DOI:
10.1039/C3SC52106K
(Edge Article)
Chem. Sci., 2014,
5, 90-100
Topological isomerism in a chiral handcuff catenane†
Received
27th July 2013
, Accepted 4th September 2013
First published on 5th September 2013
Abstract
The self-assembly of two topological isomers of a handcuff catenane has been achieved by utilizing the template-directed synthesis between the π-electron-rich bis-1,5-dioxynaphtho[50]crown-14 and the precursors to two fused π-electron-deficient cyclobis(paraquat-p-phenylene) cyclophanes. Characterization of the product using 1H NMR spectroscopy and single-crystal X-ray diffraction, with supporting density functional theory (DFT) calculations, suggests that the 1,5-dioxynaphthalene units in the major topological isomer align themselves with the same relative orientations inside the cyclophanes on account of restrictions imposed by the lengths of the polyether loops. The DFT calculations also reveal that the energies of the two topological isomers are similar to each other, supporting the experimental observation that both isomers can be isolated as a mixture from a one-pot reaction. The two isomers – designated as the meta–meta and ortho–ortho isomers with different topologies that are not interconvertible – only differ in the manner in which the polyether loops wind their way around the central 1,2,4,5-tetrasubstituted benzenoid ring in the ditopic host. X-Ray crystallography proves that by far the major topological isomer in the solid state is the meta–meta one. 1H NMR spectroscopy confirms that it is also the major isomer in solution, whilst also revealing the presence of a minor isomer, which is assumed, for the time-being, to have the ortho–ortho topology. The free fused ditopic host has been obtained using a protocol similar to that employed in the template-directed synthesis of the handcuff catenane, except that the crown ether is replaced with an acyclic template which can be removed post-synthesis. The results of isothermal titration calorimetry studies shed some light on the mechanism of binding of π-electron-rich guests: they lead us to believe that when two electron-rich guests bind to the ditopic host, they do so with allosteric negative cooperativity.
Introduction
Although they can have visually appealing chemical topologies1 and can potentially be incorporated into artificial molecular machines2 (AMMs) and artificial molecular switches3 (AMSs) in, for example, molecular electronic devices4 (MEDs), compounds containing mechanically interlocked molecules1,5–12 (MIMs) pose a considerable challenge when it comes to considering their chemical synthesis. Going back over half a century, various synthetic strategies for the production of MIMs have been pursued: aside from statistical methods5 employed early on, MIMs are usually prepared nowadays in good yields using template-directed protocols, e.g., covalent6 and coordinative,1b–e,g,h,j,m,n as well as a range of noncovalent bonding interactions,7–12 where the molecular recognition has most notably been sustained by donor–acceptor,7 cation8 and anion,9 hydrogen bonding10 (both neutral10a–c and charged10d,e based), hydrophobic11 and, in recent times, radical–radical interactions.12
A handcuff catenane is an example (Fig. 1) of a MIM with an architecture which consists of two covalently bound rings—call it a bis-macrocycle or a ditopic host—mechanically interlocked by a third ring that is threaded through both rings of the ditopic host. Three previous examples of MIMs with this topology have been reported8b,c,9b,13 in the literature. The research conducted by Li and Becher13 on handcuff catenanes relied upon donor–acceptor interactions between an electron-rich tetrathiafulvalene-based bis-macrocycle and an electron-deficient-cyclobis(paraquat-p-phenylene) (CBPQT4+) ring, whilst Sauvage et al.8b,c have made use of Cu(I) coordination to 1,10-phenanthroline derivatives to template the formation of handcuff catenanes. Chloride anion templation between a bis-macrocycle and two threaded components, followed by their cyclization to afford a handcuff catenane has been reported recently by Beer et al.9b who also described the first solid-state structure of this higher order MIM.
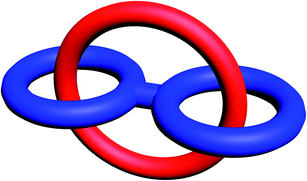 |
| Fig. 1 Generic topology of a handcuff catenane. | |
We recall now the fact that we enter the realm of chemical topology14 when considering the molecular structures of catenanes and doubly interlocked catenanes, e.g., the Solomon link, which is related to a handcuff catenane insofar as both these MIMs contain two components and four crossing points. There is an important distinction, however, between the Solomon links and handcuff catenanes reported to date. The former exhibit chirality—that is, they exist either as enantiomers or diastereoisomers—as a result of being unconditionally topologically chiral14,15 while the latter—at least all those examples reported to date—are achiral, although they can be designed to be topologically chiral.14–16 The previous examples of handcuff catenanes reported in the literature, however, are not only all achiral, but they also exhibit only one topology, i.e., there is only one way in which the large ring winds its way through both rings of the ditopic host.
Herein, we describe (i) the synthesis and solid-state structure of a free ditopic host DBB·8PF6 based on a fused [CBPQT]28+ species,17 (ii) binding studies between electron-rich guests and DBB·8PF6 which shed light on the mechanism by which catenation may occur, (iii) the formation of a topologically chiral handcuff catenane HC·8PF6, comprised of a π-electron-deficient ditopic host consisting of two fused CBPQT4+ cyclophanes, interlocked with a π-electron-rich crown ether,18 namely bis-1,5-dioxynaphtho[50]crown-14 (DN50C14), (iv) the solid-state structure of this handcuff catenane, (v) the two topological diastereoisomers that can possibly be generated, and (vi) the relative stabilities of these diastereoisomers determined by DFT calculations. Catenation of this fused [CBPQT]28+ has been reported19 previously in the presence of bisparaphenylene[34]crown-10 (BPP34C10), where a bis[2]catenane was isolated, but no single crystals suitable for solid-state structure analysis could be obtained.
Experimental section
General methods
All reagents were purchased from commercial suppliers and used without further purification. Compounds 2·2PF6,20DN50C14 (ref. 18) and BHEEN21 were prepared according to literature procedures. Analytical thin-layer chromatography (TLC) was performed on aluminium sheets, precoated with silica gel 60-F254 (Merck 5554). Flash column chromatography was carried out using silica gel 60 (Silicycle) as the stationary phase. Compound purification was performed on a preparative RP-HPLC instrument (Shimadzu LC-8A), using a C18 column (Agilent, 10 μm packing, 30 × 250 mm). The eluents used were MeCN and H2O, both mixed with 0.1% (v/v) trifluoroacetic acid (TFA). The detector was set to λ = 254 nm. Nuclear magnetic resonance (NMR) spectra were recorded at 298 K on Bruker Avance III 500 and 600 MHz spectrometers, with working frequencies of 499.373 and 600.168 MHz for 1H, and 125.579 and 150.928 MHz for 13C nuclei, respectively. All 13C NMR spectra were recorded with the simultaneous decoupling of 1H nuclei. Chemical shifts are reported in ppm relative to the signals corresponding to the residual non-deuterated solvents (1.94 ppm for CHD2CN). Electrospray Ionization (ESI) mass spectra were obtained on an Agilent 6210 LC-TOF high resolution mass spectrometer.
