DOI:
10.1039/D4VA00034J
(Critical Review)
Environ. Sci.: Adv., 2024, Advance Article
Cross-cutting research and future directions under the GAPS networks†
Received
2nd February 2024
, Accepted 8th April 2024
First published on 16th April 2024
Abstract
The Global Atmospheric Passive Sampling (GAPS) network and GAPS Megacities network (GAPS-MC) are the only global-scale air monitoring programs for persistent organic pollutants (POPs), which support the Global Monitoring Plan (GMP) of the Stockholm Convention on POPs. The GAPS data represents all five United Nation regions and informs the spatial and temporal trends of listed POPs, their long-range transport in air, and new priorities for POPs monitoring and research. This information contributes to the Effectiveness Evaluation of the Stockholm Convention, which is assessed every six years. To ensure its long-term sustainability and relevance, the GAPS network is engaging in cross-cutting studies across fields of science and policy – leading to more holistic and integrative work on air pollution, health, climate science, and biodiversity. Future work under GAPS will continue to advance areas of intersection and tap-into topics and expertise in areas such as non-target analysis (chemical mixture approaches including transformation products in air), advanced data analysis and land-use assessment methods, top-down and inverse global modeling, global air health assessment/warning systems, and citizen science and outreach.
Environmental significance
Environmental Significance Statement for “Cross-cutting Research and Future Directions under the GAPS networks” by Tom Harner, Amandeep Saini, Pourya Shahpoury, Anita Eng, Jasmin Schuster, Egide Kalisa and Jacob Mastin. The manuscript is a review of developments and applications in research and monitoring under the GAPS global passive air sampling networks, which include the core GAPS network operating since 2005 at ∼60 sites, and the GAPS Megacities network, operating since 2018 at 23 sites. The paper describes new areas of study beyond assessing persistent organic pollutants (POPs) in the global atmosphere – now also targeting broad areas of chemical pollution, health, climate change and biodiversity loss. Future challenges and priorities are also discussed.
|
Introduction
The Global Atmospheric Passive Sampling (GAPS) network (Fig. 1) was initiated in 2005 (ref. 1–3) to address domestic and international needs for tracking persistent organic pollutants (POPs) and chemicals of emerging interest in air. The core GAPS network, which has been operational for almost 20 years, includes more than 60 sites on all 7 continents and relies on collaboration with partner institutes and researchers.4–17 The network utilizes double-domed passive sampling shelters which house polyurethane foam (PUF) disk substrates and in some cases sorbent-impregnated PUF (SIP) disks.14,18 SIP disks have a greater sorptive capacity and are used when targeting more volatile chemicals, which may approach equilibrium with the PUF disk.18,19
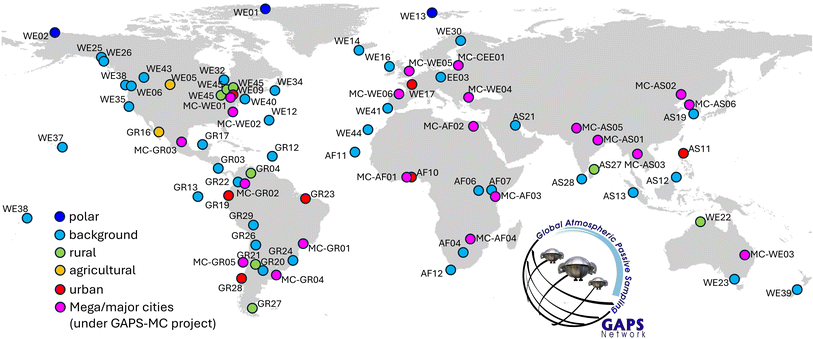 |
| Fig. 1 Sampling sites of the GAPS and GAPS megacities networks. Site names associated with codes are listed in Tables S1 and S2.† | |
The GAPS-type passive shelters allow both gas- and particle-associated chemicals to be sampled on the PUF and SIP disk substrates,20 with a sampling rate that is air-side controlled.21 For the given dimensions and surface area of the GAPS-type PUF disk (Tisch Environmental, Cleaves Ohio) and SIP disk (ANECO, Institut für Umweltschutz GmbH & Co), the sampling rate for PUF/SIP disks is approximately 4 m3 per day. Additional details regarding sampling characteristics are provided in the ESI (Text S1†) and in ref. 22.
The PUF and SIP disks are sheltered in double-dome flying-saucer-like housings (Tisch Environmental) made of stainless steel which protect the substrates from wet deposition and high winds but allow particles smaller than about 5 μm to infiltrate and be collected.20 The collection of particles by PUF and SIP disks samplers makes it possible to extend the use of these samplers to address many cross-cutting issues tied to air particulate matter (PM). These include: (i) chemical mixtures in air, including transformation products of POPs and priority chemicals.23 Oxidized chemicals typically experience reduced volatility compared to the parent chemical and will therefore sorb more strongly to PM so it is advantageous that both gas- and particle-phases are captured by the samplers; (ii) studies on trace metals in air,24,25 which are mainly associated with PM; (iii) linkages to PM-associated health effects such as oxidative potential,26 (iv) linkages to climate impacts associated with PM acting as a short-lived climate forcer e.g., black/brown carbon,27 and (v) biodiversity and impacts associated with bioaerosols and the aerobiome.28 Ambient PM comprises approximately 16–25% bioaerosol by mass.29
PUF and SIP disks samplers are typically deployed under GAPS network for periods of 2–3 months to up to one year (trace metals).25 Concentrations in air are derived from the quantity of chemicals accumulated in the PUF or SIP disk divided by the effective air sample volume, which can be estimated for most chemicals using the GAPS template.30 Despite the fact that calculations in the GAPS template are based on numerous calibration studies and have been verified independently by numerous research groups, the resulting values for concentrations in air should be considered semi-quantitative.31 However, for the purposes of generating a time-weighted average value for air, which spans a few months to a year, the passive sampling result may be preferable and possibly more accurate (as an average value) than averages estimated from intermittent “grab samples” of air using pumped samplers,32 especially if the grab samples represent only a small percentage of the averaging time period. This is especially true for chemicals whose levels in air may vary substantially from day to day and this variability cannot be captured by intermittent short-term (e.g., 24 h, once per week per month) sampling using active (pumped) air samplers. Eng et al. (2024) provide an overview of international studies reporting on the characterization/calibration of PUF/SIP disk samplers.22
In addition to the GAPS core network, a new GAPS Megacities (GAPS-MC) program was initiated in 2018 (Fig. 1), to study POPs and emerging chemicals in highly exposed and vulnerable urban populations.14 Whereas the GAPS network focuses on background sites and for characterizing the regional and global transport of chemicals, GAPS-MC targets human and environmental exposure and health impacts at 23 representative, major urban sites, where most POPs and many emerging chemicals are highly elevated in air.
This paper summarizes the transformation and continual evolution of the GAPS network from a program supporting the Global Monitoring Plan and Effectiveness Evaluation of the Stockholm Convention on POPs, to an “evergreen” platform for investigating current and future priorities and cross-cutting science and policy priorities such as chemical pollution and health, climate change, and biodiversity – as shown in Fig. 2. Some future directions and intersections of work under the GAPS networks are also discussed.
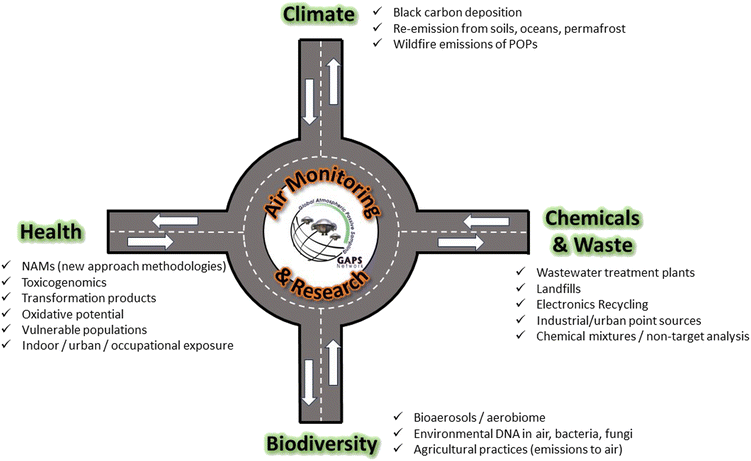 |
| Fig. 2 Intersections of science and policy with respect to air monitoring and research under the GAPS networks. | |
Informing domestic/international chemicals policy – new priorities and cross-cutting issues for air
The GAPS and GAPS-MC networks (collectively referred to as GAPS networks) inform domestic (Canadian) and international policy with respect to the presence and atmospheric transport of priority chemicals. They are the only global-scale programs reporting to the Global Monitoring Plan (GMP) of the Stockholm Convention on POPs. Reporting under the GMP occurs on a 6 year cycle and the 3rd phase of reporting was completed in 2023.33
Results from the GAPS networks support the Effectiveness Evaluation of the Stockholm Convention and inform on future needs for research and monitoring under the GMP. Because the GAPS program operates as a collaborative partnership that is coordinated by a single laboratory, where all samples are analyzed centrally, the data are internally consistent and well-suited for spatial and temporal trends analysis. Melymuk et al., have demonstrated the value of using a central lab in reducing data variability and uncertainty.31 Non-analyzed samples (raw PUF and SIP disks) and stored sample extracts collected under the GAPS networks comprise the GAPS sample archive (sample bank), which is maintained at at Environment and Climate Change Canada (ECCC) facilities in Toronto. The GAPS archive has allowed for retrospective analysis for addressing new research priorities as discussed below.
In recent years, the GAPS networks and sample archive have been used for investigatory work to expand the scope of the network beyond just POPs. By doing so, the GAPS network can address and contribute to new developments and priorities in the field of air monitoring and research. For instance, retrospective analysis of GAPS-MC samples allowed for confirmation of the presence of numerous transformation products of organophosphate esters (OPE) flame retardants in ambient air of megacities, revealed through laboratory oxidation studies.34 These transformation products represent just a very small subset of the diverse and complex chemical mixture make-up of ambient air, which is still largely uncharacterized and unknown.35 High resolution analysis (target and non-target) frameworks and analysis strategies will be essential in future studies. In addition, new approach methodologies (NAMs) for assessing chemical mixture toxicity and effects and advanced in silico approaches to derive chemical properties will be needed to provide a fuller characterization of hazards and risks.36
More recently, archived extracts from the GAPS network were screened for tire-wear derived chemicals (TDCs) and their transformation products (e.g., 6PPD-Q), to reveal their abundance and global distribution in ambient air.37 TDCs are known to cause acute mortality in Coho Salmon and concerns have been raised regarding levels in urban air and potential health impacts, including oxidative potential.38,39 The work on these rubber antioxidants and additives is continuing and will provide new information related to the air fate pathways and associated risks, including human exposure via inhalation.
The United Nations Environment Programme (UNEP) has declared a triple environmental crisis due to the increase in pollution (health), climate change and biodiversity loss.40 These topics provide incentive for future research and monitoring under the GAPS programs and for implementation of cross-cutting approaches as described in Fig. 2. These topics and examples are expanded upon in the following sections.
Linkages to health
Over the past decade, significant advances have been made in the development of NAMs, which do not require animal testing, for assessing toxicity endpoints associated with chemical exposure. PUF disk samplers are well suited for such studies because they provide a time-weighted average concentration of contaminants in air representing both gas-phase chemicals and particle-associated chemicals/materials.
