DOI:
10.1039/C4FO00695J
(Paper)
Food Funct., 2015,
6, 218-228
Quercetin 7-O-glucoside suppresses nitrite-induced formation of dinitrosocatechins and their quinones in catechin/nitrite systems under stomach simulating conditions
Received
4th August 2014
, Accepted 17th October 2014
First published on 17th October 2014
Abstract
Foods of plant origin contain flavonoids. In the adzuki bean, (+)-catechin, quercetin 3-O-rutinoside (rutin), and quercetin 7-O-β-D-glucopyranoside (Q7G) are the major flavonoids. During mastication of foods prepared from the adzuki bean, the flavonoids are mixed with saliva and swallowed into the stomach. Here we investigated the interactions between Q7G and (+)-catechin at pH 2, which may proceed in the stomach after the ingestion of foods prepared from the adzuki bean. Q7G reacted with nitrous acid producing nitric oxide (˙NO) and a glucoside of 2-(3,4-dihydroxybenzoyl)-2,4,6-trihydroxy-3(2H)-benzofuranone. (+)-Catechin reacted with nitrous acid producing ˙NO and 6,8-dinitrosocatechin. The production of the dinitrosocatechin was partly suppressed by Q7G, and the suppression resulted in the enhancement of Q7G oxidation. 6,8-Dinitrosocatechin reacted further with nitrous acid generating the o-quinone, and the quinone formation was effectively suppressed by Q7G. In the flavonoids investigated, the suppressive effect decreased in the order Q7G ≈ quercetin > kaempferol > quercetin 4′-O-glucoside > rutin. Essentially the same results were obtained when (−)-epicatechin was used instead of (+)-catechin. The results indicate that nitrous acid-induced formation of 6,8-dinitrosocatechins and the o-quinones can be suppressed by flavonols in the stomach, and that both a hydroxyl group at C3 and ortho-hydroxyl groups in the B-ring are required for efficient suppression.
Introduction
Quercetin glycosides and catechins are the common flavonoids found in plants such as the adzuki bean, apple, buckwheat, cacao, onion, and tea.1–5 During the ingestion of foods or beverages prepared from such plants, flavonoids are mixed with saliva in the oral cavity, and then swallowed into the stomach, where the pH is around 2. Under such conditions, nitrite in saliva, which is produced from salivary nitrate by nitrate-reducing bacteria in the oral cavity,6 is transformed into nitrous acid (pKa = 3.3). The concentration of nitrite in mixed whole saliva ranges from 0.05 to 1 mM.7 Nitrous acid (E° = 0.983 V; 0.865 V at pH 2.0, calculated value) can generate reactive nitrogen species such as NO+, ˙NO2 and N2O3 by self-decomposition, and can react with (+)-catechin (E°′ = 0.49 V, pH 2.0) (1a and 1b), quercetin (E°′ = 0.45 V, pH 2.0) (3)8,9 (Fig. 1) and other polyphenols producing nitric oxide (˙NO).10–13 The major reaction products of catechins and quercetin have been reported to be 6,8-dinitrosocatechins (2a and 2b)14 and 2-(3,4-dihydroxybenzoyl)-2,4,6-trihydroxy-3(2H)-benzofuranone (5),15,16 respectively. The differences in the oxidation products are supposed to be due to the differences in the reactivity of ˙NO with semiquinone radicals of (+)-catechin and quercetin generated in (+)-catechin/nitrous acid and quercetin/nitrous acid systems, respectively.17 The functions of ˙NO produced by the above reactions in the stomach are reviewed;18 they include antimicrobial activity,19,20 inhibition of stress-induced gastric mucosal injury,21 increase in gastric blood flow, mucus formation,22–24 and inhibition of lipid peroxidation by scavenging the peroxyl and alkoxyl radicals of unsaturated fatty acids.25 On the other hand, nitrous acid can produce carcinogenic nitrosoamines through the N-nitrosation of secondary amines or amides.26–29 In flavonoids, catechins are effective inhibitors of the N-nitrosoamine formation.14,30–32
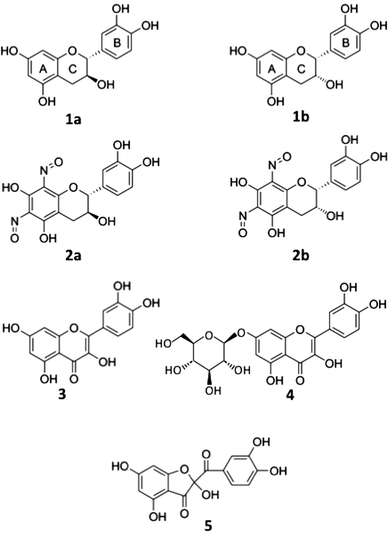 |
| Fig. 1 Compounds concerned in this study. 1a, (+)-catechin; 1b, (−)-epicatechin; 2a, 6,8-dinitrosocatechin; 2b, 6,8-dinitrosoepicatechin; 3, quercetin; 4, quercetin 7-O-β-D-glucopyranoside; 5, 2-(3,4-dihydroxybenzoyl)-2,4,6-trihydroxy-3(2H)-benzofuranone. | |
Recently, it has been reported that (+)-catechin in the methanol extract of the adzuki bean and (−)-epicatechin in the methanol extract of apple are transformed into 2a and 2b, respectively, after their incubation in mixed whole saliva under acidic conditions,33,34 and that 2a and 2b are oxidized by nitrous acid.17,33 The oxidation products are postulated to be o-quinones of 2a and 2b from the result that ascorbic acid reduces the oxidation products to 2a and 2b and that thiocyanate reacts with the oxidation products producing 6′-thiocyanate-6,8-dinitrosocatechin and 6′-thiocyanate-6,8-dinitrosoepicatechin, respectively.33 In addition, quercetin effectively suppresses the oxidation of 2a and 2b.17 Quercetin 7-O-glucoside (Q7G) (4) is a major flavonoid in the adzuki bean in addition to (+)-catechin,4,5 and Q7G can be postulated to be as reactive as quercetin because of the presence of a free hydroxyl group at C3 and ortho-hydroxyl groups in the B ring. The presence of both Q7G and (+)-catechin in the adzuki bean prompted us to investigate the effects of Q7G on the nitrous acid-induced formation of 2a and 2b from catechins and on the formation of o-quinones from 2a and 2b.
The main aim of this study is to explore the possible interactions of Q7G with catechins and 2a and 2b during their oxidation by nitrous acid under stomach simulating conditions. In addition to the above interactions, the interactions of (+)-catechin and 2a and 2b with quercetin 3-rutinoside (rutin), quercetin 4′-glucoside (Q4′G), and kaempferol were also studied in the presence of nitrous acid. Taking the results obtained in this study into account, the importance of interactions of flavonols with catechins and 2a and 2b during their reactions with nitrous acid in the stomach is discussed from the point of prevention of formation of 2a and 2b and the quinones.
Results and discussion
Reaction of Q7G with nitrous acid
Fig. 2A shows nitrite-induced changes in absorption spectra of Q7G in 50 mM KCl–HCl (pH 2.0). After the addition of sodium nitrite, absorption peaks of Q7G at 254 and 366 nm decreased increasing the absorbance at 288 nm. Such spectral changes have been reported during nitrous acid-induced oxidation of quercetin, and the oxidation product (Qox) is determined to be 5.15
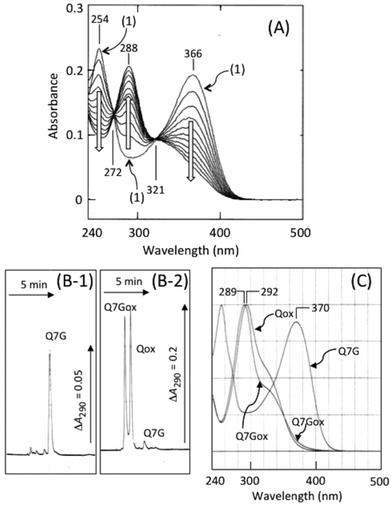 |
| Fig. 2 Nitrous acid-induced oxidation of Q7G. (A) Nitrite-induced changes in absorption spectra. The reaction mixture (1 mL) contained 25 μM Q7G in 50 mM KCl–HCl (pH 2.0). Sodium nitrite (0.2 mM) was added to the spectrum 1, and scanning was repeated 10 times at 1 min intervals. Light path of the measuring beam was 4 mm. (B) HPLC of the oxidation product of Q7G (Q7Gox). B-1, before incubation of 50 μM Q7G; B-2, after incubation of 50 μM Q7G with 0.25 mM NaNO2 for 2 min in 50 mM KCl–HCl (pH 2.0). An oxidation product of quercetin (Qox) was co-chromatographed for comparison. Mobile phase, methanol and 0.2% formic acid (1 : 1, v/v). (C) Absorption spectra of Q7G, Q7Gox, and Qox in the mobile phase. | |
The reaction product of Q7G (Q7Gox) was separated as a single peak by HPLC at the retention time of 2.9 min (Fig. 2B). In Fig. 2B, Qox (retention time, 3.4 min) was co-chromatographed. The UV/visible absorption spectrum of the product had a peak at 289 nm with a shoulder around 320 nm, and the absorption spectrum was similar to Qox (Fig. 2C). To understand the relationship between Q7Gox and Qox, Q7Gox was incubated with β-glucosidase. During the incubation of Q7Gox for 40 min, its concentration decreased irrespective of the presence or absence of β-glucosidase in a similar way with a half time of approximately 20 min, but the decrease in the presence of the glucosidase accompanied the formation of a compound that was identified to be Qox from the retention time and UV/visible absorption spectrum. Therefore, we estimated that Q7Gox was a glucoside of 5.
Fig. 3 shows the effects of nitrite concentration on the consumption of Q7G and quercetin and the formation of Q7Gox and Qox. The concentrations of both quercetin (○) and Q7G (●) decreased by nitrite without significant differences. The result suggests that Q7G was oxidized by nitrous acid as effectively as quercetin. During the decrease in the concentrations of Q7G and quercetin, their oxidation products, Q7Gox (■) and Qox (□), were produced. Their production roughly correlated with the consumption of their mother compounds.
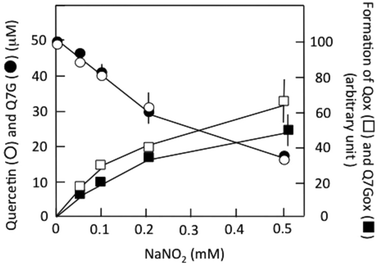 |
| Fig. 3 Nitrite-induced oxidation of Q7G and quercetin. The reaction mixture (1 mL) contained 50 μM Q7G or quercetin in 50 mM KCl–HCl (pH 2.0). The reactions were initiated by adding various concentrations of NaNO2 under anaerobic conditions. After 1 min of incubation, the concentrations of the reactants and products were determined by HPLC as described in Experimental section. (○), quercetin remained; (●), Q7G remained; (□), Qox formed; (■), Q7Gox formed. Formation of Qox and Q7Gox was estimated from the peak area of HPLC at 290 nm. Each data point represents mean with SD (n = 3–4). | |
Rate constants of the consumption of Q7G and quercetin were estimated under anaerobic conditions by postulating the following reaction:
| Quercetin/Q7G + HNO2 → Quercetin/Q7G radical + ˙NO + H2O | (1) |
The values were (12.5 ± 1.2) × 102 M−1 min−1 (n = 4) for Q7G and (13.5 ± 1.1) × 102 M−1 min−1 (n = 4) for quercetin. The oxidation of Q7G and quercetin by nitrous acid was faster under aerobic than anaerobic conditions, suggesting the contribution of ˙NO2 produced by the autoxidation of ˙NO to the oxidation of Q7G under aerobic conditions. It has been reported that the suppressive effect of Q7G is about 50% of that of quercetin for the lipid peroxidation induced by 2,2′-azobis(2-amidopropyl) dihydrochloride in large egg yolk phosphatidylcholine unilamellar vesicles.35 The smaller effect can be attributed to the difference in solubility of the lipid bilayer between Q7G and quercetin.
Characterization of reaction products of (+)-catechin
Fig. 4(I and II) show the HPLC of (+)-catechin and products generated in (+)-catechin/nitrous acid systems, and Fig. 4(III) shows their absorption spectra. Accompanying the reaction of (+)-catechin with nitrite under acidic conditions for 10 min, 2a was formed (trace I-2). The retention time (7.5 min) and the absorption spectrum (the peak at 274 nm with a shoulder around 320 nm) were identical with those of 6,8-dinitrosocatechin, which had been prepared in this study (see the Experimental section). As the mechanism of 2a formation, the following reactions have been proposed.17,33 | (+)-Catechin + HNO2 → (+)-Catechin radical + ˙NO + H2O | (2) |
| (+)-Catechin radical + ˙NO → Mononitrosocatechin | (3) |
| Mononitrosocatechin + HNO2 → Mononitrosocatechin radical + ˙NO + H2O | (4) |
| Mononitrosocatechin radical + ˙NO → 2a | (5) |
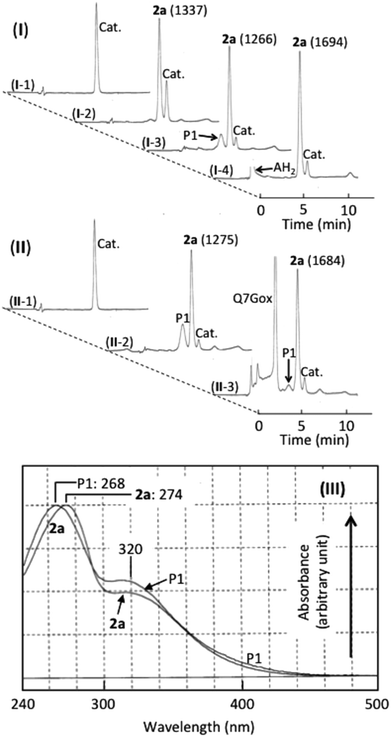 |
| Fig. 4 Characterization of reaction products of (+)-catechin. (I) HPLC. The reaction mixture contained 0.1 mM (+)-catechin and 0.2 mM NaNO2 in 50 mM KCl–HCl (pH 2.0). (I-1) Before addition of nitrite; (I-2) 10 min after addition of nitrite; (I-3) 25 min after the addition of nitrite; (I-4) 15 min after addition of 1 mM ascorbic acid to (I-3). (II) HPLC with Q7G. The reaction mixture contained 0.1 mM (+)-catechin and 0.5 mM NaNO2 in 50 mM KCl–HCl (pH 2.0). (II-1) Before addition of nitrite; (II-2) 10 min after addition of nitrite; (II-3) 10 min after addition of nitrite in the presence of 0.1 mM Q7G. HPLC was performed using a Shim-pack CLC-ODS and the mobile phase was a mixture of methanol and 0.2% formic acid (1 : 3, v/v). Numbers in parentheses, peak areas of 2a. (III) Absorption spectra of 2a and P1 in the mobile phase. AH2, ascorbic acid; Cat., (+)-catechin. | |
During further incubation of the reaction mixture, the concentration of (+)-catechin decreased without increasing the concentration of 2a, and a new component (P1) was produced (trace I-3). From the retention time (7.0 min) and the absorption spectrum (the peak at 269 nm with a shoulder around 320 nm) and from the ascorbic acid-induced disappearance of P1 increasing the concentration of 2a by 33% (trace I-4), P1 was postulated to be a quinone of 2a. The postulation is supported by not only its reduction to 2a by ascorbic acid but also its transformation into 6′-thiocyanate-6,8-dinitrosocatechin by thiocyanate at pH 2.33o-Quinones of caffeic acid, chlorogenic acid, and rutin also react with thiocyanate at pH 2 producing the thiocyanate conjugates, which are hydrolyzed to oxathiolone derivatives and NH3.36,37 The following reactions may be possible for the formation of o-quinone from 2a.
| 2a + HNO2 → 2a radical + ˙NO + H2O | (6) |
| 2 × (2a radical) → 2a + 2a quinone | (7) |
Effects of Q7G on the formation of 2a and P1 were studied. Under the experimental conditions of trace II-2, both P1 and 2a were produced in a (+)-catechin/nitrous acid system during the incubation for 10 min. The addition of Q7G to the above system and the incubation for 10 min resulted in the formation of Q7Gox that was estimated from the retention time and the absorption spectrum. The formation of Q7Gox accompanied the inhibition of P1 formation by about 80% and enhancement of 2a production by about 30%, suppressing slightly the decrease in the concentration of (+)-catechin (trace II-3). The result suggests that Q7G might have interacted with (+)-catechin and 2a during their reactions with nitrous acid. Therefore, we studied the interactions of Q7G with (+)-catechin and 2a under stomach simulating conditions.