DBB·8PF6
1,2,4,5-Tetrabromomethyl benzene (1) (171 mg, 0.38 mmol), 1,1′-[1,4-phenylene-bis(methylene)]-bis(4,4′-bipyridinium)-bis(hexafluorophosphate)20 (2·2PF6) (0.54 g, 0.75 mmol), and 1,5-bis[2-(2-hydroxyethoxy)ethoxy]naphthalene21 (BHEEN) (0.77 g, 2.3 mmol) were combined in anhydrous DMF (10 mL). The reaction mixture was subjected to a pressure of 15 kbar in a Teflon high-pressure reaction vessel for 3 days. Solvent was removed in vacuo and the residue was dissolved in saturated aqueous NH4Cl solution and liquid–liquid extraction with CHCl3 was employed for 2 days to remove BHEEN. The aqueous layer was isolated and the crude product was precipitated by addition of NH4PF6 and collected by filtration. The residue was purified by reverse phase high-performance liquid chromatography (RP-HPLC) (H2O–MeCN/0 → 50% in 65 min) to yield a white solid DBB·8PF6 (123.0 mg, 17%). 1H NMR (CD3CN, 600 MHz, 298 K): δ = 8.91 (d, 3J = 7 Hz, 4H), 8.68 (d, 3J = 7 Hz, 4H), 8.20 (d, 3J = 6.4 Hz, 4H), 8.15 (d, 3J = 6.4 Hz, 4H), 7.62 (s, 2H), 7.55 (s, 8H), 6.00 (d, 3J = 14.8 Hz, 4H), 5.77 (s, 8H), 5.70 (d, 3J = 14.8 Hz, 4H) ppm. 13C NMR (CD3CN, 125 MHz, 298 K): δ = 150.1, 149.7, 145.1, 144.7, 136.5, 135.8, 135.3, 129.9, 127.4, 127.2, 64.4, 60.2 ppm. ESI-MS: calcd for [M − 2PF6]2+, m/z = 916.1316, found: m/z = 916.1294.
HC·8PF6
1,2,4,5-Tetrabromomethyl benzene (1) (42 mg, 0.09 mmol), 1,1′-[1,4-phenylene-bis(methylene)]bis(4,4′-bipyridinium) bis(hexafluorophosphate)20 (2·2PF6) (130 mg, 0.18 mmol), and DN50C14 (ref. 18) (75 mg, 0.09 mmol) were combined in anhydrous DMF (10 mL). The reaction mixture was subjected to a pressure of 15 kbar in a Teflon high-pressure reaction vessel for 3 days. Solvent was removed in vacuo and the residue was purified by reverse phase high-performance liquid chromatography (RP-HPLC) (H2O–MeCN/0 → 50% in 65 min) to yield a purple solid HC·8PF6 (22.0 mg, 7.4%). 1H NMR (CD3CN, 600 MHz, 298 K): δ = 9.11 (d, 3J = 6.4 Hz, 2H), 9.07 (m, 4H), 8.98 (s, 2H), 8.95 (d, 3J = 6.4 Hz, 2H), 8.88 (d, 3J = 6.4 Hz, 2H), 8.73 (m, 4H), 8.69 (d, 3J = 6.4 Hz, 2H), 8.07 (s, 4H), 7.99 (s, 4H), 7.74 (d, 3J = 6.4 Hz, 2H), 7.71 (d, 3J = 6.4 Hz, 2H), 7.68 (d, 3J = 6.4 Hz, 2H), 7.47 (m, 6H), 7.40 (d, 3J = 6.4 Hz, 2H), 7.28 (d, 3J = 6.4 Hz, 2H), 6.66 (d, 2J = 13.7 Hz, 2H), 6.46 (m, 4H), 6.39 (d, 3J = 7.6 Hz, 2H), 6.33 (d, 2J = 13.7 Hz, 2H), 6.14 (t, 3J = 8.4 Hz, 2H), 6.07 (d, 2J = 13.7 Hz, 2H), 5.91 (d, 2J = 13.7 Hz, 2H), 5.82–5.73 (m, 6H), 5.58 (t, 3J = 8.4 Hz, 2H), 4.53–3.57 (m, 48H), 2.82 (d, 3J = 8.4 Hz, 2H), 2.52 (d, 3J = 8.4 Hz, 2H) ppm. 13C NMR (CD3CN, 125 MHz, 298 K): δ = 151.6, 150.2, 146.4, 146.1, 145.8, 145.0, 144.83, 144.79, 144.76, 144.8, 144.2, 143.4, 143.2, 142.3, 137.6, 136.6, 136.4, 136.3, 131.1, 131.0, 130.6, 128.4, 127.1, 126.8, 126.7, 126.2, 126.1, 125.8, 125.7, 124.5, 124.4, 124.3, 123.9, 108.5, 106.9, 104.8, 103.6, 71.0, 70.4, 69.1, 69.0, 68.9, 68.8, 68.7, 68.6, 68.4, 68.3, 67.7, 64.9, 64.8, 64.7, 60.8, 60.5 ppm. ESI-MS: calcd for [M − 2PF6]2+, m/z = 1322.3308, found: m/z = 1322.3307.
Results and discussion
The free ditopic host, DBB·8PF6, was prepared (Scheme 1) in 17% yield by a template-directed protocol, using 1,5-bis[2-(2-hydroxyethoxy)ethoxy]naphthalene (BHEEN) as a π-electron-rich template which can subsequently be removed from the host using liquid–liquid extraction techniques. The 1H NMR spectrum (Fig. 2) of DBB·8PF6 shows two signals each for both the protons α and β to the bipyridinium nitrogens as a result of its having D2 symmetry – i.e., three mutually perpendicular C2 axes through the central benzenoid ring on to which both CBPQT4+ cyclophanes are fused. This particular fused ditopic host displays planar chirality. This element of chirality is coincident with the plane containing the central 1,2,4,5-tetrasubstituted benzenoid ring. The stereogenic assignments to both enantiomers are shown in Fig. 3a: two assignments can be made to the molecule based on the two directions from which the plane of chirality can be viewed from the pilot atoms. The single-crystal X-ray structure22 of DBB·8PF6 reveals (Fig. 3b) that DBB·8PF6 crystallizes as a racemic modification with both enantiomers present in the asymmetric unit. In the case of the (RR)-enantiomer, the angle between the plane of the central benzenoid ring and one of the cyclophanes is 62°, while the angle between the other cyclophane and the central benzenoid ring is 56°. For the (SS)-enantiomer, these angles are 62° and 48°, respectively. In the case of both enantiomers, the angles represent a large deviation from the expected angle of 90°, possibly as a result of crystal packing. In the two fused cyclophanes, however, the torsional angle between the pyridinium rings in the four bipyridinium (BIPY2+) units are, for the (RR)-enantiomer, 42° and 25° in one of the cyclophanes, and 38° and 20° in the other cyclophane, whilst these angles for the (SS)-enantiomer are 28° and 24° in one of the cyclophanes, and 30° and 28° in the other cyclophane. Although it is possible to create two enantiomers of this ditopic host, this compound is topologically trivial since it is possible to represent (Fig. 3c) this molecule as possessing a planar graph23 – this ditopic host displays only Euclidean chirality.