Application of NAMs to assess cumulative effects associated with the entire mixture of chemicals in air is increasingly being recognized as an essential area of study because targeted instrumental analysis is only able to quantify a small percentage of the chemicals present in air, which does not represent the full toxicological load.35 Chemical burdens in air are dominated by a multitude of unknown compounds, many of which are transformation products of other known or unknown chemicals.34,41 As non-targeted analysis methods are being developed to characterize and quantify these chemical mixtures, NAMs provide an integrative approach for assessing and characterizing risk associated with effects such as genotoxicity, endocrine disruption, carcinogenicity, cognitive and neurological effects, and a multitude of other toxicity indicators.42,43
One relatively new toxicity metric (NAM) for air is referred to as oxidative potential (OP). OP is the ability of aerosol-bound chemicals to oxidize the native antioxidant (e.g. ascorbic acid, glutathione) and catalyze the production of reactive oxygen species (˙O2−, H2O2, ˙OH) in the respiratory, cardiovascular, and neurological systems.44–46 This process could ultimately lead to oxidative stress in the body, and this is believed to be an important pathway underlying the health effects of air pollution.47,48 Prominent chemicals that contribute to OP are transition metals (in particular Cu and Fe) and quinones; however, other species such as peroxides, secondary organic matter, and oxidation products of tire-wear chemicals, among others, could contribute to OP.26,45,49–53 Research to this date has focused on characterization of OP from particulate matter collected using active sampling technique. While metals are found primarily in particulate matter, semi-volatile organic species such as quinones are distributed in both gas and particulate phase in ambient air. Therefore, sampling techniques that allow capturing both phases will have an advantage for quantifying OP in ambient air. The PUF disk passive air sampler (PUF-PAS) has been previously used to measure trace metals and polycyclic aromatic compounds (PACs) from ambient air mixture.24,25,54,55 Hence, new methods are currently being developed to measure time-integrated chemical bioaccessibility and OP using PUF-disk samplers.50
Citizens of the world and even more vulnerable sub-populations are exposed to chemical pollution everyday through inhalation of ambient air (gases and particles) as well as through indoor air and occupational exposures. PUF-PAS has been implemented internationally to investigate chemical pollution emitted from point sources and to distinguish/characterize emissions from source sectors. PUF-PASs have also been used for assessing human exposure and risk in indoor and occupational studies. These applications of PUF-PAS samplers are summarized in Eng et al. (2024). For indoor studies, PUF-PAS are sometimes housed differently, in a chamber that includes just the top dome or cover plate to prevent the deposition of dust onto the sampler and to allow for greater air flow across the PUF/SIP disk.56,57 Reduced or stagnant air flow in indoor air results in lower sampling rates compared to outdoor air, especially when the double-dome housing is used.58
Until now, indoor/occupational sampling has not been implemented under the GAPS network, although the new GAPS-MC program is targeting highly exposed urban populations to address human exposure and health concerns. The recent 3rd Global Monitoring Report for the Stockholm Convention highlighted the need for more emphasis on urban and indoor exposure to POPs to better understand human exposure, burdens of POPs in human tissues and associated health risks.33
Linkages to climate research
Black carbon (BC) is a major short-lived climate forcer (SLCF) which contributes to global warming and human health effects of air pollution.59–63 The contribution to global warming is related to BC's strong ability to absorb sunlight and warm its surroundings. This affects the patterns of clouds and precipitation, melting of the ground snow and ice, and light reflection from the land and oceans, which ultimately leads to further warming of the earth.60,64 BC's effect on human health is through its capacity to act as carrier of toxic metals and organic species, some of which are well known for their carcinogenicity, oxidative potential, and respiratory cardiovascular effects.65 Current methods for measuring BC require sampling airborne particulate matter using active sampling technique;66–69 which can be costly and challenging to deploy at a large regional or global scale or may be unfeasible at remote sites due to the need for electricity and infrastructure. Hence, a new method was developed recently to quantify BC using PUF-PAS.27 PUF-PAS was found to be a suitable matrix for studying atmospheric BC with low background/blank levels (<4%) and low within-sample variability (as low as 3%). BC concentrations from passive samples were found to be comparable with those obtained from co-located active samples, and the values reflected well the nature of the sites and dominant emissions sources. More recently, a new PAS was tested for measuring BC which uses glass wool for capturing airborne particles.70 This new design eliminates the need for sample processing and particle retrieval, which is required with PUF-PAS before BC can be quantified with a thermal-optical method.
In addition to their application for measuring SLCFs, PUF-PAS provide an opportunity to study time-integrated trends for chemical species that are produced due to climate-induced processes such as wildfires. These events have been occurring more often over the past years and emitting significant amounts of carbon into the atmosphere. The total wildfire carbon emission in Canada in 2023 (∼290 megatons) was several folds higher than the average value from 2003–2022 period (i.e. ∼290 vs. ∼60 megatons).71 Some of the species produced from forest fires, such as BC (as discussed above) and substituted aromatic species,72 contribute to the absorption of sunlight and further warming of the earth. In addition to the emissions from the combustion of biomass, the heat generated by wildfires could result in volatilization and emission of PACs and other light-absorbing species which were historically accumulated by the vegetation and soil.73 This process could contribute to further warming of the climate, but it could also contribute to the health effects of air pollution. A recent study has reported high levels of bioaccessible trace metals from wildfire driven ash compared to the surrounding soil.74 Similarly, Kobziar et al. have recently shown for the first time that large amounts of microbial biomass is emitted from wildland fires and transported long distances in air with potential health consequences to ecosystems and humans.75
Linkages to biodiversity
In addition to bacteria and fungi that can be emitted to air predominantly from soils and through wildland fires, plants and animals in the terrestrial and aquatic environment release pieces of their DNA (e.g., dead skin cells, fecal matter) which can be emitted as particulate matter (PM) to air. Approximately 16 to 25% of ambient PM comprises environmental DNA (eDNA) which is accumulated in air and contributes to the aerobiome – the collection of living and dead microscopic organisms inhabiting the atmosphere.29,76,77 This provides an opportunity to monitor eDNA through air sampling and by doing so track spatial and temporal changes in biodiversity and the aerobiome. The Kunming-Montreal Global Biodiversity Framework was adopted at the fifteenth meeting of the Conference of Parties (COP15) and sets out 4 goals and 23 targets for a world living in harmony with nature by 2025.78 Target 7 in particular relates to reducing risks to biodiversity associated with harm and cumulative effects of pollution with a target date of 2030.
Consequently, there is a need for cost-effective methods for integrating pollution and biodiversity monitoring, which can be implemented quickly and over large spatial scales to track changes in biodiversity. This presents an excellent opportunity for utilizing existing PUF-PAS samples from the GAPS networks. Kalisa et al. (2024) have recently investigated and reported on the successful proof-of-concept for a sensitive method for measuring bacterial and fungal bioaerosols using PUF-PAS deployed across several sites in the oil sands region of Canada and in Toronto (Fig. 3).76 Passive sampling is well suited because it provides a time-integrated sample of ambient PM. The time-integrated nature of the sample can help to dampen/average the day-to-day dynamic variability in eDNA for a given sampling site. Such time-weighted samples are more practical for long-term monitoring of the aerobiome as few samples are required. Work is continuing under the oil sands monitoring program and GAPS network on calibration and improved characterization of the PUF-PAS method so that results can be interpreted quantitatively.