Interactions of Q7G with catechins
Fig. 5A shows the interaction of Q7G with (+)-catechin during their reaction with nitrous acid. The formation of 2a was enhanced by about 20% by 5 μM Q7G, and suppressed by about 20% by 50 μM Q7G (■). The enhancement may be explained by the increase in ˙NO production by Q7G/nitrous acid systems (see below). The consumption of (+)-catechin was suppressed by about 35% by 5 and 15 μM Q7G and by about 65% by 50 μM Q7G (□). According to reactions (2)–(5), we can deduce that the suppression of (+)-catechin consumption may be due to Q7G-dependent reduction of the semiquinone radical of (+)-catechin, and that the suppression of 2a formation may be due to the reduction of semiquinone radicals of both (+)-catechin and mononitrosocatechin. Nitrous acid-induced formation of the semiquinone radical of (+)-catechin has been reported.11
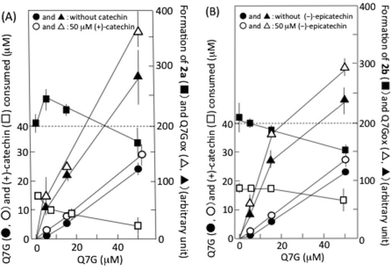 |
| Fig. 5 Interactions of Q7G with (+)-catechin (A) and (−)-epicatechin (B). The reaction mixture contained various concentrations of Q7G with and without 50 μM (+)-catechin or (−)-epicatechin in 50 mM KCl–HCl (pH 2.0). One min after the addition of 0.5 mM NaNO2 under anaerobic conditions, the reaction mixture was analyzed by HPLC. Mobile phases were mixtures of methanol and 0.2% formic acid. (A) 1 : 4 (v/v); (B) 1 : 3 (v/v). Each data point represents mean with SD (n = 3–4). (● and ○) consumption of Q7G in the absence and presence of catechins, respectively; (▲ and △) formation of Q7Gox in the absence and presence of catechins, respectively; (■) formation of 2a and 2b in the presence of (+)-catechin and (−)-epicatechin, respectively; (□) consumption of (+)-catechin or (−)-epicatechin. | |
Fig. 5A also shows the effects of (+)-catechin on the nitrous acid-induced consumption of Q7G and the formation of Q7Gox. The consumption of Q7G during 1 min incubation increased nearly linearly with the increase in Q7G concentration (●), and 50 μM (+)-catechin enhanced the consumption of 5 μM Q7G by about 40% and the consumption of 15 and 50 μM Q7G by about 20% (○). The enhancement of Q7G consumption by (+)-catechin accompanied the enhancement of Q7Gox formation (compare ▲ and △). These results can be explained by the oxidation of Q7G by semiquinone radicals generated during the reactions of (+)-catechin with nitrous acid. Such an interaction between (+)-catechin and quercetin has been reported to proceed in the presence of nitrite under acidic conditions.17
6,8-Dinitrosoepicatechin (2b) was formed in the reaction mixture that contained 50 μM (−)-epicatechin and 0.5 mM sodium nitrite in 50 mM KCl–HCl (pH 2.0), and it was postulated that 2b might be formed by reactions (2)–(5), in which (+)-catechin was replaced by (−)-epicatechin. The formation of 2b was suppressed by Q7G, and the suppression increased with the increase in the concentration of Q7G (■) (Fig. 5B). The consumption of (−)-epicatechin was also suppressed, and the degree of suppression increased with the increase in the concentration of Q7G (□). Furthermore, (−)-epicatechin (50 μM) enhanced the nitrous acid-induced consumption of Q7G as (+)-catechin (compare ● and ○), and the enhancement was accompanied by the increase in Q7Gox formation (compare ▲ and △). The result in Fig. 5B suggests that Q7G can also react with semiquinone radicals generated in (−)-epicatechin/nitrous acid systems.
Interactions of Q7G with 2a/2b
6,8-Dinitrosocatechin (2a) isolated by preparative HPLC in this study was partly transformed into 2b during its concentration and lyophilization. It has been reported that 2a and 2b are mutually transformed.14 Then, we studied the effects of Q7G on nitrous acid-induced oxidation of 2a and 2b using the isolated dinitrosocatechin that was a mixture of 2a and 2b (Fig. 6A). Accompanying the oxidation of 2a/2b by nitrous acid, P1 and P2 were produced as reported previously.17,33 Their formation was completely suppressed by 30 and 100 μM Q7G (■). The concentration of Q7G required for 50% inhibition was estimated to be 7 μM from the figure. It has been reported that 50% inhibition of the formation of (P1 + P2) from 2a/2b is observed at about 10 μM quercetin under conditions similar to Fig. 6A.17 The suppression of (P1 + P2) formation accompanied the inhibition of the consumption of 2a/2b (□). Furthermore, the inhibition of the formation of (P1 + P2) by Q7G accompanied the enhancement of Q7G consumption (compare ● and ○) and Q7Gox formation (compare ▲ and △) (Fig. 6B). These results suggest that Q7G can inhibit the formation of P1 and P2 by scavenging semiquinone radicals produced in 2a/2b/nitrous acid systems by reaction (6), if P1 and P2 are o-quinones derived from 2a and 2b, respectively, as described in Fig. 4.
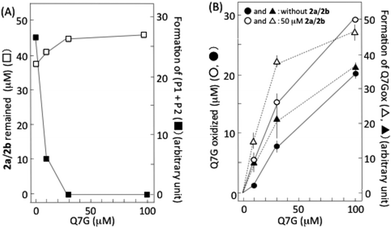 |
| Fig. 6 Interactions of Q7G with 2a/2b. The reaction mixture contained 50 μM 2a/2b and various concentrations of Q7G in 50 mM KCl–HCl (pH 2.0). One min after the addition of 0.5 mM NaNO2 under anaerobic conditions, each component was quantified by HPLC. (A) Q7G-dependent inhibition of 2a/2b oxidation and (P1 + P2) formation. (B) 2a/2b-dependent enhancement of Q7G oxidation. The mobile phase was a mixture of methanol and 0.2% formic acid (2 : 5, v/v). (□) 2a/2b remained in the reaction mixture; (■) formation of P1 + P2; (● and ○) oxidation of Q7G in the absence and presence of 2a/2b, respectively; (▲ and △) formation of Q7Gox in the absence and presence of 2a/2b, respectively. Each data point represents mean with SD (n = 3–4). In (A), error bars are within the squares. | |
˙NO production
During the reactions of nitrite with Q7G, catechin, or 2a/2b under acidic conditions, ˙NO should be produced by reactions (1), (2), (4), and (6). Then, nitrite-induced ˙NO production was studied in the presence of the above flavonoids in 50 mM KCl–HCl (pH 2.0) (Table 1). The rate of ˙NO production in the nitrous acid/Q7G system increased with the increase in Q7G concentration. The rate constant of ˙NO production by Q7G was calculated to be (3.6 ± 0.6) × 103 M−1 min−1 using the data in Table 1, postulating that ˙NO was produced by reaction (1). The rate constant in the presence of quercetin was calculated to be (3.9 ± 0.3) × 103 M−1 min−1. These values were about 2.8-folds of the rate constants for the decreases in concentrations of Q7G [(12.5 ± 1.2) × 102 M−1 min−1] and quercetin [(13.5 ± 1.1) × 102 M−1 min−1]. One of the reasons for the larger rate constants for ˙NO production is the rapid reduction of nitrous acid to ˙NO by semiquinone radicals of not only Q7G but also quercetin and/or rapid disproportionation of the semiquinone radicals to the mother compounds and quinones that are transformed rapidly into 5 from quercetin and a glucoside of 5 from Q7G.38,39 The rate constants of ˙NO production were calculated from the initial slope. This may also be a reason for the larger rate constants of ˙NO production than those of the consumption of Q7G and quercetin.
Table 1 ˙NO production in flavonoid/nitrous acid systemsa
Q7G (μM) |
0 |
5 |
15 |
50 |
˙NO produced (μM min−1) |
Reactions were initiated by addition of 0.5 mM sodium nitrite under anaerobic conditions. Each datum represents mean with SD (n = 3–4). *Significant differences in ˙NO production compared with 50 μM (+)-catechin or 50 μM (−)-epicatechin (P < 0.05). The number in parenthesis is the ratio of ˙NO production rate in the mixture of Q7G with (+)-catechin, (−)-epicatechin, or 2a/2b to the sum of ˙NO production rate by Q7G alone and that by (+)-catechin, (−)-epicatechin, or 2a/2b alone.
|
No addition |
0 |
10.3 ± 2.6 |
29.6 ± 5.5 |
73.8 ± 12.3 |
50 μM (+)-catechin |
20.9 ± 3.8 |
28.9 ± 2.6 (93%) |
41.8 ± 5.2 (83%) |
98.4 ± 19.2 (104%) |
50 μM (−)-epicatechin |
21.5 ± 4.1 |
30.9 ± 2.4 (97%) |
47.7 ± 4.3 (93%) |
100.4 ± 14.4 (105%) |
50 μM 2a/2b |
33.5 ± 4.0* |
50.9 ± 7.1 (116%) |
81.2 ± 9.9 (129%) |
120.7 ± 18.9 (112%) |
Table 1 also shows the rates of ˙NO production in the presence of (+)-catechin, (−)-epicatechin, and isolated 2a/2b. Catechin-induced ˙NO production under stomach simulating conditions has been reported.11,13 Nitrous acid-induced ˙NO production in the presence of 50 μM (+)-catechin or (−)-epicatechin was less than 30%, and the ˙NO production in the presence of 50 μM 2a/2b was about 45% of that in the presence of 50 μM Q7G. The slower ˙NO production by 2a/2b than Q7G can be explained by the difference in the rate constants of nitrous acid-induced oxidation of 2a/2b [(6.5 ± 2.5) × 102 M−1 min−1]17 from that of Q7G [(12.5 ± 1.2) × 102 M−1 min−1] (see above). Although the rate constants of nitrous acid-induced oxidation of (+)-catechin [(6.7 ± 1.0) × 102 M−1 min−1] and (−)-epicatechin [(5.8 ± 0.2) × 102 M−1 min−1] were similar to that of nitrous acid-induced oxidation of 2a/2b,17,34 ˙NO production in the presence of (+)-catechin or (−)-epicatechin was significantly slower than that in the presence of 2a/2b. The difference can be explained by ˙NO consumption by semiquinone radicals derived from (+)-catechin and (−)-epicatechin but not 2a/2b by reactions (3) and (5). The addition of 50 μM 2a/2b to Q7G/nitrous acid systems increased the rate of ˙NO production; the rates in the presence of both 2a/2b and Q7G were larger than the sums of the rate in the presence of 2a/2b alone and the rates in the presence of Q7G alone at any concentrations of Q7G studied, supporting the insignificant reaction of ˙NO with the semiquinone radical of 2a/2b. The addition of 50 μM (+)-catechin or (−)-epicatechin to Q7G/nitrous acid systems increased the rate of ˙NO production, but the rates in the presence of both catechin and Q7G were smaller than the sums of the rate in the presence of (+)-catechin or (−)-epicatechin alone and the rates in the presence of Q7G alone, especially when the concentrations of Q7G were 5 and 15 μM. These data can be explained by the consumption of ˙NO by semiquinone radicals of (+)-catechin and (−)-epicatechin, both of which were produced in catechin/Q7G/nitrous acid systems.
Interactions of (+)-catechin and 2a/2b with other flavonols
Rutin, Q4′G, and kaempferol were consumed by nitrous acid (Table 2, column 1), and the rate constants of the consumption were calculated from the data. The values were (1.3 ± 1.0), (2.8 ± 0.8), and (6.2 ± 1.2) × 102 M−1 min−1 (n = 9) for rutin, Q4′G, and kaempferol, respectively, and the values were smaller than those of Q7G [(12.5 ± 1.2) × 102 M−1 min−1] and quercetin [(13.5 ± 1.1) × 102 M−1 min−1]. These results are in accordance with a previous report on nitrite-induced oxidation of 50 μM quercetin, Q4′G, rutin, and kaempferol in 50 mM KCl–HCl (pH 2.0) (6.2, 0.9, 0.2, and 1.0 μM min−1, respectively).10
Table 2 Interactions between flavonols and (+)-catechin or 2a/2ba
Addition (100 μM) |
Consumption of flavonols (μM min−1) |
Formation of 2a (per min) (arbitrary unit) |
Formation of (P1 + P2) (per min) (arbitrary unit) |
(1) |
(2) |
(3) |
(4) |
(5) |
No addition |
(+)-Catechin |
2a/2b |
(+)-Catechin |
2a/2b |
All reactions were performed in 50 mM KCl–HCl (pH 2.0). One min after the addition of 0.5 mM NaNO2, the reaction mixtures were analyzed by HPLC. Each datum represents mean ± SD (n = 3). a and bSignificant differences between columns 1 and 2 and between columns 1 and 3 (P < 0.05); c and dsignificant inhibition of formation of 2a (column 4) and (P1 + P2) (column 5), respectively, by rutin, Q′4G and kaempferol (P < 0.05). n.s.d., no significant difference between the values without rutin.
