DOI:
10.1039/D4RA00934G
(Paper)
RSC Adv., 2024,
14, 8366-8377
The dual-model up/down-conversion green luminescence of NaSrGd(MoO4)3: Er3+ and its application for temperature sensing†
Received
5th February 2024
, Accepted 6th March 2024
First published on 12th March 2024
Abstract
Er3+-doped phosphors are widely used as dual-functional optical thermometers due to their distinctive up/down-conversion luminescence and the thermally coupled energy states (2H11/2 and 4S3/2) of Er3+. The development of high-performance Er3+-activated optical thermometers is both an intriguing subject and a formidable challenge in the field. This article investigates the up/down-conversion (UC and DC) photoluminescence properties of NaSrGd(MoO4)3 (NSGM): Er3+. When excited at 375 and 975 nm, the phosphors emit peaks at 530, 550, and 657 nm, corresponding to the 2H11/2, 4S3/2, and 4F9/2 → 4I15/2 transitions of Er3+, with the 4S3/2 → 4I15/2 transition displaying the highest intensity. The optical properties are comprehensively studied through UV-visible absorption, PL spectroscopy, and PLE spectroscopy. Optimal luminescence intensity is achieved at an Er3+ concentration of 4% mol. The resulting chromatic coordinates (x, y) and high correlated color temperature (CCT) values of the doped phosphors yield thermally stable cold emissions in the green region, boasting color purities of approximately 98.76% and 80.74% for DC and UC conversion, respectively. The optical temperature sensing properties of thermally coupled energetic states are explored based on the fluorescence intensity ratio principle. NSGM: 0.04Er3+, under 375 nm light excitation, demonstrates the maximum relative sensitivity of 0.87%/K−1 at 298.15 K, spanning a wide temperature range from 298.15 to 488.15 K. Conversely, under 975 nm light excitation, NSGM: 0.04Er3+ exhibits the maximum relative sensitivity of 0.63%/K−1 over the same temperature range, with temperature uncertainty (δT) less than 0.50 K and repeatability (R) (more than 98%). These findings position this material as a promising candidate for optical thermometer applications. The optical heating capacity of the synthesised phosphor is also determined using optical thermometry results, and heat generation up to approximately 457 K is found, indicating that NSGM: 0.04Er3+ could be useful for photo-thermal therapy.
I. Introduction
Researchers are currently highly motivated by the development of new phosphorescent materials due to their diverse applications in fields such as solid-state lighting, display devices, bio-imaging, latent fingerprint detection, non-contact temperature sensing, solar cells, and indoor plant growth.1–3 As a result, the development of an effective phosphorescent material using a suitable host and ion activator is still a pressing need. Phosphorescent materials can be broadly categorized into up/down-converting phosphorescent, where the former converts high-energy photons into low-energy ones, and the latter does the opposite. Compared with other rare earth-doped luminescent matrix materials, molybdate has been proposed as a highly efficient luminescent material owing to its significant properties of good chemical stability, high quantum yield, and versatile morphology. These materials exhibit broad and intense absorption bands in the near-UV region, primarily attributed to charge transfer (CT) transitions.4–8 The NSGM is highly regarded as an excellent luminescent material capable of efficient up/down-conversion emission. This is due to its stable physical and chemical properties, as well as its notably low phonon energy.8–11 Notably, rare earth ions, particularly Er3+ ions introduced into a suitable host, exhibit two highly intense emission peaks at around 530 and 550 nm under both UV and near-infrared excitations.12–16 Consequently, Er3+ ions can serve as effective activators, and NSGM molybdates are ideal hosts for the development of green phosphorescent materials. In addition to these considerations, the quest for DC and UC phosphor materials for high-temperature thermometry with enhanced accuracy poses a significant challenge for researchers. The challenge arises from the thermal quenching of emission in high-temperature conditions.17–21 Within this context, our article explores the realm of non-contact temperature sensing, an essential parameter that demands precise regulation in various scientific experiments. Traditional thermometers necessitate direct contact with the object under measurement, rendering them ineffective in a multitude of challenging and remote situations, such as high-temperature reactors, hazardous radiation zones, underground mines, and fast-moving objects. To address these limitations, the article discusses the potential of non-contact optical thermometers, which rely on the fluorescence intensity ratio (FIR) of two luminescence peaks, offering a reliable solution to the problem. The FIR method is based on the ratio of luminescence intensities from either thermally coupled energy levels of a single activator ion or non-thermally coupled energy levels of two activator ions. The key advantage of FIR-based thermometers lies in their high accuracy, as they are independent of the excitation source and external factors.22–26 The existence of two thermally coupled energy levels, specifically 2H11/2 and 4S3/2 of Er3+ ions enable their application in temperature sensing scenarios. In this study, NSGM was chosen as the matrix due to its simple preparation, high physical stability and environmental friendliness. Er3+ ions were used as activators. DC and UC photoluminescence emission spectra, excited by UV light at 375 nm and near-infrared radiation at 975 nm respectively, as well as sensitivities related to temperature detection, were investigated in depth. Therefore, the NSGM: Er3+ phosphor described in this article holds promise for temperature sensing applications. The optical heating capacity of the synthesized phosphorus is also determined using optical thermometry results, and heat generation is found, indicating that NSGM: Er3+ could be useful for photo-thermal therapy.
II. Experimental section
The solid-state reaction method was adopted to synthesize a series of erbium Er3+-doped NSGM materials. The raw materials, namely Na2CO3, SrCO3, Gd2O3, Er2O3 and MoO3, were used in appropriate proportions. All precursors were carefully sampled, ground with an agate mortar and then calcined at 673 K for 8 hours. They were then cooled, ground again and compressed into 8 mm diameter pellets using a stainless-steel mold. The pellets were then heated at a temperature of 1273 K for a period of 12 hours. All chemical reagents used in this study were purchased from Sigma-Aldrich and employed without the need for further purification steps. The chemical reaction formula is as follows:
Na2CO3 + 2SrCO3 + (1 − x)Gd2O3 + xEr2O3 + 6MoO3 → 2NaSrGd1−xErx(MoO4)3 + 3CO2 |
III. Characterization techniques
The powder X-ray diffraction (PXRD) patterns of the sample were performed at room temperature using a powder X-ray D8-Advance Bruker diffractometer, with monochromatic copper radiation CuKa1 (1.5406 Å) on the 20–80 2θ degree range. The samples' morphologies were examined using a Zeiss Supra55VP FEG-SEM and Bruker XFlash 5030. UV-vis-NIR absorption was determined using a UV-vis-NIR spectrometer (PerkinElmer Lambda 365). Photoluminescence excitation (PLE) and photoluminescence (PL) spectra investigations were carried out in Fluoromax 4 P model Horiba spectrometer equipped with a xenon lamp as the excitation source at room temperature. For the optical thermometry study, the sample are placed inside a homemade nano heater chamber having controlled heating with an accuracy of ±0.5 K. Through 375 nm and 975 nm diode laser excitations at constant pump power of 30 mW the emission spectra were recorded on monochromator (Horiba Jobin Yvon, iHR320) spectrometer. The monochromator uses an 1800 g per mm grating blazed at 500 nm and a photomultiplier tube (Hamamatsu, R928) to measure luminescence in the green (520–570 nm) emission bands. The temperature of the sample was varied from room temperature to 488.15 K with the interval of 10 K.
IV. Result and discussion
1. X-ray diffraction analysis
X-ray diffraction (XRD) patterns were used to study the crystal structure and phase purity of the samples. Fig. 1(a) shows XRD patterns of NSGM: xEr3+ microcrystals (with x = 0.02, 0.04, 0.06 and 0.08) in the 20–80 2θ° range. According to these NSGM XRD diagrams (JCPDS no. 70-0257), the samples exhibit a tetragonal structure with a space group I41/a (88), which is isomorphic with CaMoO4.27 The peaks are precisely indexed and no additional peaks are observed in the XRD diagram. This indicates that the prepared luminophores have a single-phase formation, confirming the successful incorporation of the Er3+ ion into the Gd3+ site. The X-ray diffraction peak shifts to the right because the ionic radii of Er3+ (r = 0.1062 nm) is smaller than that of Gd3+ (r = 0.107 nm). In addition, Rietveld refinement (Fig. S1†) was used to check the structural parameters (space group and cell parameters), and Table S1† shows the lattice parameters and corresponding unit cell volumes of the synthesized luminophores. Fig. 1(b) displays SEM micrographs of NSGM: 0.04% Er3+. The micrographs reveal particles with irregular shapes that tend to aggregate. These particles have sizes in the micrometer range, approximately 2 μm.
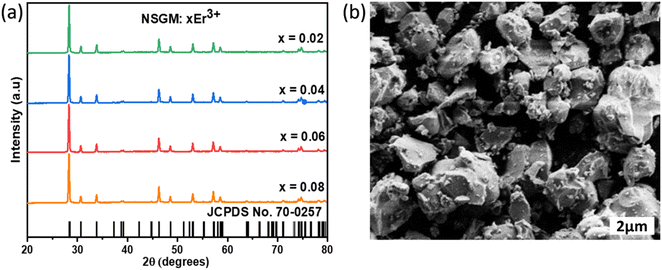 |
| Fig. 1 (a) XRD of the NSGM: xEr3+ (x = 0.02, 0.04, 0.06 and 0.8), (b) SEM image of NSGM: 0.04Er3+. | |
2. Optical properties
2.1. UV absorption spectroscopy. The diffuse reflectance spectra of NSGM: 0.04Er3+ phosphors in the 200–1100 nm range is shown in Fig. 2(a); the spectra show a broad absorption band around 320 nm, attributed to electronic transitions of the (MoO4) group.28 The other absorption bands around 380, 406, 450, 488, 530, 657, 801 and 975 nm, which are attributed to the 4I15/2 → 4G11/2, 4I15/2 → 2H9/2, 4I15/2 → 4F5/2, 4I15/2 → 4F7/2, 4I15/2 → 2H11/2, 4I15/2 → 4F9/2, 4I15/2 → 4I9/2, and 4I15/2 → 4I11/2 electron transitions, respectively, of Er3+ ions. Kubelka and Munk30 propounded the calculation of the band gap of a material using the diffuse reflectance spectrum. Can be calculated by using the Kubelka–Munk (K–M) function and the Tauc relationship and can be written by the following expression:29 |
[F(R)∞hν] = B(hν − Eg)n
| (1) |
where, B is the proportionality constant and n is a constant that characterizes the nature of the band transition which can have values n = 1/2 or 2 for direct or indirect band transitions, respectively. The plot is displayed in Fig. 2(b). Prior literature affirms that NSGM is a direct band gap material,30 and its estimation is based on plotting [F(R)hν]2 as a function of hν for a direct band gap transition. From the graph of [F(R)hν]2 versus hν, the value of Eg was extracted by extrapolating the linearly fitted regions until [F(R)hν]2 reached zero. The band gap for NSGM: 0.04Er3+ is calculated to be 3.59 eV, which is close to values observed in previous reports.6
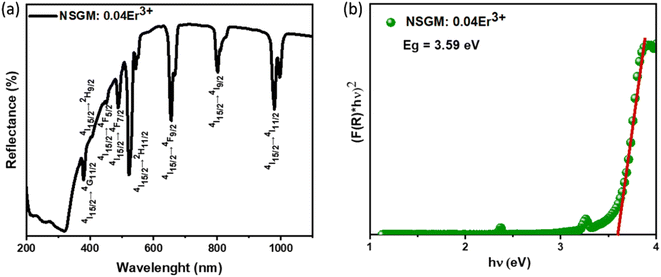 |
| Fig. 2 (a) Diffuse reflectance spectra and (b) the plot of [F(R)hν]2 versus (hν) of NSGM: 0.04Er3+. | |
2.2. Down and up-conversion photoluminescence. To gain a deeper understanding of the potential of the produced powder as a new phosphor, a thorough and systematic analysis of the excitation and photoluminescence emission of Er3+-doped NSGM was conducted. Fig. 3(a) shows the DC excitation spectrum of NSGM: 0.04 Er3+ microcrystals, measured by monitoring the emission at 545 nm. This spectrum shows a broad band and several narrow peaks. The broad excitation band ranging from 250 to 350 nm with a central wavelength of about 304 nm was attributed to the charge transfer band (CTB) from the fully filled 2p orbitals of oxygen ions to the partially filled 5d orbitals of molybdenum ions.31,32 And the narrow peaks located at about 365, 378, 406, 450, and 488 nm which correspond to the spin forbidden 4f–4f transitions of Er3+ ions from the ground state of 4I15/2 to 4G9/2, 4G11/2, 2H9/2, 4F5/2, and 4F7/2, respectively.33,34 This novel phosphor can be well excited by UV (200–500 nm), suggesting that the Er3+-activated NSGM are promising candidates for solid-state lighting as green-emitting phosphors. Furthermore, the DC emission spectrum is formed by two intense green emissions at 530 and 550 nm corresponding to the 2H11/2 → 4I15/2, 4S3/2 → 4I15/2 transitions of Er3+ ions, respectively, and a faint red emission at about 657 nm, which was attributed to the 4F9/2 → 4I15/2 transition,35 as shown in Fig. S2(b).† The observed emission bands were consistent with those found in other luminescent materials Er3+ ions-based.36,37
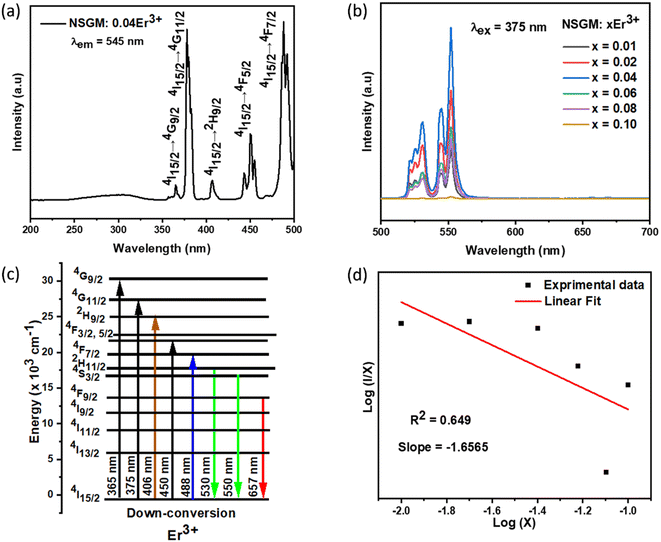 |
| Fig. 3 (a) Excitation spectra monitored at λem = 545 nm of NSGM: 0.04Er3+; (b) emission spectrum of NSGM: xEr3+ excited at 375 nm; (c) the energy level diagram Er3+ ion showing the energy transfer process in down-conversion mechanism and (d) relations between the log(I/x) and log(x) in NSGM: xEr3+. | |
Fig. 3(b) shows the DC emission spectra of NSGM: xEr3+ (x = 0.01, 0.02, 0.04, 0.06, and 0.08 and 0.10) recorded at the excitation wavelength of 375 nm. Two light emission peaks are present at 530 and 550 nm in the green region, and a faint peak is present in the red region at 657 nm. These are the characteristic emission peaks of Er3+ ions, and the electronic transitions responsible for these peaks are 2H11/2 → 4I15/2 (530 nm), 4S3/2 → 4I15/2 (550 nm) and 4F9/2 → 4I15/2 (657 nm).38–40 Fig. 3(c) shows a schematic representation of the energy levels of the Er3+ ion and the electronic transitions involved in the excitation and emission phenomena. When the material is excited at 375 nm, the electrons of Er3+ ions go from the ground state 4I15/2 to the excited state 4G11/2. The electrons then relax non-radiatively to the 2H11/2, 4S3/2 and 4F9/2 states. Then the radiative transitions 2H11/2 → 4I15/2 (530 nm), 4S3/2 → 4I5/2 (550 nm), and 4F9/2 → 4I15/2 (657 nm) take place. We can see from Fig. 3(b) that the change in doping percentage does not affect the position of the emission peaks. However, the emission becomes brighter as the doping percentage increases from 1% to 4% mol Er3+. The brightest emission is observed at 4% mol Er3+ ion doping. A further increase in the doping level reduces the brightness of the material due to the concentration quenching effect. The type of interaction between Er3+ ions that causes the concentration quenching effect. This could be realized by evaluating the critical energy transfer distance (Rc):41,42
|
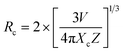 | (2) |
The formula for the critical distance of Er3+ ions in NSGM arrays is approximately Rc = 15.76 Å, indicating the involvement of the electric multipolar interaction. To better understand the energy transfer mechanism in NSGM: xEr3+, the following equation, suggested by Dexter, is provided:43–45
|
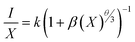 | (3) |
The electric multipole interaction includes dipole–dipole, dipole-quadrupole and quadrupole–quadrupole interactions, using (eqn (3)). The type of energy transfer mechanism can be identified by analyzing the value of θ in the relationship between luminescence intensity (I) and doping concentration (X). Fig. 3(d) illustrates a linear relationship between log(I/X) and log(X), where the slope of the fitted straight line is −1.6565. The value of θ is found to be 4.9, which is approximately the same as the value of 6. This demonstrates that the non-radiative energy transfer between Er3+ ions in the NSGM host lattice is primarily governed by the electric dipole–dipole (d–d) interaction. Fig. 3(b) illustrates the electron transition process of DC emission when excited by a 375 nm wavelength. Under a 375 nm ultraviolet excitation, electrons are excited to the 4G11/2 state and subsequently return to the ground state through a relaxation process. This results in the narrowband luminescence characteristic of the Er3+ 4f–4f luminescence in the visible region, as determined by previous reports and the energy levels of Er3+ ions.
Contrary to most prior publications, Er3+ was able to obtain self-activated UC luminescence in the absence of Yb3+ in this study. In the matching UC spectra, a green band comprising two emission peaks situated at 530 and 550 nm can be seen (Fig. 4(a)), which are ascribed to Er3+ 4f–4f electronic transitions: 2H11/2, 4S3/2 → 4I15/2.
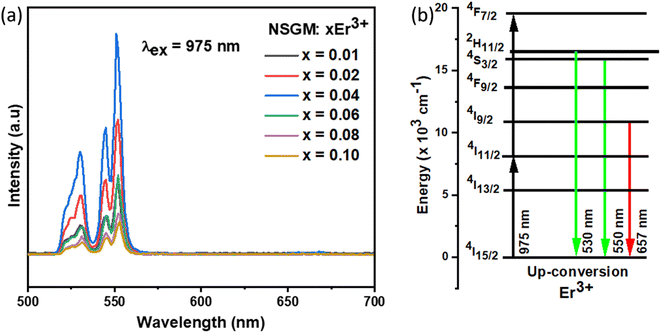 |
| Fig. 4 (a) Emission spectrum of NSGM: xEr3+ (x = 0.01, 0.02, 0.04, 0.06, 0.08 and 0.10) excited at 975 nm; (b) the energy level diagram Er3+ ion showing the energy transfer process in down-conversion mechanism. | |
The optimal doping ion concentration is found to be 4% mol Er3+, which is comparable to the luminescence behavior stimulated by 375 nm. UC luminescence is a nonlinear process whose luminous intensity (IUC) and pump power (P) meet the relationship:46,47
where
n is the number of pumping photons necessary to excite the electron of the luminescent ion from the ground state to the excited state.
37 The linear fitting of a two-log plot of UC luminescence intensity (
Fig. 5) and pump power yields the value of
n.
Fig. 5 depicts the two emission peaks (530 and 550) of the NSGM: 0.04Er
3+ sample, with slopes computed at 530 and 550 nm, respectively, suggesting that a two-photon process produced the UC luminescence.
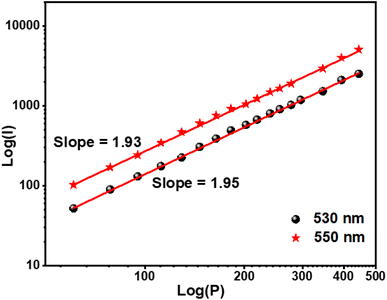 |
| Fig. 5 Log(I) versus log(P) plot of 530 and 550 nm peaks of NSGM: 0.04Er3+. | |
It should be mentioned that both the excited state absorption (ESA) of Er3+ and the energy transfer (ET) between Er3+ were the primary sources of the green and red UC emissions. Er3+ was initially excited by a 975 nm photon, transitioning from the ground state 4I15/2 level to the 4I11/2 level, or GSA: 4I15/2 + a photon → 4I11/2. Subsequently, this Er3+ may absorb another 975 nm photon (ESA process) or transmit its energy to another Er3+ (ET process) in the 4I11/2 level, resulting in the population of 4F7/2 level of Er3+. Next, the non-radiative relaxation of the Er3+ at the 4F7/2 level leads to the 2H11/2 levels (4F7/2 → 2H11/2 + phonons). Ultimately, the presence of both 2H11/2 and 4S3/2 levels in a state of thermal equilibrium allows for the acquisition of green emissions from these levels (2H11/2, 4S3/2) → 4I15/2 + photon). Additionally, because of the short energy gap between these two energy levels, the excited Er3+ in the 4I11/2 level can nonradiatively relax to the 4I13/2 level via a multi-phonon relaxation process. The Er3+ in 4I13/2 level can absorb energy from another Er3+ in 4I11/2 through an ET process or an ESA process to reach the 4F9/2 level. A 4F9/2 → 4I15/2 transition can subsequently produce the red emission (Fig. 6).48–52
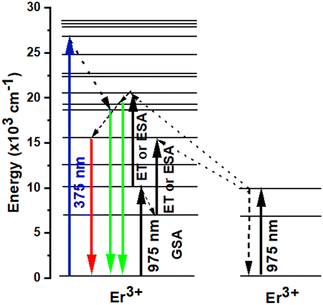 |
| Fig. 6 Energy level diagrams of Er3+ and the possible DC and UC luminescence mechanisms of NSGM: 0.04Er3+ sample under 375 nm and 975 nm excitations. | |
2.3. Photometric analysis. The photometric study explores the color appearance (chromaticity coordinates), color purity (CP) and correlated color temperature (CCT) of the light emitted by the synthesized material. Fig. 7(a) and (b) illustrates the CIE chromaticity representations obtained for (DC) and (UC) of NSGM: 0.04Er3+ at room temperature, respectively. The CIE coordinates are in the green region. The position of the CIE coordinates suggests that the synthesized material has good color purity. Color purity indicates the degree of mono-chromaticity of the light. We also assessed the purity of the colors using the following relationship:40,53 |
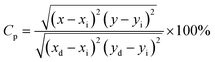 | (5) |
where (x, y), (xi, yi), and (xd, yd) represent color coordinate, white light points and dominant wavelength point, respectively. CCT of phosphor material is equal to the temperature of an ideal body radiator that emits the light of the same chromaticity as the phosphor does. McCamy formula is followed to determine the CCT of the light emitted from the synthesized materials:40,53 |
CCT = −437n3 + 3526n2 − 6823n + 5520.23
| (6) |
where n = (x − xe)/(y − ye) and xe = 0.3320, ye = 0.1858. The computed values for color coordinates and correlated color temperature (CCT) are determined to be approximately (x = 0.276, y = 0.673, and CCT = 6343 K) for DC, and (x = 0.359, y = 0.607, and CCT = 5092 K) for UC. Moreover, the calculated color purity (CP) is found to be approximately 98.76% and 80.74% for DC conversion and UC conversion, respectively. The fact that the CCT values exceed 5000 K and the CP values surpass 80% indicates that the suggested material has the potential to be a green phosphor suitable for cold white-LEDs. Notably, chromaticity coordinates (x, y) of the DC phosphors are most close to pure green emission. The color purity of the sample was estimated and reached a maximum value of 98.76%, indicating the high pure green light emission of the obtained phosphor. Overall, the high color purity of the UC and DC phosphor suggest that it is suitable as a candidate material for optoelectronic devices.
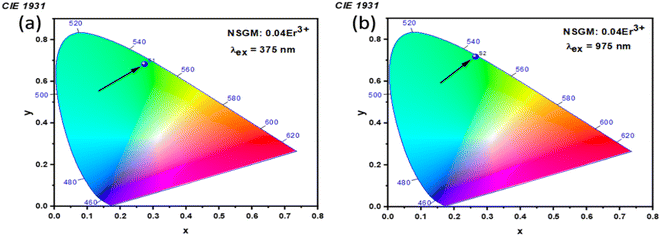 |
| Fig. 7 (a) and (b) Shows the CIE chromatic diagram of NSGM: 0.04Er3+ at excitations of 375 nm and 975 nm, respectively. | |
2.4. Temperature-sensing properties of NSGM: Er3+.
2.4.1. Temperature-dependent DC photoluminescence and temperature sensing. Due to the small energy separation between the thermally coupled 2H11/2 and 4S3/2 levels of Er3+ ions, the 2H11/2 level can be populated from the 4S3/2 level through thermal excitation and reach thermal quasi-equilibrium. This leads to varying FIR values in the green emissions of the 2H11/2 → 4I15/2 and 4S3/2 → 4I15/2 transitions of Er3+ ions at different temperatures.54,55 This characteristic makes luminescent materials based on Er3+ ions suitable for non-invasive optical temperature sensors using the FIR technique. To investigate the feasibility of the synthesized compounds for non-contact thermometry, the temperature-dependent PL emission spectra of NSGM: 0.04Er3+ microcrystal were recorded in the temperature range of 298.15–488.15 K under 375 nm excitation. As shown in the figure, the position of the emission bands remains constant with temperature, while the emission intensities gradually decrease due to thermal quenching effects. It is worth noting that the emission intensity from the 4S3/2 → 4I15/2 transition is stronger than that from the 2H11/2 → 4I15/2 transition at lower absolute temperatures, whereas the opposite phenomenon is observed at higher temperatures (refer to the Fig. 8). This further indicates that the population between the 2H11/2 and 4S3/2 levels can be redistributed at elevated temperatures, and the (2H11/2, 4S3/2) levels are thermally coupled.
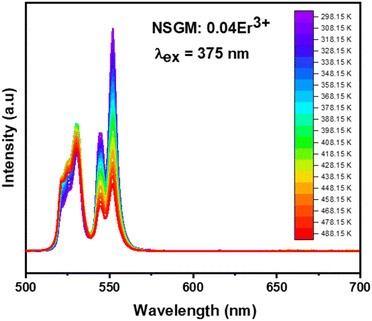 |
| Fig. 8 Temperature-dependent down-conversion photoluminescence emission spectra of NSGM: 0.04 Er3+ under 375 nm excitation. | |
By utilizing the temperature-dependent PL emission spectra, the FIR values of the green emissions were calculated.
The corresponding results are presented in the Fig. 9(a). According to previous literature, the relative population of thermally coupled levels (TCL) (2H11/2, 4S3/2) follows the Boltzmann distribution and their FIR can be expressed as follows:54,56
|
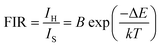 | (7) |
where,
IH and
IS represent the integrated emission intensities for the
4H
11/2 →
4I
15/2 (530 nm) and
4S
3/2 →
4I
15/2 (550 nm) transitions of Er
3+ ions, respectively,
B is a constant, Δ
E is the energy gap between the
4S
3/2 and
2H
11/2 states,
k is the Boltzmann constant and
T is the temperature, Δ
E represents the energy separation between the
2H
11/2 and
4S
3/2 levels,
k is the Boltzmann constant, and
T stands for temperature. The temperature-dependent (FIR
1) shown in the figure demonstrates that FIR values increase with temperature.
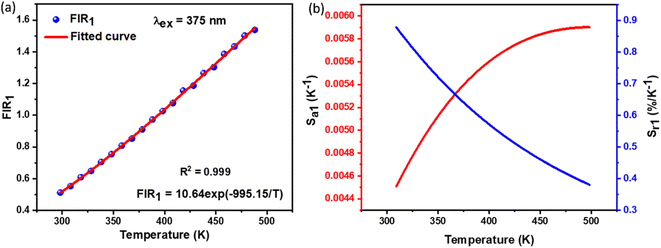 |
| Fig. 9 (a) The determined FIR1 values, (b) the absolute sensitivity and relative sensitivity with temperature of NSGM: 0.04Er3+ based on down-conversion. | |
Furthermore, to determine the feasibility of the synthesized microcrystals for thermometry, it is very important to analyze the relative and absolute sensitivity of the sensor, which is the ratio of change of the FIR with temperature, and which can be defined as follows:54,57
|
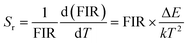 | (8) |
|
 | (9) |
The sensor relative and absolute sensitivity, which was calculated from eqn (8), for the NSGM: 0.04Er3+ microcrystals as a function of temperature is shown in Fig. 10(b) the maximum Sr1 was found for NSGM: 0.04 Er3+ at 298.15 K reaching 0.87% K−1 and the maximum Sa1 was found to be about 0.0059 K−1 at 488.15 K in the temperature range of interest. To determine its usefulness, the sensor sensitivity of the material is compared to that of other RE-doped phosphors (Table 1). As demonstrated in Table 1, the phosphor produced in this study performed well when compared to other host lattices such as phosphate,58 fluoride,55,59 oxide,20,60 and tungsten.61,62 Furthermore, based on the operational temperature range (298.15–488.15 K), NSGM: Er3+ phosphor has a high sensitivity temperature, which is promising for temperature sensor applications.
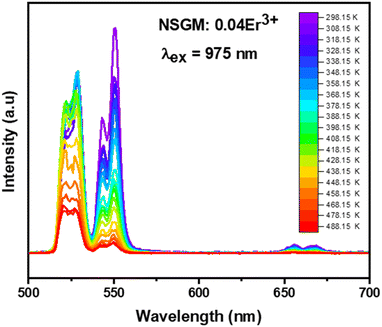 |
| Fig. 10 Temperature-dependent up-conversion photoluminescence emission spectra of NSGM: 0.04 Er3+ under 975 nm excitation. | |
Table 1 Temperature sensitivity comparison of the phosphors
Materials |
Temperature range |
Srmax |
Excitation wavelength |
Ref. |
NaYF4: Er3+/Yb3+ |
93–673 K |
0.0029 K−1 at 368 K |
980 nm |
59 |
YOF: Er3+/Yb3+ |
260–490 K |
0.0060 K−1 at 490 K |
980 nm |
55 |
CaZnOS: Er3+ |
303–603 K |
0.0033 K−1 at 603.15 K |
980 nm |
63 |
YNbO4: Er3+/Yb3+ |
298–673 K |
0.0072 K−1 at 406 K |
980 nm |
20 |
La2MoO6: Er3+ |
303–463 K |
0.0097 K−1 at 460 K |
379 nm |
64 |
La2O3: Er3+/Yb3+ |
303–600 K |
0.0091 K−1 at 303 K |
980 nm |
60 |
Ba5Gd8Zn4O21: Er3+/Yb3+ |
260–490 K |
0.0032 K−1 at 490 K |
980 nm |
65 |
PKAZLF glass: Er3+ |
298–773 K |
0.0079 K−1 at 630 K |
488 nm |
66 |
CaLa2ZnO5: Er3+/Yb3+ |
298–513 K |
0.0059 K−1 at 483 K |
980 nm |
67 |
YVO4: Er3+/Yb3+ |
300–485 K |
0.0116 K−1 at 380 K |
980 nm |
19 |
YNbO4: Er3+/Yb3+ |
298–673 K |
0.0072 K−1 at 406 K |
980 nm |
64 |
AgLa(MoO4)2: Er3+/Yb3+ |
300–510 K |
0.018 K−1 at 480 K |
980 nm |
21 |
NaZnPO4: Er3+/Yb3+/Li+ |
300–603 K |
0.0065 K−1 at 306 K |
980 nm |
58 |
NaZnPO4: Er3+/Yb3+/Ba2+ |
300–523 K |
0.0043 K−1 at 523 K |
970 nm |
58 |
CaWO4: Er3+/Yb3+ |
294–923 K |
0.0092 K−1 at 455 K |
980 nm |
61 |
SrWO4: Er3+/Yb3+ |
300–518 K |
0.014 K−1 at 403 K |
980 nm |
62 |
NSGM: Er3+ |
298.15–488.15 K |
0.0087 K−1 at 298.15 K |
375 nm |
This work |
NSGM: Er3+ |
298.15–488.15 K |
0.0063 K−1 at 298.15 K |
975 nm |
This work |
2.4.2. Temperature-dependent UC photoluminescence and temperature sensing. Fig. 10 demonstrates the UC emission spectra of NSGM: 0.04Er3+ excited by 975 nm in the wavelength region 500–700 nm, measured over a temperature range of 298.15 K to 488.15 K. The position and shape of the emission peaks remain unchanged at different temperatures. However, the emission intensity of peaks at 530 nm and 550 nm reacts differently. The electronic transitions responsible for these emissions are mentioned in the PL DC section and shown in the energy level diagram (Fig. 4(b)). The emission intensity of the 530 nm peak first increases with increasing temperature up, and after that, the emission intensity slowly decreases with increasing temperature. In contrast, the emission intensity of the 550 nm peak decreases steadily with increasing temperature. These patterns of emission intensity variation with temperature differ due to the thermal coupling of the 4H11/2 and 4S3/2 energy levels from which these transitions occur.The ratio of the emission intensities of the thermally coupled energy levels changes with temperature, which is useful for temperature sensing applications. The energy levels 2H11/2 and 4S3/2 follow the Boltzmann distribution so that the fluorescence intensity ratio (FIR2) between them is shown in eqn (7). The Fig. 10(a) shows the plot of the fluorescence intensity ratio (FIR2) of two emission bands at 530 nm and 550 nm as a function of temperature for the NSGM: 0.04Er3+ phosphor. The sensitivity was calculated using (eqn (8)) and the plot is shown in Fig. 10(b). The maximum value of sensitivity relative (Sr2) and absolute (Sa2) are observed to be 0.63% K−1 at 298.15 K and 0.0036 K−1 at 488.15 K, respectively (Fig. 11).
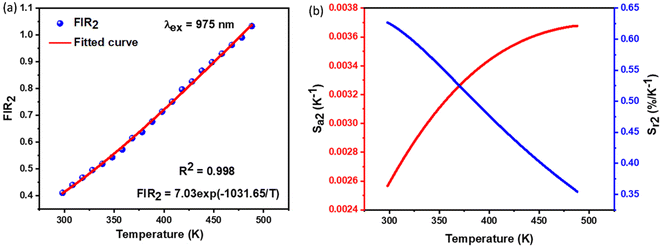 |
| Fig. 11 (a) The determined FIR2 values, (b) the absolute sensitivity and relative sensitivity with temperature of NSGM: 0.04Er3+ based on up-conversion. | |
In summary, both methods showed good performance for temperature detection, presenting high sensitivities compared to published works reported in Table 1, whether using down-conversion or up-conversion. However, down-conversion stood out in terms of relative sensitivity Srmax = 0.87% K−1, indicating that it is more sensitive to temperature variations than up-conversion Srmax = 0.63% K−1.
To assess the reliability of the measurements, the uncertainty in temperature determination (δT) was calculated using the following equation:68
|
 | (10) |
This uncertainty, δFIR, represents the limit of resolution or the relative uncertainty of the thermometric parameter, which is the smallest change in the ratio that can be experimentally observed. It is important to note that the limit of resolution depends on both the thermometer's performance and the characteristics of the experimental setup.47 Therefore, to improve the limit of resolution, enhanced data acquisition methods and improvements in measurement performance can be considered. This could include adjustments such as increasing the integration time and using averaging of successive measurements to reduce experimental noise. To determine the detection limit, measurements were conducted at room temperature under similar conditions for the NSGM: Er3+ phosphor using both UC and DC excitation. The results are presented as histograms in the (Fig. S3(a) and (b)†). The FIR uncertainty (δFIR) was obtained by calculating the standard deviation of the statistical distribution for NSGM: 0.04Er3+, yielding values of 0.0010 and 0.0013 for FIR1 and FIR2, respectively. Using equation (eqn (10)), all δT (Fig. 12(a) and (b)) uncertainty values are below 0.50 K. This indicates that the NSGM: 0.04Er3+ microcrystals exhibit high accuracy across this entire temperature range.
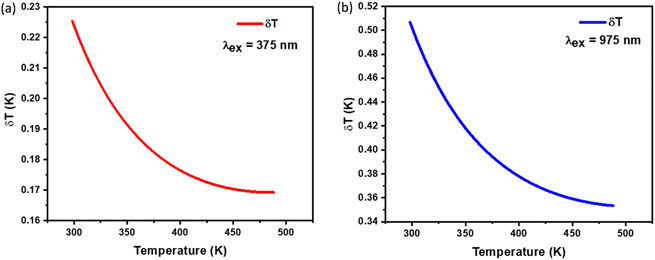 |
| Fig. 12 Temperature resolution values δT, corresponding to (a) FIR1 (DC), (b) FIR2 (UC) for NSGM: 0.04Er3+. | |
The repeatability (R) of the sample must be assessed to establish if the crystals are an effective thermal probe. The following equation may be used to determine this parameter:69
|
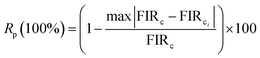 | (11) |
where
Mi(
T)
c is the measured parameter (FIR or band centroid) in the
ith cycle and
M(
T)
cis the mean value of the
M(
T)
c over 10 cycles. The FIR measurement was performed five times in a succession without altering the experimental equipment.
Fig. 13(a) and (b) shows the cycle measurements of NSGM: 0.04Er
3+ generated for emission-based DC and UC, respectively. The calculated value of
R (more than 98%) indicates that the NSGM doped Er
3+ manufactured have acceptable thermal repeatability.
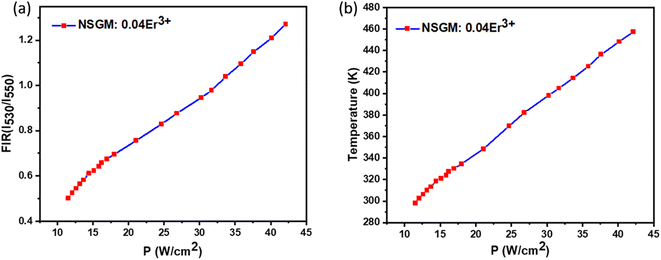 |
| Fig. 13 Repeatability assessment (R) (a) and (b) for NSGM: 0.04Er3+ for (DC) and (UC), respectively. | |
2.5. Optical heating study. In order to investigate the optical heating effect in Er3+ doped NSGM phosphor caused by the laser excitation 975 nm, the green UC emission spectra were recorded in the 500–700 nm range at various excitation power density (11.52 to 42.12 W cm−2) shown in Fig. S4.† The laser beam was focused on the sample surface within 10 mm2 area. Variation in the intensity of 2H11/2/4I15/2 and 4S3/2/4I15/2 transitions is not same for the sample due to the change in their populations in the corresponding levels. This dissimilar variation of two transitions gives rise to the variation of FIR with excitation power. The FIR (IH/IS) was calculated with excitation power density for the sample and the plot is shown in Fig. 14(a).
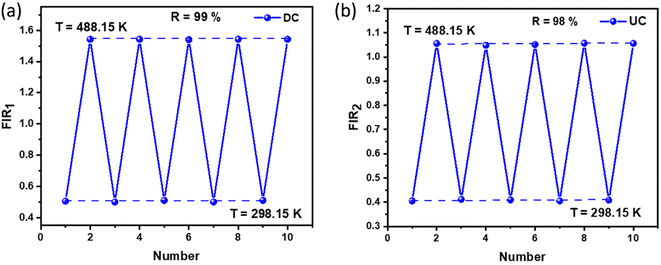 |
| Fig. 14 Evolution of FIR (a) and temperature (b) as a function of power densities under excitation at 975 nm. | |
It is observed that on increasing the power density from 11.52 to 42.12 W cm−2, the FIR value increases. This indicates that internal sample temperature increases with laser power excitation. A larger variation of FIR value indicates larger heating of material. Thus, it is concluded that laser excitation generates heat inside the sample. The variation in temperature as a function of sample power is essentially due to the energy absorbed by the crystalline powders and transferred as heat through non-radiative channels.70 This optical heating generated by laser excitation can be calculated using (eqn (8)), which is transformed into the following form:
|
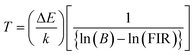 | (12) |
where, all the terms have their usual meanings. Using the Δ
E and
B values obtained from the temperature sensing study (Δ
E = 670.15 cm
−1 and
B = 7.03), along with the FIR values corresponding to various power densities, the temperature increase of the sample was calculated using (
eqn (12)). The sample temperature of the phosphor
versus increasing power density is shown in
Fig. 14(b). The internal sample temperature is found to increase with excitation power, reaches to 298 to 457 K, for Er
3+ doped NSGM for the same pump power variation from 11.52 to 42.12 W cm
−2.
71,72 The heat generated in the sample is due to the non-radiative relaxation process and crystalline nature of the phosphor powders.
73 When energy cannot be fully utilized in radiative transitions, excess energy will lead to phonon-assisted non-radiative transitions, resulting in a rise in temperature.
74 The result suggests that Er
3+ doped NSGM phosphor is capable of transferring the NIR laser excitation pump power into heat and act as an optical heater which could be useful for photo-thermal therapy.
2.6. Conclusion. In summary, Er3+-doped (NSGM) green phosphors were successfully synthesized through the standard solid-state reaction method. XRD analysis confirmed the formation of a well-crystalline tetragonal phase of NSGM: Er3+ with the I41/a space group (88), and SEM analysis revealed an average particle size of approximately 2 μm. Absorption measurements indicated the formation of the molybdate host, evidenced by the characteristic band of MoO42− groups in the visible region. The energy gap values for NSGM: 0.04 Er3+ were determined to be 3.59 eV. Under excitation at 375 and 975 nm, the dominant peaks at 530 and 550 nm corresponded to the 2H11/2, 4S3/2 → 4I15/2 transitions, resulting in green light emission. Chromaticity parameter studies indicated a thermally stable cold emission in the green spectrum, with color purities of approximately 98.76% and 80.74% for DC and UC conversion, respectively. Furthermore, the DC and UC temperature-sensing properties of the phosphors were examined by analyzing the fluorescence intensity ratio between the 2H11/2, 4S3/2 → 4I15/2 transitions within the temperature range of 298.15 to 488.15 K. NSGM: 0.04Er3+ demonstrated higher sensitivity to temperature, with a maximum relative sensitivity of 0.87%/K−1 under 375 nm light excitation. Conversely, under 975 nm light excitation, NSGM: 0.04Er3+ exhibited a maximum relative sensitivity of 0.63%/K−1 within the same temperature range. Luminescence thermometry showcased outstanding accuracy in temperature measurement, with a calculated temperature uncertainty (δT) of less than 0.50 K and repeatability (R) exceeding 98%. This exceptional precision highlights the potential of luminescence thermometry as a high-precision optical sensing technique. These results position this material as promising candidate for temperature sensing applications. Additionally, laser-induced optical heating inside the sample was quantified using the appropriate formula, revealing a temperature increase of around 457 K with an increased power density of up to 42.12 W cm−2. This observation suggests that NSGM: 0.04Er3+ holds promise for photo-thermal therapy.
Conflicts of interest
There are no conflicts to declare.
References
- K. Saidi, C. Hernández-Álvarez, M. Runowski, M. Dammak and I. Rafael Martín Benenzuela, ACS Appl. Nano Mater., 2023, 6, 19431–19442 CrossRef CAS.
- R. Gopal and J. Manam, Appl. Phys. A, 2022, 128, 772 CrossRef CAS.
- A. Kumar and J. Manam, Ceram. Int., 2022, 48, 13615–13625 CrossRef CAS.
- Z. E. A. A. Taleb, K. Saidi and M. Dammak, Dalton Trans., 2023, 52, 18069–18081 RSC.
- B. P. Singh, A. K. Parchur, R. S. Ningthoujam, A. A. Ansari, P. Singh and S. B. Rai, Dalton Trans., 2014, 43, 4770–4778 RSC.
- Z. E. A. A. Taleb, K. Saidi, M. Dammak, D. Przybylska and T. Grzyb, Dalton Trans., 2023, 52, 4954–4963 RSC.
- S. Sinha, M. K. Mahata, K. Kumar, S. P. Tiwari and V. K. Rai, Spectrochim. Acta, Part A, 2017, 173, 369–375 CrossRef CAS.
- Y. Jin, J. Zhang, S. Lü, H. Zhao, X. Zhang and X. Wang, J. Phys. Chem. C, 2008, 112, 5860–5864 CrossRef CAS.
- J. H. Chung, J. H. Ryu, J. W. Eun, J. H. Lee, S. Y. Lee, T. H. Heo, B. G. Choi and K. B. Shim, J. Alloys Compd., 2012, 522, 30–34 CrossRef CAS.
- Y. Zhou, X.-H. He and B. Yan, Opt. Mater., 2014, 36, 602–607 CrossRef CAS.
- S. Vidya, S. Solomon and K. Thomas, Phys. Status Solidi, 2012, 209, 1067–1074 CrossRef CAS , accessed December 13, 2023..
- C. Hernández-Álvarez, G. Brito-Santos, I. R. Martín, J. Sanchiz, K. Saidi, K. Soler-Carracedo, Ł. Marciniak and M. Runowski, J. Mater. Chem. C, 2023, 11, 10221–10229 RSC.
- X. Liu, J. Wang, R. Lei, S. Zhao, F. Huang, D. Deng and S. Xu, Sens. Actuators, A, 2020, 315, 112287 CrossRef CAS.
- K. Saidi, M. Dammak, K. Soler-Carracedo and I. R. Martín, J. Alloys Compd., 2022, 891, 161993 CrossRef CAS.
- A. Torquato, R. A. de Oliveira, T. O. Sales, G. Y. Poirier, G. Batista, F. C. Cassanjes, C. Jacinto and M. R. Dousti, Opt. Mater., 2021, 112, 110723 CrossRef CAS.
- S. Li, W. Wang, X. Wei, L. Li, Q. Zhang, Y. Li and Y. Pan, Opt. Mater., 2021, 113, 110840 CrossRef CAS.
- K. Saidi, I. Kachou, K. Soler-Carracedo, M. Dammak and I. R. Martín, ACS Appl. Nano Mater., 2023, 6, 17681–17690 CrossRef CAS.
- M. K. Mahata, K. Kumar and V. K. Rai, Spectrochim. Acta, Part A, 2014, 124, 285–291 CrossRef CAS.
- M. K. Mahata, K. Kumar and V. Kr. Rai, Sens. Actuators, B, 2015, 209, 775–780 CrossRef CAS.
- Y. Tian, Y. Tian, P. Huang, L. Wang, Q. Shi and C. Cui, Chem. Eng. J., 2016, 297, 26–34 CrossRef CAS.
- T. Li, C. Guo, S. Zhou, C. Duan and M. Yin, J. Am. Ceram. Soc., 2015, 98, 2812–2816 CrossRef CAS.
- Y. K. Kshetri, B. Chaudhary, T.-H. Kim, H. S. Kim and S. W. Lee, J. Eur. Ceram. Soc., 2021, 41, 2400–2406 CrossRef CAS.
- H. Gong, X. Peng, G. A. Ashraf, F. Hu, R. Wei and H. Guo, Ceram. Int., 2022, 48, 4023–4030 CrossRef CAS.
- J. Hu, X. Zhang, H. Zheng, F. Lu, X. Peng, R. Wei, F. Hu and H. Guo, Ceram. Int., 2022, 48, 3051–3058 CrossRef CAS.
- L. Li, P. Yang, W. Xia, Y. Wang, F. Ling, Z. Cao, S. Jiang, G. Xiang, X. Zhou and Y. Wang, Ceram. Int., 2021, 47, 769–775 CrossRef CAS.
- Q. Zhang, L. Li, F. Liu, S. Li, X. Wei, W. Wang, H. Chen, Y. Pan and Y. Li, J. Solid State Chem., 2022, 308, 122927 CrossRef CAS.
- M. Rajendran and S. Vaidyanathan, New J. Chem., 2020, 44, 5354–5365 RSC.
- Y. Jin, J. Zhang, S. Lü, H. Zhao, X. Zhang and X. Wang, J. Phys. Chem. C, 2008, 112, 5860–5864 CrossRef CAS.
- S. Sinha, M. K. Mahata, H. C. Swart, A. Kumar and K. Kumar, New J. Chem., 2017, 41, 5362–5372 RSC.
- M. Rajendran, R. Devi, S. Mund, K. Singh and S. Vaidyanathan, J. Mater. Chem. C, 2021, 9, 15034–15046 RSC.
- L. Li, Y. Pan, X. Zhou, C. Zhao, Y. Wang, S. Jiang, A. Suchocki and M. G. Brik, J. Alloys Compd., 2016, 685, 917–926 CrossRef CAS.
- B. Han, J. Zhang, Z. Wang, Y. Liu and H. Shi, J. Lumin., 2014, 149, 150–154 CrossRef CAS.
- X. Chai, J. Li, Y. Zhang, X. Wang, Y. Li and X. Yao, RSC Adv., 2016, 6, 64072–64078 RSC.
- G. S. R. Raju, E. Pavitra and J. S. Yu, Dalton Trans., 2014, 43, 9766–9776 RSC.
- S. Zeng, H. Wang, W. Lu, Z. Yi, L. Rao, H. Liu and J. Hao, Biomaterials, 2014, 35, 2934–2941 CrossRef CAS.
- X. Huang, J. Mater. Sci., 2016, 51, 3490–3499 CrossRef CAS.
- P. Du, L. Luo and J. S. Yu, Ceram. Int., 2016, 42, 5635–5641 CrossRef CAS.
- A. Verma and S. K. Sharma, Ceram. Int., 2017, 43, 8879–8885 CrossRef CAS.
- S. Sinha and K. Kumar, Opt. Mater., 2018, 75, 770–780 CrossRef CAS.
- A. Kumar and J. Manam, J. Alloys Compd., 2020, 829, 154610 CrossRef CAS.
- P. Rohilla and A. S. Rao, Mater. Res. Bull., 2022, 150, 111753 CrossRef CAS.
- M. K. Pradhan and S. Dash, J. Rare Earths, 2022, 40, 1837–1848 CrossRef CAS.
- R. S. Yadav, M. Rai, S. B. Rai and S. J. Dhoble, