar); 7.68 (t, J = 4.3 Hz, 1H, Ar); 7.34 (d, J = 5.4 Hz, 3H, Ar); 5.88 (q, J = 5.1 Hz, 1H, CH); 3.89 (q, J = 9.8 Hz, 1H, CH); 3.68 (s, 2H, CH2); 3.48 (q, J = 5.1 Hz, 1H, CH). ESI-MS: 401.1 (C17H13BrN4OS [M + H]+).
4.2.3.7. 2-(5-Phenyl-3-(pyridin-3-yl)-4,5-dihydro-1H-pyrazol-1-yl)thiazol-4(5H)-one (4g). Yellow solid. Yield 59%, m.p. 134–135 °C; 1H NMR (CDCl3, 300 MHz); δ: 8.59 (d, J = 4.7 Hz, 2H, Ar); 7.92 (d, J = 5.4 Hz, 2H, Ar); 7.81 (t, J = 8.9 Hz, 1H, Ar); 7.51 (d, J = 5.1 Hz, 4H, Ar); 5.81 (q, J = 3.1 Hz, 1H, CH); 3.89 (q, J = 7.3 Hz, 1H, CH); 3.84 (s, 2H, CH2); 3.76 (q, J = 7.2 Hz, 1H, CH). ESI-MS: 323.3 (C17H14N4OS [M + H]+).
4.2.3.8. 2-(3-(Pyridin-3-yl)-5-p-tolyl-4,5-dihydro-1H-pyrazol-1-yl)thiazol-4(5H)-one (4h). Yellow solid. Yield 71%, m.p. 147–149 °C; 1H NMR (CDCl3, 300 MHz); δ: 8.57 (d, J = 2.6 Hz, 2H, Ar); 7.69 (d, J = 8.2 Hz, 2H, Ar); 7.59 (t, J = 2.0 Hz, 1H, Ar); 7.27 (d, J = 9.1 Hz, 3H, Ar); 5.82 (q, J = 4.1 Hz, 1H, CH); 3.99 (q, J = 11.3 Hz, 1H, CH); 3.89 (s, 2H, CH2); 3.41 (q, J = 4.2 Hz, 1H, CH); 2.44 (s, 3H, CH3). ESI-MS: 337.1 (C18H16N4OS [M + H]+).
4.2.3.9. 2-(5-(4-Methoxyphenyl)-3-(pyridin-3-yl)-4,5-dihydro-1H-pyrazol-1-yl)thiazol-4(5H)-one (4i). Yellow solid. Yield 54%, m.p. 143–144 °C; 1H NMR (CDCl3, 300 MHz); δ: 8.57 (d, J = 3.3 Hz, 2H, Ar); 7.81 (d, J = 7.9 Hz, 2H, Ar); 7.36 (t, J = 4.9 Hz, 1H, Ar); 6.72 (d, J = 8.1 Hz, 3H, Ar); 5.81 (q, J = 3.1 Hz, 1H, CH); 3.89 (q, J = 8.3 Hz, 1H, CH); 3.86 (d, J = 3.1 Hz, 3H, OCH3); 3.82 (s, 2H, CH2); 3.74 (q, J = 8.2 Hz, 1H, CH). ESI-MS: 353.3 (C18H16N4O2S [M + H]+).
4.2.3.10. 2-(5-(4-Fluorophenyl)-3-(pyridin-3-yl)-4,5-dihydro-1H-pyrazol-1-yl)thiazol-4(5H)-one (4j). Yellow solid. Yield 52%, m.p. 154–155 °C; 1H NMR (CDCl3, 300 MHz); δ: 8.69 (d, J = 6.1 Hz, 2H, Ar); 7.91 (d, J = 8.2 Hz, 2H, Ar); 7.72 (t, J = 6.3 Hz, 1H, Ar); 7.39 (d, J = 5.4 Hz, 3H, Ar); 5.85 (q, J = 6.2 Hz, 1H, CH); 3.98 (q, J = 5.7 Hz, 1H, CH); 3.87 (s, 2H, CH2); 3.81 (q, J = 7.1 Hz, 1H, CH). ESI-MS: 341.2 (C17H13FN4OS [M + H]+).