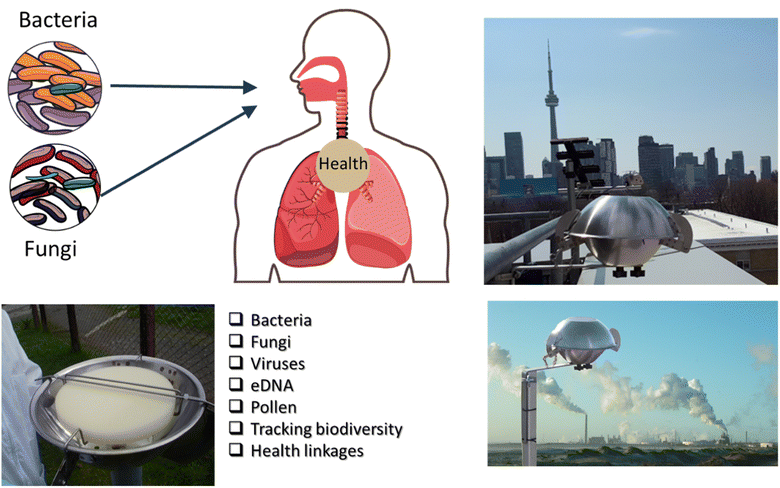 |
| Fig. 3 Tracking the aerobiome using PUF-PAS.76 | |
Research-policy gaps and future intersections under GAPS network
As presented above, research and monitoring of POPs and emerging chemicals under GAPS network is evolving to include cross-cutting issues in policy and science related to pollution/health, climate change and biodiversity loss. This increase in scope of the GAPS network is resulting in leveraging of activities, resources (funding), and generating a greater and richer breadth of results. This allows for a more holistic interpretation of contaminants and their impact on human health and the environment. This direction is consistent with calls for greater collaboration across the science–policy interfaces.79–81 This is being extended even further to include integration across policy–policy interfaces (e.g. Fig. 2) and outreach to the public.82
Future activities under the GAPS network will tackle the problem of an ever-increasing number of listed POPs and emerging chemicals and the associated challenges for air science, risk assessment and risk management.81,83
Significant contributions from the unique data and samples available from the GAPS network are envisioned in the following areas:
(i) Top-down approaches and inverse modelling – top-down approaches and inverse modelling, especially for the many POPs where emissions are unknown,84 and for newly revealed chemicals in air (e.g., transformation products) where absolutely no data exists on emissions.
(ii) Chemical mixtures approach – advanced analytical methods (non-target), in silico tools, and NAMs, can lead to rapid and cost-efficient approaches to assess chemical mixtures in air holistically, with increasing awareness of contributions from known and unknown components.
(iii) Specialized data analysis methods – advanced data analysis tools like principal components analysis and GIS land-use information)85 have been demonstrated for the GAPS network and could be used for interpreting and linking spatial and temporal trend information from different data sets. Herkert and Hornbuckle at the University of Iowa have developed and made available an on-line model for assessing PUF-PAS sampling rates using local meteorological data for any location in the world (https://s-iihr41.iihr.uiowa.edu/pufpas_model/).86,87
(iv) Global warning system – building internal capacity within ECCC as well as extending partnerships to include institutions involved in leading-edge NAMs and high-resolution analysis for establishing links between pollution, health, climate and biodiversity. For instance, a new project initiated in 2023 and led by Stockholm University (Airborne global early warning system for persistent mobile organic contaminants or ALARM) will use the existing GAPS network platforms to investigate persistent mobile organic contaminants (PMOCs) on a global scale.
(v) Citizen science and outreach – The role of citizen science can be powerful in reaching vulnerable populations and outreach with the public and institutions to better engage across the public interface.88 Raising awareness in the public is essential for the much-needed population-scale changes in thinking that will be needed to address current and future challenges.
Conflicts of interest
There are no conflicts of interest to declare.
Acknowledgements
Partial funding for this work was provided through the Chemicals Management Plan (Government of Canada), the United Nations Environment Programme (UNEP), and the Northern Contaminants Program (NCP). We would also like to thank and acknowledge the contributions made by our collaborators at all GAPS and GAPS-MC sites including Lynwill Martin, Willem Booysen, Peter Cloete, Casper Labuschagne, Thumeka Mkololo, Kenneth Arinaitwe, Stephen Mulinda, Vincent Madadi, Rose Alani, Katie Read, Luis Neves, Charita S. Kwan, Kristine Manalang, Vergel G. Valenzuela, Mohd Firdaus Bin Jahaya, Mohan Kumar A/L Sammathuria, Ahmad Fairudz B. Jamaluddin, Siti Aizza Binti Sarmami, Azra Binti Mohamed, Chrysanthus B. Gerardus, Mohd Fadley B. Yunus, Reza Mahdi, Ji-young Jeong, Karell Martínez-Guijarro, Penny Vlahos, Rohana Chandrajith, Jonathan Martin, Orjan Gustafsson, Krishnakant Budhavnt, Luisa Castillo, Angel Gálvez, Carlos Gonzales, Gina Marcela Avila Castano, Justin Yearwood, Lianda Chapman, Lisa Senhouse, Ana Cristina Tello, Sergio de los Santos Villalobos, Martin Villa Ibarra, Carlos Mauricio Alarcon Lazcano, Belén Lana, Angel David Galvez Serna, Carlos Mario Gonzales Duque, Gilberto Fillmann, Rodrigo O. Meire, Karina Miglioranza, Karla Pozo, Mariett Torres Gutierrez, Isabel Moreno, Fabricio Avila, Thomás Ištok, Milan Vana, Jana Klanova, Adéla Šmejkalová, Andrew Platt, Bryan Thomas, Ross Burgener, Jackson Vanfleet Brown, Renata Raina-Fulton, Vickie Irish, Nadine Borduas-Dedekind, Andrew Peters, Christina Menniti, Dan Dickinson, Pernilla Bohlin Nizzetto, Ove Hermanson, Dorothea Schulze, Hans Erik Fjeld, Erpur Snær Hansen, Gerður Stefánsdóttir, Sibylle von Löwis, Arni Sigurdsson, Jórunn Harðardóttir, Brian Delaney, Kieran Harper, Thomas Doherty, Hélène Blanchoud, Elodie Moreau Guigon, Dylan Campbell, Sam Cleland, Nigel Somerville, Jeremy Ward, Jennifer Powell, Melita Keywood, Pat Roach, Dylan Nordin, Carolyn (Elaine) Furbish, Mirka Hatanpää, Katriina Kyllönen, Janne Kärki, Doug Worthy, Bob Kessler, Andre Leclerc, Brian Congdon, Jason Surette, Gregory Stroud, Sarah Medill, Patrick Kleeman, Darryl Kuniyuki, Greg Rose, Christine Smith, Ryan Musick, Tim Holland, Chris Stewart, Emma Shipley, Begoña Jiménez, Juan Muñoz, Tawnya Hewitt, Lisa Larson, Natalia Prats, Concepción Bayo Pérez, Kenny Yan, Dave Halpin, Liisa Jantunen, Tamer Shoeib, Ravindra Sinha, Jianmin Ma, Narumol Jariyasopit, Takahiro Nishino, R. Suresh, Jerzy Falandysz, Maria Tominaga, Nestor Y. Rojas, Omar Amador-Muñoz, Carlos Manzano, Kurunthachalam Kannan, Gavin Stevenson, Alan Yates, Andy Sweetman, Beatriz H. Aristizabal-Suluaga.
References
- K. Pozo, T. Harner, S. C. Lee, F. Wania, D. C. Muir and K. C. Jones, Seasonally resolved concentrations of persistent organic pollutants in the global atmosphere from the first year of the GAPS study, Environ. Sci. Technol., 2008, 43(3), 796–803 CrossRef PubMed.
- K. Pozo, T. Harner, M. Shoeib, R. Urrutia, R. Barra and O. Parra, et al., Passive-sampler derived air concentrations of persistent organic pollutants on a north-south transect in Chile, Environ. Sci. Technol., 2004, 38(24), 6529–6537 CrossRef CAS PubMed.
- K. Pozo, T. Harner, F. Wania, D. C. Muir, K. C. Jones and L. A. Barrie, Toward a global network for persistent organic pollutants in air: results from the GAPS study, Environ. Sci. Technol., 2006, 40(16), 4867–4873 CrossRef CAS PubMed.
- A. Eng, K. Su, T. Harner, K. Pozo, R. K. Sinha and B. Sengupta, et al., Assessing Dicofol Concentrations in Air: Retrospective Analysis of GAPS Network Samples from Agricultural Sites in India, Environ. Sci. Technol. Lett., 2016, 3, 150–155 CrossRef CAS.
- S. Genualdi, T. Harner, Y. Cheng, M. MacLeod, K. M. Hansen and R. van Egmond, et al., Global distribution of linear and cyclic volatile methyl siloxanes in air, Environ. Sci. Technol., 2011, 45(8), 3349–3354 CrossRef CAS PubMed.
- S. Genualdi, S. C. Lee, M. Shoeib, A. Gawor, L. Ahrens and T. Harner, Global pilot study of legacy and emerging persistent organic pollutants using sorbent-impregnated polyurethane foam disk passive air samplers, Environ. Sci. Technol., 2010, 44(14), 5534–5539 CrossRef CAS PubMed.
- M. Koblizkova, S. C. Lee and T. Harner, Sorbent impregnated polyurethane foam disk passive air samplers for investigating current-use pesticides at the global scale, Atmos. Pollut. Res., 2012, 3(4), 456–462 CrossRef CAS.
- S. C. Lee, E. Sverko, T. Harner, K. Pozo, E. Barresi and J. Schachtschneider, et al., Retrospective analysis of “new” flame retardants in the global atmosphere under the GAPS Network, Environ. Pollut., 2016, 217, 62–69 CrossRef CAS PubMed.
- K. Pozo, T. Harner, S. C. Lee, R. K. Sinha, B. Sengupta and M. Loewen, et al., Assessing seasonal and spatial trends of persistent organic pollutants (POPs) in Indian agricultural regions using PUF disk passive air samplers, Environ. Pollut., 2011, 159(2), 646–653 CrossRef CAS PubMed.
- C. Rauert, T. Harner, J. K. Schuster, A. Eng, G. Fillmann and L. E. Castillo, et al., Air monitoring of new and legacy POPs in the Group of Latin America and Caribbean (GRULAC) region, Environ. Pollut., 2018, 243, 1252–1262 CrossRef CAS PubMed.
- C. Rauert, T. Harner, J. K. Schuster, K. Quinto, G. Fillmann and L. E. Castillo, et al., Towards a regional passive air sampling network and strategy for new POPs in the GRULAC region: Perspectives from the GAPS Network and first results for organophosphorus flame retardants, Sci. Total Environ., 2016, 573, 1294–1302 CrossRef CAS PubMed.
- C. Rauert, J. K. Schuster, A. Eng and T. Harner, Global Atmospheric Concentrations of Brominated and Chlorinated Flame Retardants and Organophosphate Esters, Environ. Sci. Technol., 2018, 52, 2777–2789 CrossRef CAS PubMed.
- C. Rauert, M. Shoieb, J. K. Schuster, A. Eng and T. Harner, Atmospheric concentrations and trends of poly-and perfluoroalkyl substances (PFAS) and volatile methyl siloxanes (VMS) over 7 years of sampling in the Global Atmospheric Passive Sampling (GAPS) network, Environ. Pollut., 2018, 238, 94–102 CrossRef CAS PubMed.
- A. Saini, T. Harner, S. Chinnadhurai, J. K. Schuster, A. Yates and A. Sweetman, et al., GAPS-Megacities: A new global platform for investigating persistent organic pollutants and chemicals of emerging concern in urban air, Environ. Pollut., 2020, 115416 CrossRef CAS PubMed.
- J. K. Schuster, T. Harner, A. Eng, C. Rauert, K. Su and K. C. Hornbuckle, et al., Tracking POPs in Global Air from the First 10 Years of the GAPS Network (2005 to 2014), Environ. Sci. Technol., 2021, 55, 9479–9488 CrossRef CAS PubMed.
- J. K. Schuster, T. Harner, G. Fillmann, L. Ahrens, J. C. Altamirano and B. Aristizábal, et al., Assessing polychlorinated dibenzo-p-dioxins and polychlorinated dibenzofurans in air across Latin American countries using polyurethane foam disk passive air samplers, Environ. Sci. Technol., 2015, 49(6), 3680–3686 CrossRef CAS PubMed.
- J. K. Schuster, T. Harner and E. Sverko, Dechlorane Plus in the Global Atmosphere, Environ. Sci. Technol. Lett., 2020, 8, 39–45 CrossRef.
- M. Shoeib, T. Harner, S. C. Lee, D. Lane and J. Zhu, Sorbent-impregnated polyurethane foam disk for passive air sampling of volatile fluorinated chemicals, Anal. Chem., 2008, 80(3), 675–682 CrossRef CAS PubMed.
- A. Dreyer, M. Shoeib, S. Fiedler, J. Barber, T. Harner and K.-W. Schramm, et al., Field intercomparison on the determination of volatile and semivolatile polyfluorinated compounds in air, Environ. Chem., 2010, 7(4), 350–358 CrossRef CAS.