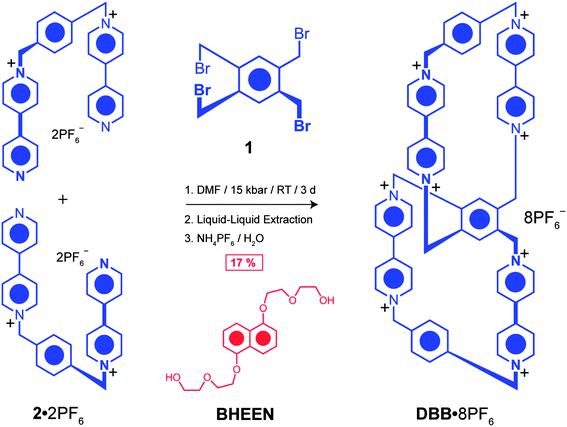 |
| Scheme 1 Synthesis of the free, fused ditopic host DBB·8PF6. | |
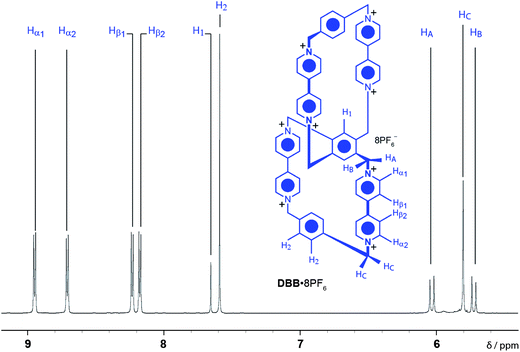 |
| Fig. 2
1H NMR Spectrum (CD3CN, 600 MHz, 298 K) of DBB·8PF6. | |
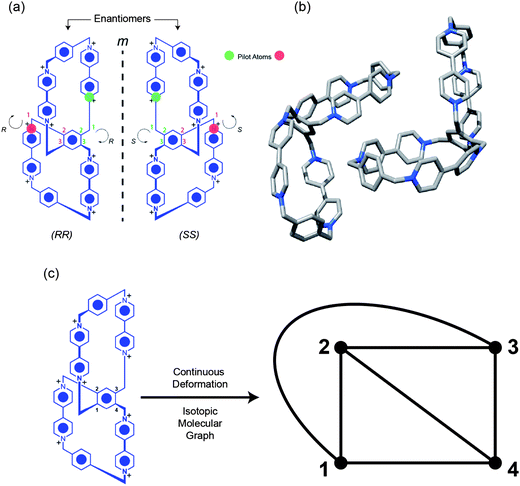 |
| Fig. 3 (a) Illustration of the enantiomers of DBB8+ as a result of the presence of a plane of chirality associated with the central benzenoid ring. The assignment of chirality is based on the priority of the carbon atoms contained within the central benzenoid unit when observed from the pilot atoms highlighted in red and green. (b) Asymmetric unit of the X-ray crystal structure of DBB8+, revealing co-crystallization of both enantiomers. (c) Continuous deformation shows that the ditopic host can be represented with a planar molecular graph. | |
Binding studies (see ESI†) carried out using isothermal titration calorimetry (ITC) with the ditopic host DBB·8PF6 and the guest BHEEN reveal that much weaker binding (K1 = 2047 ± 249 M−1, K2 = 52 ± 6 M−1) occurs between the host and guest in this system compared to that (Ka = 36
400 ± 300 M−1) involving CBPQT4+ and BHEEN.24 The reason for this significantly weaker binding is believed to arise from diminished [C–H⋯O] interactions between the host and the polyether loops in the guest molecules. The binding mechanism displays allosteric negative cooperativity25 (K2 < 0.25 × K1), an observation which is indicative of disfavored templation during the final SN2 reaction to form the ditopic host.
The crystal structure26 of (BHEEN)2⊂DBB·8PF6 reveals (see Fig. S17 in the ESI†) that, in the presence of two guest molecules, both CBPQT4+ cyclophanes are aligned so that they are as far apart as possible, the angle between the planes of the central benzenoid ring and the CBPQT4+ cyclophanes being 88°. The crystal structure of (BHEEN)2⊂DBB·8PF6 also reveals that a third molecule of BHEEN, which is not included within a CBPQT4+ cyclophane, interacts (see Fig. S17 in the ESI†) with [(BHEEN)2⊂DBB]8+ in a side-on manner, similar to what has been reported previously27 for host–guest inclusion complexes with CBPQT4+ and guests related to BHEEN. As is typically the case,18 the host–guest inclusion complex is also stabilized by [C–H⋯O] (3.02–3.42 Å) and [C–H⋯π] (3.35–3.42 Å) interactions. The torsional angles between the pyridinium rings in the four BIPY2+ units are significantly less than those present in the solid-state structure of free DBB8+: they are 11° for the BIPY2+ unit involved in an alongside interaction with the uncomplexed BHEEN molecule, and close to 20° in the case of the other three BIPY2+ units. We can relate this crystal structure to the weak binding of BHEEN to DBB8+ since we now know that there is no steric barrier to the binding of a second BHEEN, so it is possible to suggest that, upon binding of one BHEEN to DBB8+, the (BHEEN⊂DBB)8+ complex is much less electron-deficient than the vacant ditopic host, and therefore less willing to accept another electron-rich guest. We also note that one of the terminal oxygen atoms in both of the included BHEEN molecules in the solid-state superstructure of [(BHEEN)2⊂DBB]8+ are directed towards the “ortho regions” (ESI, Fig. S17†) of the ditopic host, with [C–H⋯O] interactions between the methylene carbon atoms and the terminal BHEEN oxygen atoms characterized by [C⋯O] distances ranging from 3.22–3.45 Å.
Given the nature of this weak binding, it is not difficult to comprehend why the yield of DBB·8PF6 is relatively low compared to that of CBPQT4+, when using BHEEN as a template. This observation suggests that the more favored compound is the substituted CBPQT4+ cyclophane, and the remaining bromomethyl groups are free to react with residual 2·2PF6 to form a range of oligomers. Increases in yields are expected through the use of guests having stronger binding affinities towards the ditopic host, such as guests containing tetrathiafulvalene derivatives.24 These binding studies have important consequences for the formation of the handcuff catenane HC·8PF6;28 most notably, it is unlikely that this topology will be the most favored product.
Inspection of molecular models, supported by computer modeling employing molecular mechanics,29 (Fig. 4) establishes that the polyether loops in DN50C14 are sufficiently long to permit both 1,5-dihydroxynaphthalene (DNP) units to act as templates, with one DNP unit ending up being situated in one of the CBPQT4+ cyclophanes, while the other DNP unit finds itself being located in the other CBPQT4+ cyclophane of the handcuff catenane HC8+. Furthermore, modeling reveals that it is possible for the polyether loops to wind their way around the central 1,2,4,5-tetrasubstituted benzenoid ring in two distinctly different ways – one in which (i) the polyether loops traverse the “ortho regions” between C-1 and C-2, and C-4 and C-5, on the benzenoid ring, and the other in which (ii) the polyether loops traverse the “meta regions” defined by C-3 and C-6 on the benzenoid ring. We will refer to the first of these two topologies as the ortho–ortho isomer and the second one as the meta–meta isomer (Fig. 5). It should be noted that these two topological isomers cannot interconvert without the breaking and making of a covalent bond in either DN50C14 or one of the two cyclophanes.
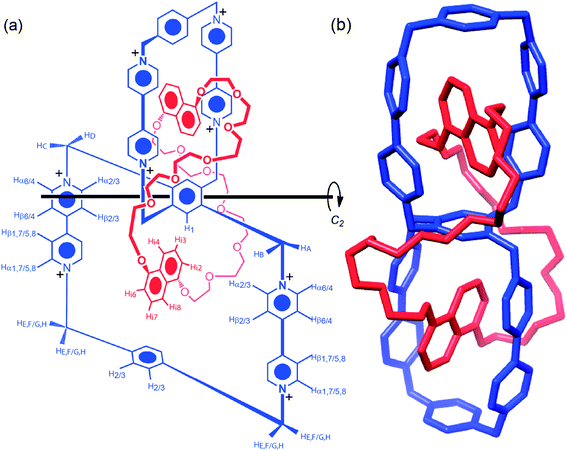 |
| Fig. 4 Structural formula (a), and MMFF94 minimized structure (b) of HC8+. Protons on the structural formula are labeled in keeping with their corresponding 1H NMR assignments in Fig. 7. A C2 axis, through the central benzenoid ring of the ditopic host, relates both CBPQT4+/DNP host–guest systems. | |
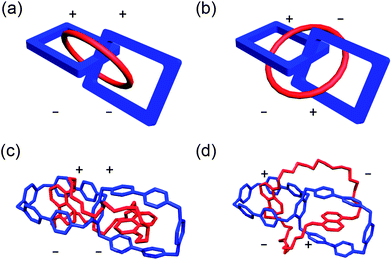 |
| Fig. 5 Generic topologies (a and b) and the corresponding DFT-optimized structures ((c and d), respectively) of the lowest energy topological isomers of HC8+. Structures (a and c) correspond to the major topology observed by single-crystal X-ray diffraction. Positive (+) and negative (−) symbols indicate over and under crossings, respectively. | |
The template-directed synthesis (Scheme 2) of the handcuff catenane HC·8PF6 was achieved in one step using 1,2,4,5-tetra(bromomethyl)benzene (1), 1,1′-[1,4-phenylenebis-methylene]bis(4,4′-bipyridinium)-bis(hexafluorophosphate) (2·2PF6), and DN50C14,18 under ultra-high pressure conditions (15 kbar), followed by purification using reverse-phase high performance liquid chromatography (RP-HPLC) and counterion exchange. The yield30 (7.4%), obtained employing this synthetic protocol is low since, unlike most other catenations involving π-electron rich crown ethers and the CBPQT4+ cyclophane,7f there are no alongside interactions to stabilize the transition states associated with the final SN2 substitutions leading to the formation of the CBPQT4+ cyclophane. This approach to the template-directed synthesis of HC·8PF6, where a macrocyclic polyether is used to template the formation of a ditopic host, differs from the protocols reported for the preparation of the other handcuff catenanes where a bis-macrocycle is prepared in the first instance to be followed subsequently by the threading of two guests8b,c,9b or a ditopic guest13 to form a pseudorotaxane, from where the desired topology is obtained after the ring-closing steps.
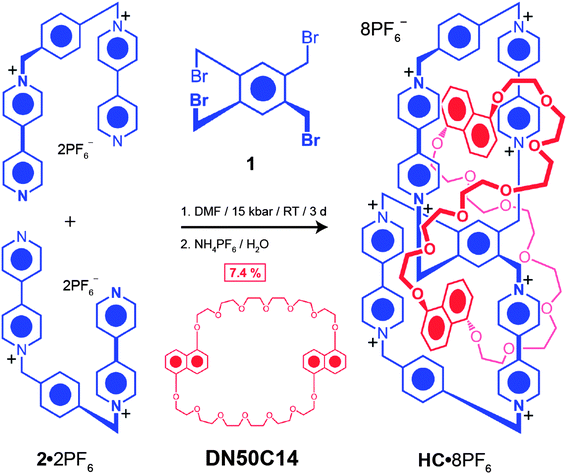 |
| Scheme 2 Synthesis of the handcuff catenane HC·8PF6. | |
Single-crystals suitable for X-ray diffraction31 were grown by vapor diffusion of i-Pr2O into a solution of HC·8PF6 in MeCN. The solid-state structure (Fig. 6) of HC8+ reveals a structure similar to that obtained by molecular modeling with the polyether loops linking the DNP units occupying the “meta regions” around the central benzenoid ring – i.e., HC8+ exists32 as the meta–meta isomer in the solid state. The location of the polyether loops in the “meta regions” around the fused cyclophanes within the solid-state structure of HC8+ is different from those (see Fig. S17 in the ESI†) observed within [(BHEEN)2⊂DBB]8+, where the polyether chains are oriented towards the “ortho regions” of the fused cyclophanes. Analysis of the solid-state structure of HC8+, which exhibits C2 symmetry, reveals numerous [C–H⋯O] interactions33 between (i) protons α and β to the nitrogens in the bipyridinium units, as well as (ii) protons located on the central benzenoid ring and polyether loop oxygen atoms. In addition, the DNP units enter into weak [C–H⋯π] interactions34 with the para-xylylene linkers and the central benzenoid ring. The torsional angles between adjacent pyridinium rings in the two different BIPY2+ units differ significantly in the two equivalent fused cyclophanes: on one side of the cyclophane, the torsional angle is 16° while, on the other side, it is such (3°) that the two pyridinium rings are almost coplanar.
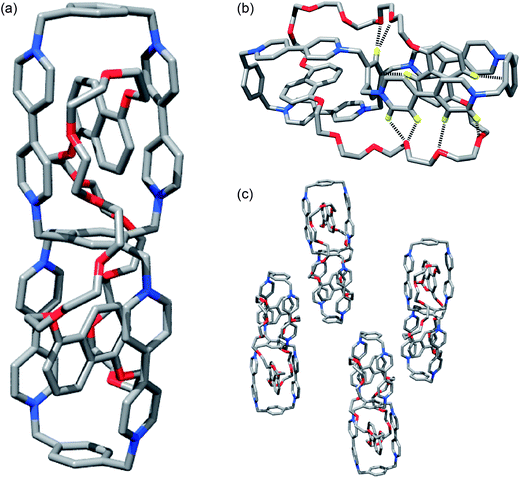 |
| Fig. 6 Alternative views (a and b) of the single-crystal X-ray structure of one enantiomer of HC8+. Yellow hydrogen atoms and dashed lines (b) represent [C–H⋯O] interactions with [C⋯O] distances ranging of 3.04–3.33 Å and [C–H⋯π] interactions (3.40 Å). Packing view (c) reveals the co-crystallization of both enantiomers of HC8+. | |
The 1H NMR spectrum (Fig. 7) of HC·8PF6 recorded in CD3CN can be interpreted in terms of the handcuff catenane adopting averaged C2 symmetry overall with local C2h symmetry being preserved inside both of the cyclophanes imposed by the entrapped DNP units that are not undergoing concerted degenerate inversion on the 1H NMR timescale at room temperature. The outcome is that (i) eight resonances (δ = 9.11, 9.07, 9.07, 8.95, 8.88, 8.73, 8.73, 8.69 ppm) are observed for the protons α to the nitrogens and (ii) eight resonances (δ = 7.74, 7.71, 7.68, 7.47, 7.47, 7.47, 7.40, 7.28 ppm) are observed for the protons β to the nitrogens on the bipyridinium units, while (iii) four resonances (δ = 6.66, 6.46, 6.33, 6.07 ppm) are observed for the protons on the methylene groups attached to the 1, 2, 4, 5 positions on the central benzenoid ring with its (iv) single resonance (a singlet) appearing at δ = 8.98 ppm. The DNP units appear in the 1H NMR spectrum recorded in CD3CN at room temperature as (i) two resonances (δ = 6.39 and 6.46 ppm) for Hi2 and Hi6 respectively, (ii) two resonances (δ = 5.58 and 6.14 ppm) for Hi3 and Hi7 respectively, and (iii) two resonances (δ = 2.82 and 2.52 ppm) for Hi4 and Hi8, respectively. When the 1H NMR spectrum (Fig. S13†) is recorded in CD3SOCD3 at high temperatures (298–363 K), these pairs of resonances coalesce35 in keeping with the concerted degenerate DNP inversion process (Fig. 8) becoming rapid on the 1H NMR timescale. The free energy (ΔG‡) of activation for this process is associated with a ΔGc‡ value36 of ca. 16 kcal mol−1. It is accompanied by the rotation of the bipyridinium units37 in HC8+ becoming fast on the 1H NMR timescale as well.
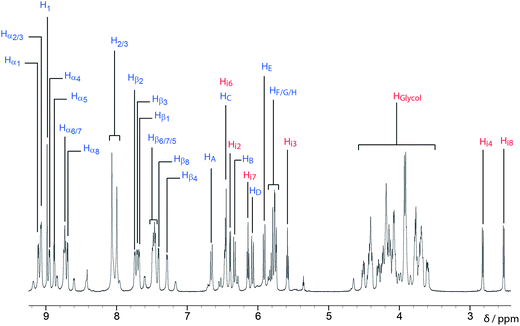 |
| Fig. 7
1H NMR spectrum (CD3CN, 600 MHz, 298 K) of HC·8PF6. Protons have been assigned in keeping with the labels on the structural formula in Fig. 4a. | |
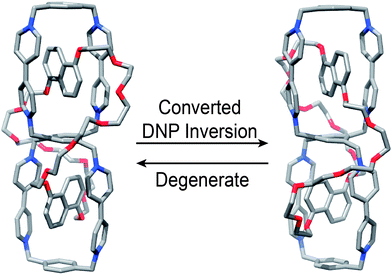 |
| Fig. 8 Degenerate co-conformations of HC8+. Upon concerted DNP reorientation, the resulting structure is related to the original one by a 180° rotation around one of two possible axes. | |
A second, minor species is also observed in the 1H NMR spectrum of HC·8PF6. Upon examination of a 2D 1H EXSY spectrum and variable-temperature (VT) 1H NMR spectra (see ESI†), it becomes evident that (i) the protons in this minor species do not undergo exchange, and (ii) the resonances of the major and minor species do not coalesce. After numerous attempts32 to remove this minor species from the product, including both fractional crystallization and recycling RP-HPLC, no separation of it from the major species could be achieved. This outcome suggests that the minor species is very similar in both structure and charge to the major species. 2D 1H DOSY NMR spectroscopy (see ESI†) confirms this hypothesis, revealing that the major and minor species have similar diffusion properties in solution. We believe that these major and minor products are in fact topological isomers, and can be visualized by considering the nature of the crossing points in both isomers38 (Fig. 5). Given that the meta–meta isomer is observed in the solid-state by single-crystal X-ray diffraction (Fig. 6), we presume that this isomer is the major product present (Fig. 7) in solution by 1H NMR spectroscopy.
Finally, DFT calculations were employed in order to shed some light on the relative stabilities of each isomer. For each isomer, we considered the two co-conformations arising from different DNP orientations, leading to a total of four structures of HC8+. The structures were optimized at the B3LYP-D3/6-31G** level.39 Single-point calculations with the larger 6-311G**++ basis set and with two different continuum solvation models were then carried out. In vacuum, the ortho–ortho isomer is favored by ca. 2.4 kcal mol−1, while solvation favors the meta–meta isomer observed experimentally (see Table S1†). Upon the addition of solvation using a continuum Poisson–Boltzmann40 (PBF) or SM8 (ref. 41) solvation model in DMF, the proposed meta–meta isomer is predicted to be more energetically favorable (see ESI†) by 5.9 and 2.4 kcal mol−1 for the PBF and SM8 models, respectively, suggesting that, upon solvation, the DFT calculations match the situation which is observed experimentally. These DFT calculations indicate that the proposed structure for the minor topology is indeed a reasonable candidate to account for the ortho–ortho isomer observed experimentally. The relative alignment of the DNP units inside the fused cyclophanes of the lowest energy co-conformation of the ortho–ortho isomer is opposite to that observed for the meta–meta isomer, hence the C2 axis of symmetry of the lowest energy minor isomer is perpendicular to, yet lying in the same plane as, that of the major isomer. Additionally, the calculated relative energies of the co-conformations adopted by the DNP units in the meta–meta isomer reveal that the orientations of the DNP units observed in the solid-state are considerably lower in energy – both in vacuum and in DMF – compared with the alternative relative orientations of the DNP units (see ESI†). This observation is in agreement with the 1H NMR spectroscopic evidence. Fig. 9 illustrates the different enantiomers and diastereoisomers of the handcuff catenane.
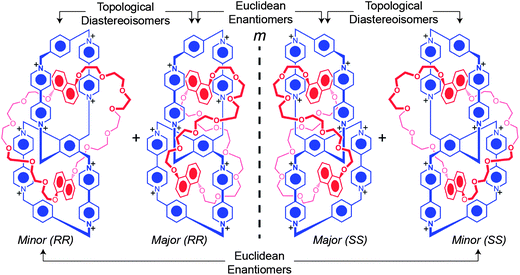 |
| Fig. 9 Illustration of the ortho–ortho (minor) and meta–meta (major) isomers of HC8+ and their stereochemical relationships to one another. | |
Conclusions
It is possible to use a template-directed protocol to access a donor–acceptor handcuff catenane with a couple of distinctive topologies that cannot be interconverted without the breaking and making of at least one covalent bond. The fact that one topological isomer is very much more abundant than the other isomer in the reaction mixture makes it difficult to isolate the minor isomer with ortho–ortho stereochemistry from the major one with meta–meta stereochemistry. Since the crown ether containing two 1,5,dioxynaphthalene units, which act as the template for the formation of two fused tetracationic cyclophanes, is capable of undergoing relative motion of a degenerate nature on the 1H NMR timescale, it should be possible to design and construct molecular switches based on the handcuff catenane topology.
Acknowledgements
The authors thank our joint collaborators Dr Turki S. Al-Saud and Dr Nezar H. Khdary from the King Abdulaziz City of Science and Technology (KACST) in Saudi Arabia for their interest in this research. A.K.B. acknowledges Fulbright New Zealand for a Fulbright Graduate Award and the New Zealand Federation of Graduate Women for a Postgraduate Fellowship award. S.T.S. thanks the International Institute for Nanotechnology (IIN) at Northwestern University (NU) for a postdoctoral fellowship and the QUEST high-performance computing center at NU. D.C. acknowledges the National Science Foundation (NSF) for a Graduate Research Fellowship and the IIN at Northwestern University for a Ryan Fellowship.
Notes and references
-
(a)
G. Schill, Catenanes, Rotaxanes and Knots, Academic Press, New York, 1971 Search PubMed;
(b) C. O. Dietrich-Buchecker, J.-P. Sauvage and J.-P. Kintzinger, Tetrahedron Lett., 1983, 24, 5095–5098 CrossRef CAS;
(c) C. O. Dietrich-Buchecker, J.-P. Sauvage and J.-P. Kintzinger, J. Am. Chem. Soc., 1984, 106, 3043–3045 CrossRef CAS;
(d) M. Cesario, C. O. Dietrich-Buchecker, J. Guilhem, C. Pascard and J.-P. Sauvage, J. Chem. Soc., Chem. Commun., 1985, 244–247 RSC;
(e) J.-P. Sauvage and J. Weiss, J. Am. Chem. Soc., 1985, 107, 6108–6110 CrossRef CAS;
(f) P. R. Ashton, T. T. Goodnow, A. E. Kaifer, M. V. Reddington, A. M. Z. Slawin, N. Spencer, J. F. Stoddart, C. Vicent and D. J. Williams, Angew. Chem., Int. Ed., 1989, 28, 1396–1399 CrossRef;
(g) D. H. Busch and N. A. Stephenson, Coord. Chem. Rev., 1990, 100, 119–154 CrossRef CAS;
(h) C. Dietrich-Buchecker, B. Frommberger, I. Lüer, J.-P. Sauvage and F. Vögtle, Angew. Chem., Int. Ed., 1993, 32, 1434–1437 CrossRef;
(i) M. B. Nielsen, C. Lomholt and J. Becher, Chem. Soc. Rev., 2000, 29, 153–164 RSC;
(j) T. J. Hubin and D. H. Busch, Coord. Chem. Rev., 2000, 200–202, 5–52 CrossRef CAS;
(k) O. Š. Miljanić and J. F. Stoddart, Proc. Natl. Acad. Sci. U. S. A., 2007, 104, 12966–12970 CrossRef PubMed;
(l) C. J. Bruns and J. F. Stoddart, Top. Curr. Chem., 2012, 323, 19–72 CrossRef CAS PubMed;
(m) S. P. Black, A. R. Stefankiewicz, M. M. J. Smulders, D. Sattler, C. A. Schalley, J. R. Nitschke and J. K. M. Sanders, Angew. Chem., Int. Ed., 2013, 52, 5749–5753 CrossRef CAS PubMed;
(n) J. E. Beves, C. J. Campbell, D. A. Leigh and R. G. Pritchard, Angew. Chem., Int. Ed., 2013, 52, 6464–6467 CrossRef CAS PubMed.
-
(a) A. Coskun, M. Banaszak, R. D. Astumian, J. F. Stoddart and B. A. Grzybowski, Chem. Soc. Rev., 2012, 41, 19–30 RSC;
(b) B. Lewandowski, G. De Bo, J. W. Ward, M. Papmeyer, S. Kuschel, M. J. Aldegunde, P. M. E. Gramlich, D. Heckmann, S. M. Goldup, D. M. D'Souza, A. E. Fernandes and D. A. Leigh, Science, 2013, 339, 189–193 CrossRef CAS PubMed.
-
(a) R. A. Bissell, E. Córdova, A. E. Kaifer and J. F. Stoddart, Nature, 1994, 369, 133–137 CrossRef CAS;
(b) M. Asakawa, P. R. Ashton, V. Balzani, A. Credi, C. Hamers, G. Mattersteig, M. Montalti, A. N. Shipway, N. Spencer, J. F. Stoddart, M. S. Tolley, M. Venturi, A. J. P. White and D. J. Williams, Angew. Chem., Int. Ed., 1998, 37, 333–337 CrossRef CAS;
(c) E. R. Kay, D. A. Leigh and F. Zerbetto, Angew. Chem., Int. Ed., 2007, 46, 72–191 CrossRef CAS PubMed;
(d) D. Canevet, M. Salle, G. Zhang, D. Zhang and D. Zhu, Chem. Commun., 2009, 2245–2269 RSC;
(e) N. H. Evans, C. J. Serpell and P. D. Beer, Chem.–Eur. J., 2011, 17, 7734–7738 CrossRef CAS PubMed;
(f) A. C. Fahrenbach, C. J. Bruns, D. Cao and J. F. Stoddart, Acc. Chem. Res., 2012, 45, 1581–1592 CrossRef CAS PubMed.
-
(a) C. P. Collier, G. Mattersteig, E. W. Wong, Y. Luo, K. Beverly, J. Sampaio, F. M. Raymo, J. F. Stoddart and J. R. Heath, Science, 2000, 289, 1172–1175 CrossRef CAS PubMed;
(b) J. E. Green, J. W. Choi, A. Boukai, Y. Bunimovich, E. Johnston-Halperin, E. DeIonno, Y. Luo, B. A. Sheriff, K. Xu, Y. Shik Shin, H.-R. Tseng, J. F. Stoddart and J. R. Heath, Nature, 2007, 445, 414–417 CrossRef CAS PubMed;
(c) A. Coskun, J. M. Spruell, G. Barin, W. R. Dichtel, A. H. Flood, Y. Y. Botros and J. F. Stoddart, Chem. Soc. Rev., 2012, 41, 4827–4859 RSC.
-
(a) E. Wasserman, J. Am. Chem. Soc., 1960, 82, 4433–4434 CrossRef CAS;
(b) R. Wolovsky, J. Am. Chem. Soc., 1970, 92, 2132–2133 CrossRef CAS;
(c) I. T. Harrison, J. Chem. Soc., Perkin Trans. 1, 1974, 301–304 RSC.
- G. Schill and A. Lüttringhaus, Angew. Chem., Int. Ed., 1964, 3, 546–547 CrossRef.
-
(a) M. R. Bryce, J. Mater. Chem., 2000, 10, 589–598 RSC;
(b) J. F. Stoddart and H. M. Colquhoun, Tetrahedron, 2008, 64, 8231–8263 CrossRef CAS;
(c) H. Y. Au-Yeung, G. D. Pantoş and J. K. M. Sanders, Proc. Natl. Acad. Sci. U. S. A., 2009, 106, 10466–10470 CrossRef CAS PubMed;
(d) J. F. Stoddart, Chem. Soc. Rev., 2009, 38, 1802–1820 RSC;
(e) H. Y. Au-Yeung, G. D. Pantoş and J. K. M. Sanders, Angew. Chem., Int. Ed., 2010, 49, 5331–5334 CrossRef CAS PubMed;
(f) A. C. Fahrenbach, K. J. Hartlieb, A. C.-H. Sue, C. J. Bruns, G. Barin, S. Basu, M. A. Olson, Y. Y. Botros, A. Bagabas, N. H. Khdary and J. F. Stoddart, Chem. Commun., 2012, 48, 9141–9143 RSC.
-
(a) K. S. Chichak, S. J. Cantrill, A. R. Pease, S.-H. Chiu, G. W. V. Cave, J. L. Atwood and J. F. Stoddart, Science, 2004, 304, 1308–1312 CrossRef CAS PubMed;
(b) J. Frey, T. Kraus, V. Heitz and J.-P. Sauvage, Chem. Commun., 2005, 5310–5312 RSC;
(c) J. Frey, T. Kraus, V. Heitz and J.-P. Sauvage, Chem.–Eur. J., 2007, 13, 7584–7594 CrossRef CAS PubMed;
(d) J. F. Stoddart, Chem. Soc. Rev., 2009, 38, 1521–1529 RSC;
(e) J. J. Henkelis, T. K. Ronson, L. P. Harding and M. J. Hardie, Chem. Commun., 2011, 47, 6560–6562 RSC.
-
(a) D. Curiel and P. D. Beer, Chem. Commun., 2005, 1909–1911 RSC;
(b) N. H. Evans, C. J. Serpell and P. D. Beer, Angew. Chem., Int. Ed., 2011, 50, 2507–2510 CrossRef CAS PubMed.
-
(a) C. A. Hunter, J. Am. Chem. Soc., 1992, 114, 5303–5311 CrossRef CAS;
(b) F. Vögtle, S. Meier and R. Hoss, Angew. Chem., Int. Ed., 1992, 31, 1619–1622 CrossRef;
(c) A. G. Johnston, D. A. Leigh, R. J. Pritchard and M. D. Deegan, Angew. Chem., Int. Ed., 1995, 34, 1209–1212 CrossRef CAS;
(d) M. E. Belowich, C. Valente, R. A. Smaldone, D. C. Friedman, J. Thiel, L. Cronin and J. F. Stoddart, J. Am. Chem. Soc., 2012, 134, 5243–5261 CrossRef CAS PubMed;
(e) C. Ke, R. A. Smaldone, T. Kikuchi, H. Li, A. P. Davis and J. F. Stoddart, Angew. Chem., Int. Ed., 2013, 52, 381–387 CrossRef CAS PubMed.
-
(a) A. Harada, J. Li and M. Kamachi, Nature, 1992, 356, 325–327 CrossRef CAS;
(b) M. Fujita, F. Ibukuro, H. Hagihara and K. Ogura, Nature, 1994, 367, 720–723 CrossRef CAS.
-
(a) H. Li, A. C. Fahrenbach, S. K. Dey, S. Basu, A. Trabolsi, Z. Zhu, Y. Y. Botros and J. F. Stoddart, Angew. Chem., Int. Ed., 2010, 49, 8260–8265 CrossRef CAS PubMed;
(b) A. Trabolsi, N. Khashab, A. C. Fahrenbach, D. C. Friedman, M. T. Colvin, K. K. Cotí, D. Benítez, E. Tkatchouk, J.-C. Olsen, M. E. Belowich, R. Carmieli, H. A. Khatib, W. A. Goddard, M. R. Wasielewski and J. F. Stoddart, Nat. Chem., 2010, 2, 42–49 CrossRef CAS PubMed;
(c) J. C. Barnes, A. C. Fahrenbach, D. Cao, S. M. Dyar, M. Frasconi, M. A. Giesener, D. Benítez, E. Tkatchouk, O. Chernyashevskyy, W. H. Shin, H. Li, S. Sampath, C. L. Stern, A. A. Sarjeant, K. J. Hartlieb, Z. Liu, R. Carmieli, Y. Y. Botros, J. W. Choi, A. M. Z. Slawin, J. B. Ketterson, M. R. Wasielewski, W. A. Goddard III and J. F. Stoddart, Science, 2013, 339, 429–433 CrossRef CAS PubMed.
- Z.-T. Li and J. Becher, Chem. Commun., 1996, 639–640 RSC.
- R. S. Forgan, J.-P. Sauvage and J. F. Stoddart, Chem. Rev., 2011, 111, 5434–5464 CrossRef CAS PubMed.
-
(a) D. M. Walba, Tetrahedron, 1985, 41, 3161–3212 CrossRef CAS;
(b) J.-F. Nierengarten, C. O. Dietrich-Buchecker and J.-P. Sauvage, J. Am. Chem. Soc., 1994, 116, 375–376 CrossRef CAS.
- D. K. Mitchell and J.-P. Sauvage, Angew. Chem., Int. Ed., 1988, 27, 930–931 CrossRef.
- Although species containing multiple covalently attached CBPQT4+ hosts have been reported, these compounds are typically prepared using coupling techniques, such as Cu-mediated azide–alkyne cycloaddition (CuAAC), rather than multiple SN2 reactions between a benzyl halide and pyridine nitrogen, as was utilized in this approach, to enable the preparation of a rigid ditopic host. For examples of coupling reactions between CBPQT4+ cyclophanes, see:
(a) R. Klajn, M. A. Olson, P. J. Wesson, L. Fang, A. Coskun, A. Trabolsi, S. Soh, J. F. Stoddart and B. A. Grzybowski, Nat. Chem., 2009, 1, 733–738 CrossRef CAS PubMed;
(b) M. A. Olson, A. Coskun, R. Klajn, L. Fang, S. K. Dey, K. P. Browne, B. A. Grzybowski and J. F. Stoddart, Nano Lett., 2009, 9, 3185–3190 CrossRef CAS PubMed.
-
DN50C14 has been shown previously (see: R. S. Forgan, D. C. Friedman, C. L. Stern, C. J. Bruns and J. F. Stoddart, Chem. Commun., 2010, 46, 5861–5863 RSC ) to facilitate the formation of ring-in-ring complexes as well as template the formation of CBPQT4+ cyclophanes independently around each of its two DNP units, leading to the production of a [3]catenane which has been employed subsequently in the template-directed synthesis of a [2]pseudorota[3]catenane. See: R. S. Forgan, J. J. Gassensmith, D. B. Cordes, M. M. Boyle, K. J. Hartlieb, D. C. Friedman, A. M. Z. Slawin and J. F. Stoddart, J. Am. Chem. Soc., 2012, 134, 17007–17010 CrossRef CAS PubMed.
- P. R. Ashton, A. S. Reder, N. Spencer and J. F. Stoddart, J. Am. Chem. Soc., 1993, 115, 5286–5287 CrossRef CAS.
- P.-L. Anelli, P. R. Ashton, R. Ballardini, V. Balzani, M. Delgado, M. T. Gandolfi, T. T. Goodnow, A. E. Kaifer, D. Philp, M. Pietraszkiewicz, L. Prodi, M. V. Reddington, A. M. Z. Slawin, N. Spencer, J. F. Stoddart, C. Vicent and D. J. Williams, J. Am. Chem. Soc., 1992, 114, 193–218 CrossRef CAS.
- C. L. Brown, D. Philp, N. Spencer and J. F. Stoddart, Isr. J. Chem., 1992, 32, 61–67 CrossRef CAS.
- ESI.†.
- J.-C. Chambron, C. Dietrich-Buchecker and J.-P. Sauvage, Top. Curr. Chem., 1993, 165, 131–162 CrossRef CAS.
- J. W. Choi, A. H. Flood, D. W. Steuerman, S. Nygaard, A. B. Braunschweig, N. N. P. Moonen, B. W. Laursen, Y. Luo, E. DeIonno, A. J. Peters, J. O. Jeppesen, K. Xu, J. F. Stoddart and J. R. Heath, Chem.–Eur. J., 2006, 12, 261–279 CrossRef CAS PubMed.
- P. Thordarson, Chem. Soc. Rev., 2011, 40, 1305–1323 RSC.
- ESI.†.
- M. Asakawa, W. Dehaen, G. L'Abbé, S. Menzer, J. Nouwen, F. M. Raymo, J. F. Stoddart and D. J. Williams, J. Org. Chem., 1996, 61, 9591–9595 CrossRef CAS.
- A catenation was attempted with one equivalent of bisparaphenylene[46]crown-14 (see: D. B. Amabilino, P. R. Ashton, C. L. Brown, E. Córdova, L. A. Godinez, T. T. Goodnow, A. E. Kaifer, S. P. Newton and M. Pietraszkiewicz, J. Am. Chem. Soc., 1995, 117, 1271–1293 CrossRef CAS ) i.e., a crown ether with fewer π-electron-rich aromatic units. The handcuff catenane, however, was not formed, even under high pressure conditions, most likely on account of the inability of this crown ether to template the formation of the fused ditopic host. The handcuff catenane HC8+ incorporating DN50C14 is only formed under high-pressure reaction conditions: attempts to obtain it employing a high-dilution reaction protocol were not at all successful, suggesting to us that chelate cooperativity does not play a significant role in the formation of the handcuff catenane.
- Molecular mechanics modeling was performed using the MMFF94 forcefield in Avogadro: an open-source molecular builder and visualization tool. Version 1.0.3; see M. D. Hanwell, D. E. Curtis, D. C. Lonie, T. Vandermeersch, E. Zurek and G. R. Hutchison, J. Cheminf., 2012, 4, 17.
- In principle, other products incorporating DN50C14 might also be expected to be formed using this synthetic protocol – namely, a [2]-catenane consisting of a DN50C14 ring threaded through one cyclophane, leaving the other cyclophane empty, in addition to a bis[2]catenane similar to that reported previously. See ref. 19. Both HPLC and mass spectrometry indicate that the bis[2]catenane is present in the product mixture, whilst no trace of a [2]-catenane could be found, most likely because of a lack of an acyclic electron-rich compound to template the formation of the second cyclophane.
- ESI.†.
- After numerous unsuccessful attempts to effect fractional crystallization of the ortho–ortho isomer, we have come to the conclusion that the two topological isomers co-crystallize. This realization is a consequence of recording the 1H NMR spectra following dissolution of a number of different batches of crystals, only to discover that the ortho–ortho isomer is always present as a minor component along with the meta–meta isomer in CD3CN solutions.
- [C–H⋯O] Interactions between the protons α and β to the BIPY2+ nitrogen atoms and the oxygen atoms in the polyether loops vary between 3.02–3.33 Å ([C⋯O] distances), while very much weaker [C–H⋯O] interactions are present between protons on the central benzenoid ring and the oxygen atoms of the polyether loop, with a [C⋯O] distance of 3.44 Å observed in the solid state. See: F. M. Raymo, M. D. Bartberger, K. N. Houk and J. F. Stoddart, J. Am. Chem. Soc., 2001, 123, 9264–9267.
- Weak [C–H⋯π]
interactions are operative between Hi4 and the central benzenoid ring, as well as between Hi8 and the terminal para-xylylene linkers, with a [C⋯π] distance of 3.40 Å being operative in both cases.
- Rate constants, kc, for the DNP inversion process were determined by dynamic 1H NMR spectroscopy in CD3SOCD3. The Eyring equation, ΔGc‡ = –RT
ln(kch/kBTc) was used to calculate ΔGc‡ values at the coalescence temperatures, Tc. For Hi4/8: Δν = 197 Hz, kc = 438 s−1, Tc = 343 K, ΔGc‡ = 16.0 kcal mol−1; for Hi3/7: Δν = 276 Hz, kc = 614 s−1, Tc = 343 K, ΔGc‡ = 15.8 kcal mol−1. See: I. O. Sutherland, Annu. Rep. NMR Spectrosc., 1971, 4, 71–235 CrossRef CAS.
- By comparison, DNP inversion in a [2]catenane consisting of a CBPQT4+ cyclophane and 1,5-dinaptho[38]crown-10 occurs with a ΔGc‡ value of 17.2 kcal mol−1. See: P. R. Ashton, C. L. Brown, E. J. T. Chrystal, T. T. Goodnow, A. E. Kaifer, K. P. Parry, D. Philp, A. M. Z. Slawin, N. Spencer, J. F. Stoddart and D. J. Williams, J. Chem. Soc., Chem. Commun., 1991, 634–639 RSC.
- At elevated temperature, the eight resonances each for protons α and β to the bipyridinium nitrogens coalesce to give only two signals for both the α and β protons—similar to that observed for DBB·8PF6 at room temperature—as a result of (i) a loss of the local C2h symmetry from fast DNP inversion and (ii) rapid bipyridinium rotation on the 1H NMR timescale. See: S. A. Vignon and J. F. Stoddart, Collect. Czech. Chem. Commun., 2005, 70, 1493–1576 CrossRef CAS.
- In an attempt to prevent the formation of one of the topological isomers, a smaller electron-rich crown ether, bis-1,5-dioxynaphthalene[44]crown-12, was prepared (see J.-Y. Ortholand, A. M. Z. Slawin, N. Spencer, J. F. Stoddart and D. J. Williams, Angew. Chem., Int. Ed., 1989, 28, 1394–1395 CrossRef ) to replace DN50C14. Although it was expected that the pentaethylene glycol chains separating the two DNP units would be sufficiently long to permit formation of only one topological isomer of the handcuff catenane, no trace of the handcuff catenane was observed by RP-HPLC, suggesting that DN50C14 is the smallest symmetrical DNP-based crown ether that is capable of templating handcuff catenanation involving two fused π-electron-deficient cyclobis(paraquat-p-phenylene) cyclophanes.
-
(a) A. D. Becke, J. Chem. Phys., 1993, 98, 5648–5652 CrossRef CAS;
(b) C. T. Lee, W. T. Yang and R. G. Parr, Phys. Rev. B: Condens. Matter Mater. Phys., 1988, 37, 785–789 CrossRef CAS;
(c) P. J. Stephens, F. J. Devlin, C. F. Chabalowski and M. J. Frisch, J. Phys. Chem., 1994, 98, 11623–11627 CrossRef CAS;
(d) S. H. Vosko, L. Wilk and M. Nusair, Can. J. Phys., 1980, 58, 1200–1211 CrossRef CAS;
(e) S. Grimme, J. Antony, S. Ehrlich and H. Krieg, J. Chem. Phys., 2010, 132, 154104 CrossRef PubMed.
-
Jaguar, Version 7.9, Schrödinger, LLC, New York (USA), 2012 Search PubMed.
-
(a) A. V. Marenich, R. M. Olson, C. P. Kelly, C. J. Cramer and D. G. Truhlar, J. Chem. Theory Comput., 2007, 3, 2011–2033 CrossRef CAS;
(b) R. M. Olson, A. V. Marenich, C. J. Cramer and D. G. Truhlar, J. Chem. Theory Comput., 2007, 3, 2046–2054 CrossRef CAS.
Footnote |
† Electronic supplementary information (ESI) available. CCDC 915343–915345. For ESI and crystallographic data in CIF or other electronic format see DOI: 10.1039/c3sc52106k |
|
This journal is © The Royal Society of Chemistry 2014 |
Click here to see how this site uses Cookies. View our privacy policy here.