4.2.3.11. 2-(5-(4-Chlorophenyl)-3-(pyridin-3-yl)-4,5-dihydro-1H-pyrazol-1-yl)thiazol-4(5H)-one (4k). Yellow solid. Yield 73%, m.p. 187–189 °C; 1H NMR (CDCl3, 300 MHz); δ: 8.57 (d, J = 5.4 Hz, 2H, Ar); 7.74 (d, J = 8.5 Hz, 2H, Ar); 7.58 (d, J = 7.6 Hz, 2H, Ar); 7.48 (d, J = 8.4 Hz, 3H, Ar); 5.86 (q, J = 3.8 Hz, 1H, CH); 4.02 (q, J = 11.5 Hz, 1H, CH); 3.89 (s, 2H, CH2); 3.41 (q, J = 4.0 Hz, 1H, CH). ESI-MS: 357.9 (C17H13ClN4OS [M + H]+).
4.2.3.12. 2-(5-(4-Bromophenyl)-3-(pyridin-3-yl)-4,5-dihydro-1H-pyrazol-1-yl)thiazol-4(5H)-one (4l). Yellow solid. Yield 67%, m.p. 157–159 °C; 1H NMR (CDCl3, 300 MHz); δ: 8.56 (d, J = 5.0 Hz, 2H, Ar); 7.66 (d, J = 7.2 Hz, 2H, Ar); 7.57 (t, J = 11.0 Hz, 2H, Ar); 7.27 (d, J = 4.9 Hz, 2H, Ar); 5.86 (q, J = 3.9 Hz, 1H, CH); 3.99 (q, J = 11.4 Hz, 1H, CH); 3.88 (s, 2H, CH2); 3.42 (q, J = 4.2 Hz, 1H, CH). ESI-MS: 401.4 (C17H13BrN4OS [M + H]+).
4.2.3.13. 2-(5-Phenyl-3-(pyridin-4-yl)-4,5-dihydro-1H-pyrazol-1-yl)thiazol-4(5H)-one (4m). White solid. Yield 63%, m.p. 189–191 °C; 1H NMR (CDCl3, 300 MHz); δ: 8.55 (d, J = 3.2 Hz, 2H, Ar); 7.81 (d, J = 6.5 Hz, 2H, Ar); 7.70 (t, J = 6.9 Hz, 1H, Ar); 7.48 (d, J = 7.1 Hz, 4H, Ar); 5.91 (q, J = 9.1 Hz, 1H, CH); 3.95 (q, J = 8.3 Hz, 1H, CH); 3.87 (s, 2H, CH2); 3.79 (q, J = 9.2 Hz, 1H, CH). ESI-MS: 323.3 (C17H14N4OS [M + H]+).
4.2.3.14. 2-(3-(Pyridin-4-yl)-5-(p-tolyl)-4,5-dihydro-1H-pyrazol-1-yl)thiazol-4(5H)-one (4n). Yellow solid. Yield 66%, m.p. 176–178 °C; 1H NMR (CDCl3, 300 MHz); δ: 8.62 (d, J = 8.3 Hz, 2H, Ar); 7.73 (d, J = 6.8 Hz, 2H, Ar); 7.64 (t, J = 8.1 Hz, 1H, Ar); 7.47 (d, J = 5.1 Hz, 3H, Ar); 5.79 (q, J = 4.6 Hz, 1H, CH); 4.02 (q, J = 7.3 Hz, 1H, CH); 3.91 (s, 2H, CH2); 3.50 (q, J = 6.1 Hz, 1H, CH); 2.49 (s, 3H, CH3). ESI-MS: 337.2 (C18H16N4OS [M + H]+).
4.2.3.15. 2-(5-(4-Methoxyphenyl)-3-(pyridin-4-yl)-4,5-dihydro-1H-pyrazol-1-yl)thiazol-4(5H)-one (4o). Yellow solid. Yield 62%, m.p. 145–147 °C; 1H NMR (CDCl3, 300 MHz); δ: 8.59 (d, J = 3.9 Hz, 2H, Ar); 7.75 (d, J = 8.6 Hz, 2H, Ar); 7.29 (t, J = 3.8 Hz, 1H, Ar); 6.99 (d, J = 4.1 Hz, 3H, Ar); 5.79 (q, J = 3.1 Hz, 1H, CH); 3.99 (q, J = 11.3 Hz, 1H, CH); 3.87 (d, J = 5.1 Hz, 3H, OCH3); 3.85 (s, 2H, CH2); 3.79 (q, J = 4.2 Hz, 1H, CH). ESI-MS: 353.2 (C18H16N4O2S [M + H]+).
4.2.3.16. 2-(5-(4-Fluorophenyl)-3-(pyridin-4-yl)-4,5-dihydro-1H-pyrazol-1-yl)thiazol-4(5H)-one (4p). Yellow solid. Yield 47%, m.p. 172–174 °C; 1H NMR (CDCl3, 300 MHz); δ: 8.64 (d, J = 5.4 Hz, 2H, Ar); 7.87 (d, J = 5.1 Hz, 2H, Ar); 7.79 (t, J = 6.2 Hz, 1H, Ar); 7.34 (d, J = 6.2 Hz, 3H, Ar); 5.81 (q, J = 7.8 Hz, 1H, CH); 3.98 (q, J = 5.2 Hz, 1H, CH); 3.86 (s, 2H, CH2); 3.77 (q, J = 3.4 Hz, 1H, CH). ESI-MS: 341.4 (C17H13FN4OS [M + H]+).
4.2.3.17. 2-(5-(4-Chlorophenyl)-3-(pyridin-4-yl)-4,5-dihydro-1H-pyrazol-1-yl)thiazol-4(5H)-one (4q). Yellow solid. Yield 56%, m.p. 165–166 °C; 1H NMR (CDCl3, 300 MHz); δ: 8.63 (d, J = 6.1 Hz, 2H, Ar); 7.81 (d, J = 7.5 Hz, 2H, Ar); 7.63 (d, J = 6.7 Hz, 2H, Ar); 7.51 (d, J = 10.4 Hz, 3H, Ar); 5.83 (q, J = 4.1 Hz, 1H, CH); 4.04 (q, J = 9.3 Hz, 1H, CH); 3.92 (s, 2H, CH2); 3.35 (q, J = 4.3 Hz, 1H, CH). ESI-MS: 357.7 (C17H13ClN4OS [M + H]+).
4.2.3.18. 2-(5-(4-Bromophenyl)-3-(pyridin-4-yl)-4,5-dihydro-1H-pyrazol-1-yl)thiazol-4(5H)-one (4r). Red solid. Yield 55%, m.p. 166–168 °C; 1H NMR (CDCl3, 300 MHz); δ: 8.51 (d, J = 5.3 Hz, 2H, Ar); 7.64 (d, J = 6.9 Hz, 2H, Ar); 7.53 (t, J = 9.1 Hz, 2H, Ar); 7.41 (d, J = 8.4 Hz, 2H, Ar); 5.81 (q, J = 6.1 Hz, 1H, CH); 3.94 (q, J = 3.7 Hz, 1H, CH); 3.89 (s, 2H, CH2); 3.49 (q, J = 7.6 Hz, 1H, CH). ESI-MS: 401.4 (C17H13BrN4OS [M + H]+).
4.3. Preparation, purification of HER-2 and EGFR and inhibitory assay
A 1.7 kb cDNA encoded for human HER-2 cytoplasmic domain (HER-2-CD, amino acids 676–1245) and 1.6 kb cDNA encoded for the EGFR cytoplasmic domain (EGFR-CD, amino acids 645–1186) were cloned into baculoviral expression vectors pBlueBacHis2B and pFASTBacHTc (Huakang Company, China), separately. A sequence that encodes (His)6 was located at the 5′ upstream to the HER-2 and EGFR sequences. Sf-9 cells were infected for 3 days for protein expression. Sf-9 cell pellets were solubilized at 0 °C in a buffer at pH 7.4 containing 50 mM HEPES, 10 mM NaCl, 1% Triton, 10 μM ammonium molybdate, 100 μM sodium vanadate, 10 μg mL−1 aprotinin, 10 μg mL−1 leupeptin, 10 μg mL−1 pepstatin, and 16 μg mL−1 benzamidine HCl for 20 min followed by 20 min centrifugation. Crude extract supernatant was passed through an equilibrated Ni-NTA superflow packed column and washed with 10 mM and then 100 mM imidazole to remove non-specifically bound material. Histidine tagged proteins were eluted with 250 and 500 mM imidazole and dialyzed against 50 mM NaCl, 20 mM HEPES, 10% glycerol, and 1 μg mL−1 each of aprotinin, leupeptin, and pepstatin for 2 h. The entire purification procedure was performed at 4 °C or on ice (Tsou, Mamuya et al. 2001).
Both EGFR and HER-2 kinase assays were set up to assess the level of autophosphorylation based on DELFIA/time-resolved fluorometry. Compounds 4a–4r were dissolved in 100% DMSO and diluted to the appropriate concentrations with 25 mM HEPES at pH 7.4. In each well, 10 μL compound was incubated with 10 μL (12.5 ng for HER-2 or 5 ng for EGFR) recombinant enzyme (1
:
80 dilution in 100 mM HEPES) for 10 min at room temperature. Then, 10 μL of 5 mM buffer (containing 20 mM HEPES, 2 mM MnCl2, 100 μM Na3VO4, and 1 mM DTT) and 20 μL of 0.1 mM ATP–50 mM MgCl2 were added for 1 h. Positive and negative controls were included in each plate by incubation of enzyme with or without ATP–MgCl2. At the end of incubation, liquid was aspirated, and plates were washed three times with wash buffer. A 75 μL (400 ng) sample of europium labeled anti-phosphotyrosine antibody was added to each well for another 1 h of incubation. After washing, enhancement solution was added and the signal was detected using a Victor (Wallac Inc.) with excitation at 340 nm and emission at 615 nm. The percentage of auto-phosphorylation inhibition by the compounds was calculated using the following formula: 100% − [(negative control)/(positive control − negative control)]. The IC50 values were obtained from curves of percentage inhibition with eight concentrations of compound. As the contaminants in the enzyme preparation are fairly low, the majority of the signal detected by the anti-phosphotyrosine antibody is from EGFR or HER-2.
4.4. Antiproliferative activity
The antiproliferative activities of the prepared compounds were evaluated using a standard (MTT)-based colorimetric assay with some modification. Cell lines were grown to log phase in DMEM supplemented with 10% fetal bovine serum, under a humidified atmosphere of 5% CO2 at 37 °C. Cell suspensions were prepared and 100 μL per well dispensed into 96 well plates giving 105 cells per well. The plates were returned to the incubator for 24 h to allow the cells to reattach. Subsequently, cells were treated with the target compounds at increasing concentrations in the presence of 10% FBS for 48 h. Then, cell viability was assessed by the conventional 3-(4,5-dimethylthiazol-2-yl)-2,5-diphenyltetrazolium bromide (MTT) reduction assay, carried out strictly according to the manufacturer instructions (Sigma). The absorbance (OD570) was read on an ELISA reader (Tecan, Austria).
4.5. Molecular docking study
Docking of compounds into the 3D EGFR complex structure (PDB code: 1M17) was carried out using the Discovery Studio (version 3.5) as implemented through the graphical user interface DS-LigandFit protocol. The three-dimensional structures of the aforementioned compounds were constructed using Chem3D ultra 11.0 software [Chemical Structure Drawing Standard; Cambridge Soft corporation, USA (2009)], then they were energetically minimized by using MOPAC with 100 iterations and a minimum RMS gradient of 0.10. The crystal structures of the EGFR complex were retrieved from the RCSB Protein Data Bank (http://www.rcsb.org/pdb/home/home.do). All bound water and ligands were eliminated from the protein and polar hydrogen was added. The whole EGFR complex was defined as a receptor and the site sphere was selected based on the ligand binding location of ATP, then the ATP molecule was removed and 4o and 4g were placed during the molecular docking procedure. Types of interactions of the docked protein with ligands were analyzed after the end of molecular docking.
4.6. 3D-QSAR
The ligand-based 3D-QSAR approach was performed using QSAR software of DS 3.5 (Discovery Studio 3.5, Accelrys, Co. Ltd.). The training sets were composed of inhibitors with the corresponding pIC50 values which were converted from the obtained IC50 (μM), and test sets comprised compounds of data sets as listed in Table 4.
In Discovery Studio, the CHARMm force field is used and the electrostatic potential and the van der Waals potential are treated as separate terms. A +1e point charge is used as the electrostatic potential probe and a distance-dependent dielectric constant is used to mimic the solvation effect. For the van der Waals potential a carbon atom with a 1.73 Å radius is used as a probe.
All the definitions of the descriptors can be seen in the “Help” section of the DS 3.5 software and they were calculated by the QSAR protocol of DS 3.5. The alignment conformation of each molecule was the one with the lowest interaction energy in the docked results of CDOCKER. The predictive ability of 3D-QSAR modeling can be evaluated based on the cross-validated correlation coefficient, which qualifies the predictive ability of the models. Scrambled test (Y scrambling) was performed to investigate the risk of chance correlations. The inhibitory potencies of compounds were randomly reordered 30 times and subject to leave-one-out validation tests, respectively. The models were also validated by test sets, in which the compounds are not included in the training sets, respectively. Usually, one can believe that the modeling is reliable, when the R2 for test sets is larger than 0.6.
Acknowledgements
The work was financed by a grant (no. J1103512) from the National Natural Science Foundation of China.
References and notes
- L. Seymour, Cancer Treat. Rev., 1999, 25, 301–312 CrossRef CAS PubMed.
- S. Kamath and J. K. Buolamwini, J. Med. Chem., 2003, 46, 4657–4668 CrossRef CAS PubMed.
- A. A. Adjei, J. Natl. Cancer Inst., 2001, 93, 1062–1074 CrossRef CAS PubMed.
- B. J. Druker, Trends Mol. Med., 2002, 8, S14–S18 CrossRef CAS.
- X. Zhang, J. Gureasko, K. Shen, P. A. Cole and J. Kuriyan, Cell, 2006, 125, 1137–1149 CrossRef CAS PubMed.
- S. Xu, T. Xu, L. Zhang, Z. Zhang, J. Luo, Y. Liu, X. Lu, Z. Tu, X. Ren and K. Ding, J. Med. Chem., 2013, 56, 8803–8813 CrossRef CAS PubMed.
- P. Rani, V. K. Srivastava and A. Kumar, Eur. J. Med. Chem., 2004, 39, 449–452 CrossRef CAS PubMed.
- K. S. Kolibaba and B. J. Druker, Biochim. Biophys. Acta, Rev. Cancer, 1997, 1333, F217–F248 CrossRef CAS.
- Y. Luo, Y. Li, K. Qiu, X. Lu, J. Fu and H. Zhu, Bioorg. Med. Chem., 2011, 19, 6069–6076 CrossRef CAS PubMed.
- S. S. Sridhar, L. Seymour and F. A. Shepherd, Lancet Oncol., 2003, 4, 397–406 CrossRef CAS.
- A. J. Bridges, Curr. Med. Chem., 1999, 6, 825–844 CAS.
- P. Blume-Jensen and T. Hunter, Nature, 2001, 411, 355–365 CrossRef CAS PubMed.
- F. Ciardiello and G. Tortora, Clin. Cancer Res., 2001, 7, 2958–2970 CAS.
- D. H. Boschelli, Drugs Future, 1999, 24, 515–538 CrossRef CAS.
- V. Chandregowda, G. Venkateswara Rao and G. Chandrasekara Reddy, Org. Process Res. Dev., 2007, 11, 813–816 CrossRef CAS.
- S. Manfredini, R. Bazzanini, P. G. Baraldi, M. Guarneri, D. Simoni, M. E. Marongiu, A. Pani, P. La Colla and E. Tramontano, J. Med. Chem., 1992, 35, 917–924 CrossRef CAS.
- B. F. Abdel-Wahab, H. A. Abdel-Aziz and E. M. Ahmed, Eur. J. Med. Chem., 2009, 44, 2632–2635 CrossRef CAS PubMed.
- J. S. Solanki, T. R. Thapak, A. Bhardwaj and U. N. Tripathi, J. Coord. Chem., 2011, 64, 369–376 CrossRef CAS.
- S. Manfredini, R. Bazzanini, P. G. Baraldi, M. Bonora, M. Marangoni, D. Simoni, A. Pani, F. Scintu, E. Pinna and L. Pisano, Anti-Cancer Drug Des., 1996, 11, 193–204 CAS.
- H. Park, K. Lee, S. Park, B. Ahn, J. Lee, H. Cho and K. Lee, Bioorg. Med. Chem. Lett., 2005, 15, 3307–3312 CrossRef CAS PubMed.
- A. Tanitame, Y. Oyamada, K. Ofuji, M. Fujimoto, N. Iwai, Y. Hiyama, K. Suzuki, H. Ito, H. Terauchi and M. Kawasaki, J. Med. Chem., 2004, 47, 3693–3696 CrossRef CAS PubMed.
- Ş. Güniz Küçükgüzel, S. Rollas, H. Erdeniz, M. Kiraz, A. Cevdet Ekinci and A. Vidin, Eur. J. Med. Chem., 2000, 35, 761–771 CrossRef.
- T. D. Penning, J. J. Talley, S. R. Bertenshaw, J. S. Carter, P. W. Collins, S. Docter, M. J. Graneto, L. F. Lee, J. W. Malecha and J. M. Miyashiro, J. Med. Chem., 1997, 40, 1347–1365 CrossRef CAS PubMed.
- R. Sridhar, P. T. Perumal, S. Etti, G. Shanmugam, M. N. Ponnuswamy, V. R. Prabavathy and N. Mathivanan, Bioorg. Med. Chem. Lett., 2004, 14, 6035–6040 CrossRef CAS PubMed.
- G. R. Bebernitz, G. Argentieri, B. Battle, C. Brennan, B. Balkan, B. F. Burkey, M. Eckhardt, J. Gao, P. Kapa and R. J. Strohschein, J. Med. Chem., 2001, 44, 2601–2611 CrossRef CAS PubMed.
- P. Lv, H. Li, J. Sun, Y. Zhou and H. Zhu, Bioorg. Med. Chem., 2010, 18, 4606–4614 CrossRef CAS PubMed.
- K. Qiu, H. Wang, L. Wang, Y. Luo, X. Yang, X. Wang and H. Zhu, Bioorg. Med. Chem., 2012, 20, 2010–2018 CrossRef CAS PubMed.
- L. T. Webster, A. Gilman, T. W. Rall, A. S. Nies and P. Taylor, in The Pharmacological Basis of Therapeutics, Pergamon Press, New York, 1990, vol. 8, pp. 999–1007 Search PubMed.
- D. S. Kundariya, P. K. Patel and B. M. Bheshdadia, Orient. J. Chem., 2014, 5, 138–143 Search PubMed.
- A. A. Geronikaki, E. P. Pitta and K. S. Liaras, Curr. Med. Chem., 2013, 20, 4460–4480 CrossRef CAS.
- Y. Li, J. Geng, Y. Liu, S. Yu and G. Zhao, ChemMedChem, 2013, 8, 27–41 CrossRef CAS PubMed.
- E. Rydzik, A. Szadowska and A. Kamińska, Acta. Pol. Pharm., 1984, 41, 459–464 CAS.
- D. Havrylyuk, B. Zimenkovsky, O. Karpenko, P. Grellier and R. Lesyk, Synthesis of pyrazoline–thiazolidinone hybrids with trypanocidal activity, Eur. J. Med. Chem., 2014, 85, 245–254 CrossRef CAS PubMed.
- R. Dayam, F. Aiello, J. Deng, Y. Wu, A. Garofalo, X. Chen and N. Neamati, J. Med. Chem., 2006, 49, 4526–4534 CrossRef CAS PubMed.
- S. Manfredini, R. Bazzanini, P. G. Baraldi, M. Guarneri, D. Simoni, M. E. Marongiu, A. Pani, P. La Colla and E. Tramontano, J. Med. Chem., 1992, 35, 917–924 CrossRef CAS.
- S. Manfredini, R. Bazzanini, P. G. Baraldi, M. Bonora, M. Marangoni, D. Simoni, A. Pani, F. Scintu, E. Pinna and L. Pisano, Anti-Cancer Drug Des., 1996, 11, 193–204 CAS.
- J. Stamos, M. X. Sliwkowski and C. Eigenbrot, J. Biol. Chem., 2002, 277, 46265–46272 CrossRef CAS PubMed.
- L. T. Webster, Drugs Used in the Chemotherapy of Protozoal Infections, in The Pharmacological Basis of Therapeutics, ed. A. Gilman, T. W. Rall, A. S. Nies and P. Taylor, Pergamon Press, New York, 8th edn, 1990, pp. 999–1007 Search PubMed.
- W. J. Egan, K. M. Merz and J. J. Baldwin, J. Med. Chem., 2000, 43, 3867–3877 CrossRef CAS PubMed.
Footnotes |
† Electronic supplementary information (ESI) available. CCDC 1030396. For ESI and crystallographic data in CIF or other electronic format see DOI: 10.1039/c4ra10606g |
‡ These two authors contributed equally to this paper. |
|
This journal is © The Royal Society of Chemistry 2015 |