|
Without flavonols |
|
|
|
21.2 ± 0.2 |
21.4 ± 2.5 |
Rutin (μM) |
10 |
0.1 ± 0.1 |
0.2 ± 0.1 |
0.7 ± 0.1b |
n.s.d. |
n.s.d. |
30 |
2.5 ± 0.4 |
3.7 ± 0.3a |
3.8 ± 0.3b |
n.s.d. |
n.s.d. |
100 |
10.7 ± 1.1 |
11.4 ± 4.5 |
12.8 ± 2.7 |
n.s.d. |
n.s.d. |
Q4′G (μM) |
10 |
1.0 ± 0.1 |
1.1 ± 0.2 |
3.7 ± 0.5b |
21.1 ± 0.1 |
19.4 ± 1.1 |
30 |
5.3 ± 0.4 |
6.9 ± 0.1a |
9.5 ± 0.2b |
20.8 ± 0.2 |
14.6 ± 0.5d |
100 |
13.9 ± 1.1 |
20.4 ± 0.5a |
23.5 ± 0.4b |
19.7 ± 0.8c |
9.7 ± 0.2d |
Kaempferol(μM) |
10 |
3.3 ± 0.6 |
5.2 ± 0.6a |
4.6 ± 1.0 |
20.0 ± 0.6c |
13.0 ± 1.2d |
30 |
10.7 ± 0.2 |
12.3 ± 1.0a |
12.6 ± 2.4 |
18.9 ± 0.8c |
7.5 ± 0.6d |
100 |
23.8 ± 1.1 |
32.5 ± 3.2a |
41.8 ± 3.0b |
18.0 ± 0.2c |
6.5 ± 0.2d |
The effects of 100 μM (+)-catechin and 2a/2b on nitrous acid-induced consumption of rutin, Q4′G, and kaempferol are also shown in Table 2 (columns 2 and 3). Both (+)-catechin and 2a/2b enhanced the consumption of the above flavonols, and the enhancement of the consumption seemed to increase in the order rutin < Q4′G < kaempferol. Furthermore, Table 2 shows the effects of flavonols on nitrous acid-induced formation of 2a from (+)-catechin (column 4) and (P1 + P2) from 2a/2b (column 5). The formation of 2a and (P1 + P2) was inhibited by Q4′G and kaempferol but not rutin, and the degrees of the inhibition were smaller than those of the inhibition by Q7G (Fig. 5 and 6). The data in Table 2 and Fig. 5 and 6 indicate that both a free hydroxyl group at C3 and ortho-hydroxyl groups in the B ring are essential for the effective inhibition of the nitrous acid-induced transformation of (+)-catechin into 2a and 2a/2b to P1/P2. It has been reported that the hydroxyl group at C3 is a target for the oxidation of flavonols.40–42
Importance of interactions of catechins with flavonols
Flavonoids mixed with saliva in the oral cavity reach the gastric lumen where they can react with nitrous acid derived from nitrite, which is produced by nitrate-reducing bacteria in the oral cavity. The results of this study show that 2a and 2b are possibly produced in the stomach after the ingestion of (+)-catechin- and (−)-epicatechin-containing foods, beverages, or dietary supplements (Fig. 7). The possibility is supported by the reports about the production of 2a and 2b in the mixtures of acidified whole saliva and the methanol extracts of the adzuki bean33 and apple,34 respectively. Recently, however, we observed nitrite-induced formation of 2b by acidification of the juice obtained by mastication of apple, but could not observe the formation of 2a by acidification of the juice obtained by mastication of the boiled adzuki bean (unpublished results). From these results together with the results of the present study and ref. 33 and 34, we can deduce that (i) the detectable amounts of nitrosocatechins are not always produced in the stomach after the ingestion of catechin-rich foods or beverages, and that (ii) the formation of 2a/2b is dependent on the concentration of the components, which can interfere in the reactions of catechins with nitrous acid and can scavenge catechin semiquinone radicals. The formation of 2a/2b in the stomach may be efficient because of the lower O2 concentration in gastric juice. It has been reported on the increase in the efficiency of 2a/2b formation with the decrease in the O2 concentration.33
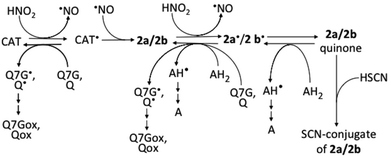 |
| Fig. 7 Possible reactions among nitrous acid, catechins, and 2a/2b. AH2, ascorbic acid; AH, monodehydroascorbic acid; A, dehydroascorbic acid; CAT, catechins; Q, quercetin; Qox, oxidized quercetin; Q7G, quercetin 7-O-glucoside; Q7Gox, oxidized Q7G; ˙, symbol for radicals. Ref. 17 and 33 were referred to prepare this figure. | |
The methanol extract of the boiled aduzki bean contained 138 ± 41, 58 ± 15, 20 ± 4, and 18 ± 2 nmol per g of (+)-catechin, Q7G, quercetin, and vignacyanidins, respectively (means with SDs, n = 3) as phenolic components that could be readily oxidized by nitrous acid. Vignacyanidins are cyanidin-(+)-catechin and -(−)-epicatechin adducts.43 The molar ratio of (+)-catechin to the sum of the other components (about 1.4) and the rate constants of their reactions with nitrous acid (see above) may make possible the reaction of (+)-catechin with nitrous acid to produce 2a, when the methanol extract of the boiled adzuki bean is added to acidified saliva. The failure of observation of 2a formation in the juice obtained by mastication of the boiled adzuki bean might be due to the presence of starch that could suppress the reaction of (+)-catechin and nitrous acid. The oxidation products of quercetin and Q7G, namely, 5 and its glucoside, may decompose to more stable components. It is known that 5 decomposes to 2,4,6-trihydroxyphenyl glyoxylic acid and 3,4-dihydroxybenzoic acid, which are derived from the A- and the B-ring of 5, respectively.44 Taking the report into account, we can deduce that the glucoside of 5 decomposes to a glucoside of 2,4,6-trihydroxyphenyl glyoxylic acid and 3,4-dihydroxybenzoic acid. The oxidation products of vignacyanidins are supposed to be the polymers.43
The major antioxidative polyphenols of apple juice are chlorogenic acid, (−)-epicatechin, and quercetin 3-glycosides such as arabinoside, xyloside, galactoside, glucoside, and rhamnoside.45,46 The molar ratio of (−)-epicatechin to chlorogenic acid is about 0.534 and that of (−)-epicatechin to quercetin glycosides is calculated to range from about 1.6 to 4 using the data in ref. 47. In the apple polyphenols, (−)-epicatechin can react more rapidly with nitrous acid than chlorogenic acid and quercetin 3-glycosides. This is deduced by comparing the rate constant of the reaction of nitrous acid with (−)-epicatechin [(5.8 ± 0.2) × 102 M−1 min−1] with those of reactions of nitrous acid with chlorogenic acid [(1.6 ± 0.3) × 102 M−1 min−1]34 and a quercetin 3-glycoside, rutin [(1.3 ± 1.0) × 102 M−1 min−1] (see above). Then, the observation of nitrite-induced 2b formation in the acidified apple juice can be explained by the more efficient reaction of nitrous acid with (−)-epicatechin than with the other polyphenols. According to the above discussion, the ingestion of a dietary supplement of catechins is supposed to result in the production of 2a/2b in the stomach because of the absence of polyphenols that suppress the nitrosation. The nitrous acid-induced formation of 6,8-dinitrosoepigallocatechin gallate and dinitrosoprocyanidin B2 from epigallocatechin gallate and procyanidin B2, respectively, has also been reported.14,30
If 2a and/or 2b are produced in the gastric lumen after the ingestion of catechin-rich foods, beverages, or dietary supplements, 2a/2b can be oxidized to the o-quinones. The o-quinones may be absorbed in the body from the stomach and the intestine to cause oxidative stress in cells. The stress may be due to the increased reactivity of o-quinones under neutral conditions than acidic conditions. The increased reactivity may contribute to the cytotoxic and carcinogenic effects through generation of reactive oxygen species, formation of polymers and glutathione-conjugate, binding to DNA, and alkylation of essential macromolecules such as DNA and proteins.48–51 Fortunately, the formation of o-quinones from 2a/2b may not be so efficient in the stomach, because ascorbic acid52 and thiocyanate53 derived from gastric juice and saliva, respectively, are present in gastric juice. The former can reduce both semiquinone radicals and o-quinones to 2a/2b, and the latter can react with o-quinones generating thiocyanate conjugates (Fig. 7). Furthermore, flavonols such as quercetin and Q7G can effectively suppress the formation of o-quinone by reducing the semiquinone radicals (Fig. 7).
It has been reported that daily consumption of an aqueous green tea extract does not impair human health,54 but high doses of catechins and other flavonoids as dietary supplements have adverse effects on human health.55–57 The adverse effects of catechins are discussed to be due to the production of undesired products from catechins in the intestine and the liver.56,57 The present study suggests that the nitrosation of catechins and the oxidation of 2a/2b in the stomach may also contribute to the adverse effects of high doses of catechins, if the 2a/2b and the quinones are absorbed in the body from the gastrointestinal tract. It has been reported that, in addition to quinones, 2a/2b are toxic for Caco cells.14
Experimental
Reagents
(+)-Catechin (1a), (−)-epicatechin (1b), quercetin (3), kaempferol, and rutin were obtained from Sigma-Aldrich Japan (Tokyo). 1-Hydroxy-2-oxo-3-(N-methyl-3-aminopropyl)-3-methyl-1-triazene (NOC 7) (purity > 90%) was obtained from Dojindo (Kumamoto, Japan). β-Glucosidase from sweet almond was obtained from Oriental Yeast Co., Ltd (Osaka, Japan). Quercetin 4′-glucoside (Q4′G) was isolated from onion bulbs as reported previously with some modifications.58
Apparatus
UV/visible absorption spectra were recorded using an UV-2450 spectrophotometer (Shimadzu, Kyoto, Japan). Analytical and preparative HPLC was carried out using a Shimadzu LC-10AS pump combined with a SPD M10Avp photodiode array detector (Shimadzu). The columns used for analytical HPLC were a Shim-pack CLC-ODS (15 cm × 6 mm i.d.) and a Shim-pack VP-ODS (15 cm × 4.6 mm i.d.) (Shimadzu), and the column used for preparative HPLC was a Shim-pack CLC-ODS (25 cm × 2 cm i.d.) (Shimadzu). The mobile phases were mixtures of methanol and 0.2% formic acid or methanol and 25 mM KH2PO4, and their flow rates were 1 and 9 mL min−1 for analytical and preparative HPLC, respectively. Atmosphere-pressure chemical ionization (APCI) and electrospray ionization (ESI) mass spectra were obtained with a Shimadzu LCMS QP8000α quadrupole mass spectrometer equipped with an APCI or ESI ion source. The sample was delivered into the ion source using an Ascentis express C18 column (15 cm × 2.1 mm i.d; particle size, 2 μm) (Sigma-Aldrich Japan, Tokyo). The mobile phase was 40% methanol containing 0.2% formic acid and the flow rate was 0.2 mL min−1. 1H and 13C nuclear magnetic resonance (NMR) spectra were recorded with an ECX-400P FT-NMR spectrometer (JEOL, Tokyo, Japan) with dimethylsulfoxide-d6 (DMSO-d6) as the solvent and tetramethylsilane as the internal standard.
Isolation of quercetin Q7G
Q7G was isolated by the method developed in this study. Dried adzuki seeds (300 g) were boiled in 700 ml of distilled water for 30 min, and the water extract was filtered under reduced pressure. The pH of the filtrate was adjusted to 2.0 by adding 6 M HCl and then extracted with 200 mL of ethyl acetate. For better separation of the ethyl acetate from water, the mixture was centrifuged at 3000g for 5 min. The above ethyl acetate extraction procedure was repeated three times, and ethyl acetate fractions were combined. After removing water in the ethyl acetate fraction by anhydrous sodium sulfate, ethyl acetate was evaporated in vacuo. The residue was dissolved in 2 mL of a mixture of methanol and 25 mM KH2PO4 (2
:
3, v/v), and then applied to the preparative HPLC column. After washing the column with a mixture of methanol and 25 mM KH2PO4 (1
:
6, v/v) for 5 min, the concentration of methanol in the mobile phase was increased stepwise using the following mixtures [methanol and 25 mM KH2PO4 (1
:
3, 1
:
2, and 2
:
3, v/v)]. Each mobile phase was flowed for 20 min, and Q7G was detected at 360 nm.
A compound assigned to be Q7G was eluted at about 7 min after the mobile phase was changed to the mixture of methanol and 25 mM KH2PO4 (2
:
3, v/v). The fraction of Q7G was collected and solvents in the fraction were evaporated in vacuo. The residues were dissolved in 2 mL of methanol and purified by repetition of dissolution in methanol and precipitation with water. The purity of Q7G was estimated by analytical HPLC using a Shim-pack VP-ODS column (15 cm × 4.6 mm i.d.). The mobile phase was a mixture of methanol and 25 mM KH2PO4 (2
:
3, v/v) and the flow rate was 1.0 mL min−1. When no significant contaminants were detected, the precipitate was lyophilized (35 mg from 2.1 kg of adzuki bean). The structure of isolated Q7G was confirmed by spectral data:5,59 UV-vis (methanol) λmax/nm (ε) 255 (22
000) and 373 (19
500); negative APCI-MS m/z (relative intensity, %) 301, (40, [M – glucose]−) and 463 (100, [M − H]−); negative ESI-MS m/z 463 (100, [M − H]−); 1H NMR (DMSO-d6) δ 3.20 (m, 1H, H-4′′), 3.29 (m, 1H, H-2′′), 3.32 (m, 1H, H-3′′), 3.47 (m, 1H, H-5′′), 3.49 (m, 1H, H-6′′a), 3.73 (m, 1H, H-6′′b), 5.09 (d, J = 7.3 Hz, 1H, H-1′′), 6.43 & 6.44 (d, J = 2.2 Hz, 1H, H-6), 6.78 (d, J = 2.2 Hz, 1H, H-8), 6.91 & 6.92 (d, J = 8.7 Hz, 1H, H-5′), 7.57 & 7.58 (dd, J = 8.2, 2.3 Hz, 1H, H-6′), 7.73 & 7.74 (d, J = 2.2 Hz, 1H, H-2′), 12.52 (s, 1H, OH); 13C NMR (DMSO-d6) δ 61.17 (C-6′′), 70.11 (C-4′′), 73.67 (C-2′′), 76.95 (C-3′′), 77.69 (C-5′′), 94.80 (C-8), 99.29 (C-6), 100.44 (C-1′′), 105.21 (C-4a), 115.90 (C-2′), 116.12 (C-5′), 120.59 (C-6′), 122.34 (C-1′), 136.62 (C-3), 145.61 (C-4′), 148.13 (C-2), 148.48 (C-3′), 156.26 (C-8a), 160.90 (C-5), 163.22 (C-7), 176.55 (C-4).
Isolation of 6,8-dinitrosocatechin
6,8-Dinitrosocatechin was isolated as reported previously.17,33 In brief, (+)-catechin (29 mg) in 100 mL of 50 mM KCl–HCl (pH 2.0) was incubated with 5 mM nitrite for 5 min under anaerobic conditions, and then the reaction mixture was extracted with 50 mL of ethyl acetate. After removing ethyl acetate, the residue was dissolved in 3 mL of 57% methanol in 0.2% formic acid (v/v) to apply to the preparative HPLC column. The mobile phases were mixtures of methanol and 0.2% formic acid. The concentration of methanol was increased stepwise as follows, 14, 20, 25, and 33% (v/v), and the mobile phases were flowed for 5, 15, 15, and 25 min, respectively. A fraction assigned to be 6,8-dinitrosocatechin, which was eluted around 7 min after changing the mobile phase to 20% methanol, was collected. After removing the methanol in the fraction, the residue was lyophilized. The yield was 8–10 mg. LC-MS analysis of the isolated dinitrosocatechin gave two peaks (2a and 2b) with retention times of 2.1 and 3.4 min, respectively, when the mobile phase was 35% of methanol in 0.2% formic acid. The structures were confirmed to be a mixture of 6,8-dinitrosocatechin (2a) and 6,8-dinitrosoepicatechin (2b) as reported previously.17,33
Reactions of Q7G, catechin, and 6,8-dinitrosocatechin with nitrous acid
All reactions of flavonoids with nitrite were studied in 1 mL of 50 mM KCl–HCl (pH 2.0) under aerobic or anaerobic conditions. Anaerobic conditions were established by bubbling argon gas through the reaction mixture for 2 min. After initiating the reactions by adding 0.5 mM sodium nitrite, argon gas was blown gently on the surface of the reaction mixture during the reaction period.
The interactions of Q7G with (+)-catechin, (−)-epicatechin, or isolated 6,8-dinitrosocatechins (2a/2b) were studied in the reaction mixture (1 mL) that contained various concentrations of Q7G and 50 μM (+)-catechin, (−)-epicatechin, or 2a/2b in 50 mM KCl–HCl (pH 2.0). When required, quercetin and other flavonols were used instead of Q7G. Reactions were initiated by adding 0.5 mM sodium nitrite. After incubation for 1 min, 50 μL of the reaction mixture was analyzed by HPLC (see below).
HPLC analysis of reaction products
The reactants and the products of the above reactions were separated and quantified using a Shim-pack CLC-ODS column. The mobile phases used to quantify (+)-catechin, (−)-epicatechin, and their products were mixtures of methanol and 0.2% formic acid (1
:
4, 1
:
3, or 1
:
2, v/v). The products of 2a and 2b formed in the presence and absence of Q7G or quercetin were quantified using a mixture of methanol and 0.2% formic acid (2
:
5, v/v), and the ratios of 1
:
1 (v/v) were used to quantify rutin, Q7G and the reaction products of Q7G and quercetin. Quercetin, Q4′G, and kaempferol were quantified using the mixture of 2
:
1 (v/v). The concentrations of reactants and products were estimated from the areas under the peaks: (+)-catechin and (−)-epicatechin at 280 nm; 2a, 2b, P1, and P2 at 320 nm; Q7G, quercetin, rutin, and Q4′G at 360 nm; Qox and Q7Gox at 290 nm.
Hydrolysis of reaction products of Q7G
Q7G was oxidized in the reaction mixture (1 mL) that contained 0.1 mM Q7G and 0.2 mM sodium nitrite in 50 mM KCl–HCl (pH 2.0) for 6 min, and then 0.3 mL of 0.1 M Na2HPO4 was added to adjust the pH to 6.8. The solution of pH 6.8 was incubated for 10, 20, 30, and 40 min after addition of 20 μL of β-glucosidase (2 mg mL−1), and an aliquot (50 μL) of each incubated solution was applied to a Shim-pack VP-ODS column (15 cm × 4.6 mm i.d.). The mobile phase was a mixture of methanol and 25 mM KH2PO4 (1
:
2, v/v).
Measurements of ˙NO production
Nitrite-induced ˙NO production was recorded using a Clark-type electrode (Rank Bothers, Cambridge, UK) at 30 °C with a polarization voltage of −0.7 V.25,60 The reaction mixture (2 mL) contained 5, 15, and 50 μM Q7G in 50 μM KCl–HCl (pH 2.0). When required, 50 μM (+)-catechin, (−)-epicatechin, or 2a/2b was added. After removing air from the reaction mixture by bubbling argon gas, 0.5 mM sodium nitrite was added to initiate ˙NO production. The rate of ˙NO production was estimated from the slope, using an ascorbic acid–nitrous acid system for calibration.
In the ascorbic acid–nitrous acid system, one molecule of ascorbic acid produces two molecules of ˙NO under acidic conditions by the following reaction, if the concentration of nitrous acid is more than two times of that of ascorbic acid
| Ascorbic acid + 2HNO2 → Dehydroascorbic acid + 2˙NO + 2H2O | (8) |
The amount of ˙NO produced by addition of 50 μM ascorbic acid in the presence of 0.5 mM sodium nitrite in 50 mM KCl–HCl (pH 2.0) was essentially the same as the amount produced by 50 μM NOC 7 in the same buffer, one molecule of which produces two molecules of ˙NO, indicating the usefulness of an ascorbic acid–nitrous acid system for calibration of ˙NO production.
Presentation of data
Each experiment was repeated more than three times. Typical data or means with SDs are presented in figures and tables. The statistical significance of the differences between groups was evaluated by Student's t-test.
Conclusion
The ingestion of nitrate-rich leafy vegetables such as lettuce and spinach results in the increase in the concentration of nitrite in mixed whole saliva.7,61 If catechin-rich foods, beverages, or dietary supplements are taken under such conditions, nitrosation of catechins and oxidation of 2a/2b to the o-quinones can proceed in the stomach. The nitrous acid-induced formation of 2a/2b and the o-quinones can be suppressed by ascorbic acid in gastric juice cooperating with antioxidative flavonols in foods and beverages. Thus, the results of the present study suggest that the ingestion of catechin-rich dietary supplements accompanied with components which can reduce the formation of semiquinone radicals of catechins and 2a/2b may decrease the adverse effects of catechins.
Abbreviations
Q7G | Quercetin 7-glucoside |
Q4′G | Quercetin 4′-glucoside |
Qox | Oxidation product of quercetin |
Q7Gox | Oxidation product of quercetin 7-O-glucoside |
Acknowledgements
Part of this study was supported by Grants-in-Aid for Scientific Research (22500790 and 23500986) from the Ministry of Education and Science in Japan. S. Veljovic-Jovanovic and F. Morina acknowledge the partial support from Ministry of Education, Science and Technological Development of Republic Serbia (III43010).
References
- C. Ölschläger, I. Regos, F. J. Zeller and D. Treutter, Phytochemistry, 2008, 69, 1389–1397 CrossRef PubMed.
-
S. Bhagwat, D. B. Haytowitz and J. M. Holden, USDA database for the flavonoid content of selected foods. Release 3.1; 2013, Available from http://www.ars.usda.gov/SP2UserFiles/Place/12354500/Data/Flav/Flav3-1.pdf.
- A.-M. Danila, A. Kotani, H. Hakamata and F. Kusu, J. Agric. Food Chem., 2007, 55, 1139–1143 CrossRef CAS PubMed.
- K. Yoshida, T. Kondo, M. Ito and T. Kondo, ITE Lett. Batter., New Technol. Med., 2005, 6, C1–C6 Search PubMed.
- S.-I. Tebayashi, S. Matsuyama, T. Suzuki, Y. Kuwahara, T. Nemoto and K. Fujii, J. Pestic. Sci., 1995, 20, 299–305 CrossRef CAS.
- J. J. Doel, N. Benjamin, M. P. Hector, M. Rogers and R. P. Allaker, Eur. J. Oral Sci., 2005, 113, 14–19 CrossRef CAS PubMed.
- A. S. Pannala, A. R. Mani, J. P. E. Specer, Y. Skinner, K. R. Bruckdorfer, K. P. Moore and C. A. Rice-Evans, Free Radical Biol. Med., 2003, 34, 576–584 CrossRef CAS.
- P. Janeiro and A. M. O. Brett, Anal. Chim. Acta, 2004, 518, 109–115 CrossRef CAS PubMed.
- A. M. O. Brett and M.-E. Ghica, Electoroanalysis, 2003, 15, 1745–1750 CrossRef CAS.
- U. Takahama, T. Oniki and S. Hirota, J. Agric. Food Chem., 2002, 50, 4317–4322 CrossRef CAS PubMed.
- L. Peri, D. Pietraforte, G. Scorza, A. Napolitano, V. Fogliano and M. Minetti, Free Radical Biol. Med., 2005, 39, 668–681 CrossRef CAS PubMed.
- B. Gago, J. O. Lundberg, R. M. Barbosa and J. Laranjinha, Free Radical Biol. Med., 2007, 43, 1233–1242 CrossRef CAS PubMed.
- B. S. Rocha, B. Gago, R. M. Barbosa and J. Laranjinha, Toxicology, 2009, 265, 41–48 CrossRef CAS PubMed.
- S. Y. H. Lee, B. Muerol, S. Pollard, K. A. Youdim, A. S. Pannala, G. G. C. Kuhnle, E. S. Debnam, C. Rice-Evans and J. P. E. Spencer, Free Radical Biol. Med., 2006, 40, 323–334 CrossRef CAS PubMed.
- S. Hirota, U. Takahama, T. N. Ly and R. Yamauchi, J. Agric. Food Chem., 2005, 53, 3265–3272 CrossRef CAS PubMed.
- T. N. Ly, C. Hazama, M. Shimoyamada, H. Ando, K. Kato and R. Yamauchi, J. Agric. Food Chem., 2005, 53, 8183–8189 CrossRef CAS PubMed.
- S. Veljovic-Jovanovic, F. Morina, R. Yamauchi, S. Hirota and U. Takahama, J. Agric. Food Chem., 2014, 62, 4951–4959 CrossRef CAS PubMed.
- B. S. Rocha, C. Nunes, C. Pereira, R. M. Barbosa and J. Laranjinha, Food Funct., 2014, 5, 1646–1652 CAS.
- N. Benjamin, F. O'Driscoll, H. Dougall, C. Duncan, L. Smith, M. Golden and H. McKenzie, Nature, 1994, 368, 502 CrossRef CAS PubMed.
- J. Xu, X. Xu and W. Verstraete, J. Appl. Microbiol., 2001, 90, 523–529 CrossRef CAS.
- M. Miyoshi, E. Kasahara, A. M. Park, K. Hiramoto, Y. Minamiyama, S. Takemura, E. F. Sato and M. Inoue, Free Radical Res., 2003, 37, 85–90 CrossRef CAS.
- H. H. Björne, J. Petersson, M. Phillipson, E. Weizberg, L. Holm and J. O. Lundberg, J. Clin. Invest., 2004, 113, 106–114 CrossRef PubMed.
- J. Petersson, M. Phillipson, E. A. Jansson, A. Patzak and J. O. Lundberg, Am. J. Physiol. Gastrointest. Liver Physiol., 2007, 292, G718–G724 CrossRef CAS PubMed.
- J. O. Lundberg, E. Weitzberg and M. T. Gladwin, Nat. Rev. Drug Discovery, 2008, 7, 156–167 CrossRef CAS PubMed.
- J. Volk, S. Gorelik, R. Granit, R. Kohen and J. Kanner, Free Radical Biol. Med., 2009, 47, 496–502 CrossRef CAS PubMed.
- M. Eichholzer and F. Gutzwiller, Nutr. Rev., 1998, 56, 95–105 CrossRef CAS PubMed.
- K. Ohsawa, S. Y. Nakagawa, M. Kimura, C. Shimada, S. Tsuda, K. Kabasawa, S. Kawaguchi and Y. F. Sasaki, Mutat. Res., 2003, 539, 65–76 CAS.
- M. De Lucia, L. Panzella, A. Pezzella, A. Napolitano and M. D'Ischia, Chem. Res. Toxicol., 2008, 21, 2407–2413 CrossRef CAS PubMed.
- B. S. Rocha, B. Gago, C. Pereira, R. M. Barbosa, S. Bartesaghi, J. O. Lundberg, R. Radi and J. Laranjinha, Curr. Drug Targets, 2011, 12, 1351–1363 CrossRef CAS.
- L. Panzella, P. Manini, A. Napolitano and M. d'Ischia, Chem. Res. Toxicol., 2005, 18, 722–729 CrossRef CAS PubMed.
- K. Tanaka, T. Hayatsu, T. Negishi and H. Hayatsu, Mutat. Res., 1998, 12, 91–98 Search PubMed.
- S. Masuda, S. Uchida, Y. Terashima, H. Kuramoto, M. Serozawa, Y. Deguchi, K. Yanai, C. Sugiyama, I. Oguni and N. Kinae, J. Health Sci., 2006, 52, 211–220 CrossRef CAS.
- U. Takahama, R. Yamauchi and S. Hirota, Free Radical Res., 2014, 48, 956–966 CrossRef CAS PubMed.
- S. Hirota and U. Takahama, Food Sci. Technol. Res., 2014, 20, 439–447 CrossRef CAS.
-
J. Terao and M. K. Piskula, in Flavonoids in Health and Disease, ed. C. A. Rice-Evans and L. Packer, Marcel Dekker, New York, 1998, pp. 277–293 Search PubMed.
- U. Takahama, M. Tanaka, T. Oniki, S. Hirota and R. Yamauchi, J. Agric. Food Chem., 2007, 55, 4169–4176 CrossRef CAS PubMed.
- U. Takahama, M. Tanaka and S. Hirota, Free Radical Res., 2010, 44, 293–303 CrossRef CAS PubMed.
- A. K. Timbola, C. D. de Souza, C. Giacomelli and A. Spinelli, J. Braz. Chem. Soc., 2006, 17, 139–148 CrossRef CAS PubMed.
- A. M. Bondžić, T. D. Lazarević-Pašti, B. P. Bondžić, M. B. Čolović, M. B. Jadranin and V. M. Vasić, New J. Chem., 2013, 37, 901–908 RSC.
- L. V. Jørgensen, C. Claus, J. Ulla, L. H. Skibsted and L. O. Dragsted, Free Radical Res., 1998, 29, 339–350 CrossRef.
- I. G. Zenkevich, A. Y. Eshchenko, S. V. Makarova, A. G. Vitenberg, Y. G. Dobryakov and V. A. Utsal, Molecules, 2007, 12, 654–672 CrossRef CAS.
- H. Ohashi, T. Kyogoku, T. Ishikawa, S. Kawase and S. Kawai, J. Wood Sci., 1999, 45, 53–63 CrossRef CAS.
- U. Takahama, R. Yamauchi and S. Hirota, Food Chem., 2013, 141, 2600–2606 CrossRef CAS PubMed.
- B. M. Fahlman and E. S. Krol, J. Photochem. Photobiol., B, 2009, 97, 7123–7131 CrossRef PubMed.
- Y. Lu and L. Y. Foo, Food Chem., 1997, 59, 187–194 CrossRef CAS.
- J.-S. Xiao, L. Liu, H. Wu, B.-J. Xie, E.-N. Yang and Z.-D. Sun, J. Agric. Food Chem., 2008, 56, 2096–2101 CrossRef CAS PubMed.
- C. Manach, A. Scalbert, C. Morand, C. Remesy and L. Jimenez, Am. J. Clin. Nutr., 2004, 79, 727–747 CAS.
- J. L. Bolton, M. A. Trush, T. M. Penning, G. Dryhurst and T. J. Monks, Chem. Res. Toxicol., 2000, 13, 135–140 CrossRef CAS PubMed.
- A. G. Siraki, T. S. Chan and P. J. O'Brien, Toxicol. Sci., 2004, 81, 148–159 CrossRef CAS PubMed.
- P. J. O'Brien, Chem.-Biol. Interact., 1991, 80, 1–41 CrossRef.
- E. L. Cavalieri, K.-M. Li, N. Balu, M. Saeed, P. Devanesan, S. Higginbotham, J. Zhao, M. L. Gross and E. G. Rogan, Carcinogenesis, 2002, 23, 1071–1077 CrossRef CAS PubMed.
- G. M. Sobala, C. J. Schorah, M. Sanderson, M. F. Dixon, D. S. Tompkins, P. Godwin and A. T. Axon, Gastroenterology, 1989, 97, 357–363 CAS.
- C. P. Schultz, M. K. Ahmed, C. Dawes and H. H. Mantsch, Anal. Biochem., 1996, 240, 7–12 CrossRef CAS PubMed.
- J. Frank, T. W. George, J. K. Lodge, A. M. Rodriguez-Mateos, J. P. E. Spencer, A. M. Minihane and G. Rimbach, J. Nutr., 2009, 139, 58–62 CrossRef CAS PubMed.
- S. Egert and G. Rimbach, Adv. Nutr., 2011, 2, 8–14 CrossRef CAS PubMed.
- A. H. Schonthal, Mol. Nutr. Food Res., 2011, 55, 874–875 Search PubMed.
- J. D. Lambert, S. Sang and C. S. Yang, Mol. Pharmaceutics, 2007, 4, 819–825 CrossRef CAS PubMed.
- S. Hirota, T. Shimoda and U. Takahama, J. Agric. Food Chem., 1998, 46, 3497–3502 CrossRef CAS.
-
L. Jurd, in The Chemistry of Flavonopid Compounds, ed. T. A. Geissman, Macmillian Co., New York, 1962, pp. 107–155 Search PubMed.
- U. Takahama and S. Hirota, Chem. Res. Toxicol., 2012, 25, 207–215 CrossRef CAS PubMed.
- C. P. Bondonno, L. A. Downey, K. D. Croft, A. Scholey, C. Stough, X. Yang, M. J. Considine, N. C. Ward, I. B. Puddey, E. Swinny, A. Mubarak and J. M. Hodgson, Food Funct., 2014, 5, 849–858 CAS.
|
This journal is © The Royal Society of Chemistry 2015 |