- M. Z. Markovic, S. Prokop, R. M. Staebler, J. Liggio and T. Harner, Evaluation of the particle infiltration efficiency of three passive samplers and the PS-1 active air sampler, Atmos. Environ., 2015, 112, 289–293 CrossRef CAS.
- M. Shoeib and T. Harner, Characterization and comparison of three passive air samplers for persistent organic pollutants, Environ. Sci. Technol., 2002, 36(19), 4142–4151 CrossRef CAS PubMed.
- A. Eng, et al., Applications of Polyurethane Foam Based Passive Air Samplers (PUF-PAS) in Research and Monitoring: A review, in preparation.
- L. Liu, M. Lu, X. Cheng, G. Yu and J. Huang, Suspect screening and nontargeted analysis of per-and polyfluoroalkyl substances in representative fluorocarbon surfactants, aqueous film-forming foams, and impacted water in China, Environ. Int., 2022, 167, 107398 CrossRef CAS PubMed.
- E. O. Gaga, T. Harner, E. Dabek-Zlotorzynska, V. Celo, G. Evans and C. H. Jeong, et al., Polyurethane Foam (PUF) Disk Samplers for Measuring Trace Metals in Ambient Air, Environ. Sci. Technol. Lett., 2019, 6(9), 545–550 CrossRef CAS.
- J. Mastin, A. Saini, J. K. Schuster, T. Harner, E. Dabek-Zlotorzynska and V. Celo, et al., Trace Metals in Global Air: First Results from the GAPS and GAPS Megacities Networks, Environ. Sci. Technol., 2023, 57(39), 14661–14673 CrossRef CAS PubMed.
- P. Shahpoury, T. Harner, G. Lammel, S. Lelieveld, H. J. Tong and J. Wilson, Development of an antioxidant assay to study oxidative potential of airborne particulate matter, Atmos. Meas. Tech., 2019, 12(12), 6529–6539 CrossRef CAS.
- Z. W. Zhang, P. Shahpoury, W. Zhang, T. Harner and L. Huang, A new method for measuring airborne elemental carbon using PUF disk passive samplers, Chemosphere, 2022, 299, 134323 CrossRef CAS PubMed.
- J. M. Robinson and M. F. Breed, The aerobiome- health axis: a paradigm shift in bioaerosol thinking, Trends Microbiol., 2023, 31(7), 661–664 CrossRef CAS PubMed.
- A. Samake, G. Uzu, J. M. F. Martins, A. Calas, E. Vince and S. Parat, et al., The unexpected role of bioaerosols in the Oxidative Potential of PM, Sci. Rep., 2017, 7(1), 10978 CrossRef CAS PubMed.
- T. Harner, 2021 v10 Template for Calculating PUF and SIP Disk Sample Air Volumes April28, 2021 Search PubMed.
- L. Melymuk, P. B. Nizzetto, T. Harner, K. B. White, X. Wang and M. Y. Tominaga, et al., Global intercomparison of polyurethane foam passive air samplers evaluating sources of variability in SVOC measurements, Environ. Sci. Policy, 2021, 125, 1–9 CrossRef CAS PubMed.
- United Nations Environmental Program (UNEP), Guidance on the global monitoring plan for persistent organic pollutants, Geneva, 2013, Contract No.: UNEP/POPS/COP.6/INF/31 Search PubMed.
- United Nations Environmental Program, Third Global Monitoring Report under the Global Monitoring Plan for Effectiveness Evaluation, Geneva, 2023, Contract No.: UNEP/POPS/COP.11/INF/38 Search PubMed.
- Q. Liu, L. Li, X. Zhang, A. Saini, W. Li and H. Hung, et al., Uncovering global-scale risks from commercial chemicals in air, Nature, 2021, 600(7889), 456–461 CrossRef CAS PubMed.
- B. I. Escher, H. M. Stapleton and E. L. Schymanski, Tracking complex mixtures of chemicals in our changing environment, Science, 2020, 367(6476), 388–392 CrossRef CAS PubMed.
- X. Zhang, A. Saini, C. Hao and T. Harner, Passive air sampling and nontargeted analysis for screening POP-like chemicals in the atmosphere: Opportunities and challenges, TrAC, Trends Anal. Chem., 2020, 132, 116052 CrossRef CAS.
- C. Johannessen, J. Liggio, X. Zhang, A. Saini and T. Harner, Composition and transformation chemistry of tire-wear derived organic chemicals and implications for air pollution, Atmos. Pollut. Res., 2022, 101533 CrossRef CAS.
- Z. Tian, H. Zhao, K. T. Peter, M. Gonzalez, J. Wetzel and C. Wu, et al., A ubiquitous tire rubber–derived chemical induces acute mortality in coho salmon, Science, 2021, 371(6525), 185–189 CrossRef CAS PubMed.
- J. Sun, S. S. H. Ho, X. Niu, H. Xu, L. Qu and Z. Shen, et al., Explorations of tire and road wear microplastics in road dust PM2. 5 at eight megacities in China, Sci. Total Environ., 2022, 823, 153717 CrossRef CAS PubMed.
- United Nations Climate Change, What is the Triple Planetary Crisis?, 2022, https://unfccc.int/news/:-United-Nations-Climate-Change, Available from: https://unfccc.int/news/what-is-the-triple-planetary-crisis Search PubMed.
- K. Fenner, C. Kooijman, M. Scheringer and K. Hungerbühler, Including transformation products into the risk assessment for chemicals:: The case of nonylphenol ethoxylate usage in Switzerland, Environ. Sci. Technol., 2002, 36(6), 1147–1154 CrossRef CAS PubMed.
- K. Ha, P. Xia, D. Crump, A. Saini, T. Harner and J. O'Brien, Cytotoxic and transcriptomic effects in avian hepatocytes exposed to a complex mixture from air samples, and their relation to the organic flame retardant signature, Toxics, 2021, 9(12), 324 CrossRef CAS PubMed.
- S. Halappanavar, D. Wu, A. Boyadzhiev, A. Solorio-Rodriguez, A. Williams and N. Jariyasopit, et al., Toxicity screening of air extracts representing different source sectors in the Greater Toronto and Hamilton areas: In vitro oxidative stress, pro-inflammatory response, and toxicogenomic analysis, Mutat. Res. – Genet. Toxicol. Environ. Mutagen., 2021, 872, 503415 CrossRef CAS PubMed.
- J. T. Bates, T. Fang, V. Verma, L. H. Zeng, R. J. Weber and P. E. Tolbert, et al., Review of Acellular Assays of Ambient Particulate Matter Oxidative Potential: Methods and Relationships with Composition, Sources, and Health Effects, Environ. Sci. Technol., 2019, 53(8), 4003–4019 CrossRef CAS PubMed.
- P. Shahpoury, Z. W. Zhang, A. Filippi, S. Hildmann, S. Lelieveld and B. Mashtakov, et al., Inter-comparison of oxidative potential metrics for airborne particles identifies differences between acellular chemical assays, Atmos. Pollut. Res., 2022, 13(12), 101596 CrossRef CAS.
- M. Shiraiwa, K. Ueda, A. Pozzer, G. Lammel, C. J. Kampf and A. Fushimi, et al., Aerosol Health Effects from Molecular to Global Scales, Environ. Sci. Technol., 2017, 51(23), 13545–13567 CrossRef CAS PubMed.
- D. Gao, S. Ripley, S. Weichenthal and K. J. G. Pollitt, Ambient particulate matter oxidative potential: Chemical determinants, associated health effects, and strategies for risk management, Free Radical Biol. Med., 2020, 151, 7–25 CrossRef CAS PubMed.
- F. J. Kelly and J. C. Fussell, Role of oxidative stress in cardiovascular disease outcomes following exposure to ambient air pollution, Free Radical Biol. Med., 2017, 110, 345–367 CrossRef CAS PubMed.
- Q. F. Liu, P. Shahpoury, J. Liggio, T. Harner, K. Li and P. Lee, et al., Understanding the Key Role of Atmospheric Processing in Determining the Oxidative Potential of Airborne Engineered Nanoparticles, Environ. Sci. Technol. Lett., 2020, 7(1), 7–13 CrossRef CAS.
- P. Shahpoury, S. Lelieveld, C. Johannessen, T. Berkemeier, V. Celo and E. Dabek-Zlotorzynska, et al., Influence of aerosol acidity and organic ligands on transition metal solubility and oxidative potential of fine particulate matter in urban environments, Sci. Total Environ., 2024, 906, 167405 CrossRef CAS PubMed.
- P. Shahpoury, Z. W. Zhang, A. Arangio, V. Celo, E. Dabek-Zlotorzynska and T. Harner, et al., The influence of chemical composition, aerosol acidity, and metal dissolution on the oxidative potential of fine particulate matter and redox potential of the lung lining fluid, Environ. Int., 2021, 148, 106343 CrossRef CAS PubMed.
- P. Wang, A. Qi, Q. Huang, Y. Wang, X. Tuo and T. Zhao, et al., Spatial and temporal variation, source identification, and toxicity evaluation of brominated/chlorinated/nitrated/oxygenated-PAHs at a heavily industrialized area in eastern China, Sci. Total Environ., 2022, 822, 153542 CrossRef CAS PubMed.
- S. Weichenthal, M. Shekarrizfard, A. Traub, R. Kulka, K. Al-Rijleh and S. Anowar, et al., Within-City Spatial Variations in Multiple Measures of PM(2.5) Oxidative Potential in Toronto, Canada, Environ. Sci. Technol., 2019, 53(5), 2799–2810 CrossRef CAS PubMed.
- N. Jariyasopit, T. Harner, C. Shin and R. Park, The effects of plume episodes on PAC profiles in the athabasca oil sands region, Environ. Pollut., 2021, 282, 117014 CrossRef CAS PubMed.
- N. Jariyasopit, P. Tung, K. Su, S. Halappanavar, G. J. Evans and Y. Su, et al., Polycyclic aromatic compounds in urban air and associated inhalation cancer risks: A case study targeting distinct source sectors, Environ. Pollut., 2019, 252, 1882–1891 CrossRef CAS PubMed.
- M. Shoeib, T. Harner, G. M. Webster, E. Sverko and Y. Cheng, Legacy and current-use flame retardants in house dust from Vancouver, Canada, Environ. Pollut., 2012, 169, 175–182 CrossRef CAS PubMed.
- B. H. Wilford, T. Harner, J. P. Zhu, M. Shoeib and K. C. Jones, Passive sampling survey of polybrominated diphenyl ether flame retardants in indoor and outdoor air in Ottawa, Canada: Implications for sources and exposure, Environ. Sci. Technol., 2004, 38(20), 5312–5318 CrossRef CAS PubMed.
- S. Hazrati and S. Harrad, Calibration of polyurethane foam (PUF) disk passive air samplers for quantitative measurement of polychlorinated biphenyls (PCBs) and polybrominated diphenyl ethers (PBDEs): Factors influencing sampling rates, Chemosphere, 2007, 67(3), 448–455 CrossRef CAS PubMed.
- Arctic Monitoring and Assessment Programme (AMAP), Trends in Stockholm Convention Persistent Organic Pollutants (POPs) in Arctic Air, Human media and Biota, Arctic Monitoring and Assessment Programme (AMAP), 2014, Report No.: 8279710841 Search PubMed.
- E. Bush. Canada's Changing Climate Report Post External Review Meeting March 27-28, 2018, 2019 Search PubMed.
- R. Niranjan and A. K. Thakur, The Toxicological Mechanisms of Environmental Soot (Black Carbon) and Carbon Black: Focus on Oxidative Stress and Inflammatory Pathways, Front. Immunol., 2017, 8, 763 CrossRef PubMed.
- U. Pöschl and M. Shiraiwa, Multiphase Chemistry at the Atmosphere-Biosphere Interface Influencing Climate and Public Health in the Anthropocene, Chem. Rev., 2015, 115(10), 4440–4475 CrossRef PubMed.
- V. Ramanathan and G. Carmichael, Global and regional climate changes due to black carbon, Nat. Geosci., 2008, 1(4), 221–227 CrossRef CAS.
- D. Shindell and G. Faluvegi, Climate response to regional radiative forcing during the twentieth century, Nat. Geosci., 2009, 2(4), 294–300 CrossRef CAS.
- M. Antiñolo, M. D. Willis, S. M. Zhou and J. P. D. Abbatt, Connecting the oxidation of soot to its redox cycling abilities, Nat. Commun., 2015, 6, 6812 CrossRef.
- F. Cavalli, M. Viana, K. E. Yttri, J. Genberg and J. P. Putaud, Toward a standardised thermal-optical protocol for measuring atmospheric organic and elemental carbon: the EUSAAR protocol, Atmos. Meas. Tech., 2010, 3(1), 79–89 CrossRef CAS.
- T. W. Chan, L. Huang, K. Banwait, W. Zhang, D. Ernst and X. L. Wang, et al., Inter-comparison of elemental and organic carbon mass measurements from three North American national long-term monitoring networks at a co-located site, Atmos. Meas. Tech., 2019, 12(8), 4543–4560 CrossRef CAS.
- J. C. Chow, J. G. Watson, L. W. A. Chen, W. P. Arnott, H. Moosmüller and K. Fung, Equivalence of elemental carbon by thermal/optical reflectance and transmittance with different temperature protocols, Environ. Sci. Technol., 2004, 38(16), 4414–4422 CrossRef CAS PubMed.
- L. Huang, J. R. Brook, W. Zhang, S. M. Li, L. Graham and D. Ernst, et al., Stable isotope measurements of carbon fractions (OC/EC) in airborne particulate: A new dimension for source characterization and apportionment, Atmos. Environ., 2006, 40(15), 2690–2705 CrossRef CAS.
- X. Wang, J. Li, X. Y. Zhang, Z. N. Cheng, H. Y. Jiang and H. X. Jiang, et al., An innovative passive sampler to reveal the high contribution of biomass burning to black carbon over Indo-China Peninsula: Radiocarbon constraints, Atmos. Environ., 2023, 294, 119522 CrossRef CAS.
- Copernicus Atmosphere Monitoring Service (CAMS), 2023: A year of intense global wildfire activity, Copernicus Atmosphere Monitoring Service (CAMS), 2024, https://atmosphere.copernicus.eu, [Available from: https://atmosphere.copernicus.eu/2023-year-intense-global-wildfire-activity Search PubMed.
- Z. Kitanovski, P. Shahpoury, C. Samara, A. Voliotis and G. Lammel, Composition and mass size distribution of nitrated and oxygenated aromatic compounds in ambient particulate matter from southern and central Europe - implications for the origin, Atmos. Chem. Phys., 2020, 20(4), 2471–2487 CrossRef CAS.
- J. Schuster, T. Harner, K. Su, A. Eng, A. Wnorowski and J.-P. Charland, Temporal and spatial trends of polycyclic aromatic compounds in air across the Athabasca oil sands region reflect inputs from open pit mining and forest fires, Environ. Sci. Technol. Lett., 2019, 6, 178–183 CrossRef CAS.
- C. M. Villarruel, L. A. Figueroa and J. F. Ranville, Quantification of Bioaccessible and Environmentally Relevant Trace Metals in Structure Ash from a Wildland-Urban Interface Fire, Environ. Sci. Technol., 2024, 58(5), 2502–2513 CrossRef CAS PubMed.
- L. N. Kobziar, P. Lampman, A. Tohidi, A. K. Kochanski, A. Cervantes and A. T. Hudak, et al., Bacterial Emission Factors: A Foundation for the Terrestrial-Atmospheric Modeling of Bacteria Aerosolized by Wildland Fires, Environ. Sci. Technol., 2024, 58(5), 2413–2422 CrossRef CAS PubMed.
- E. S. Kalisa, A. Saini, K. Lee, J. Mastin, J. K. Schuster and T. Harner, Capturing the Aerobiome: Application of Polyurethane Foam Disk Passive Samplers for Bioaerosol Monitoring, ACS EST Air, 2024 DOI:10.1021/acsestair.3c00107.
- E. L. Clare, C. K. Economou, F. J. Bennett, C. E. Dyer, K. Adams and B. McRobie, et al., Measuring biodiversity from DNA in the air, Curr. Biol., 2022, 32(3), 693–700 CrossRef CAS PubMed.
- United Nations Environment Program (UNEP), Kunming-Montreal Global Biodiversity Framework, Montreal, Canada, 2022, Contract No.: CBD/COP/DEC/15/4 Search PubMed.
- Z. Y. Wang, R. Altenburger, T. Backhaus, A. Covaci, M. L. Diamond and J. O. Grimalt, et al., We need a global science-policy body on chemicals and waste, Science, 2021, 371(6531), 774 CrossRef CAS PubMed.
- Z. Y. Wang, S. Adu-Kumi, M. L. Diamond, R. Guardans, T. Harner and A. Harte, et al., Enhancing Scientific Support for the Stockholm Convention's Implementation: An Analysis of Policy Needs for Scientific Evidence, Environ. Sci. Technol., 2022, 56(5), 2936–2949 CrossRef CAS PubMed.
- M. L. Diamond, C. A. de Wit, S. Molander, M. Scheringer, T. Backhaus and R. Lohmann, et al., Exploring the planetary boundary for chemical pollution, Environ. Int., 2015, 78, 8–15 CrossRef CAS PubMed.
- P. Vlahos, E. R. Shipley, C. Rauert, P. Tung, R. Chandrajith and S. Wickramarathna, et al., A Hitchhiker's guide to persistent organic pollutants (POPs) monitoring in air: Establishing a baseline network in Sri Lanka, Atmos. Pollut. Res., 2023, 14(12), 101901 CrossRef CAS.
- X. Zhang, R. A. Di Lorenzo, P. A. Helm, E. J. Reiner, P. H. Howard and D. C. Muir, et al., Compositional space: A guide for environmental chemists on the identification of persistent and bioaccumulative organics using mass spectrometry, Environment international, 2019, 132, 104808 CrossRef CAS PubMed.
- T. F. M. Rodgers, A. Giang, M. L. Diamond, E. Gillies and A. Saini, Emissions and fate of organophosphate esters in outdoor urban environments, Nat. Commun., 2023, 14(1), 1175 CrossRef CAS PubMed.
- J. K. Schuster, T. Harner and C. Rauert, Impacts of Proximity to Primary Source Areas on Concentrations of POPs at Global Sampling Stations Estimated from Land Cover Information, ACS Omega, 2023, 8(39), 36016–36024 CrossRef CAS PubMed.
- N. J. Herkert, S. N. Spak, A. Smith, J. K. Schuster, T. Harner and A. Martinez, et al., Calibration and evaluation of PUF-PAS sampling rates across the Global Atmospheric Passive Sampling (GAPS) network, Environ. Sci.: Processes Impacts, 2018, 20(1), 210–219 RSC.
- The University of Iowa, PUF-PAS Sampling Rate Model Interface, 2020, [Available from: https://s-iihr41.iihr.uiowa.edu/pufpas_model/ Search PubMed.
- B. Schaefer TK and C. M. Fabian, Citizen-Based Air Quality Monitoring: The Impact on Individual Citizen Scientists and How to Leverage the Benefits to Affect Whole Regions, Citizen Science: Theory and Practice, 2020, 5(1), 6 Search PubMed.
|
This journal is © The Royal Society of Chemistry 2024 |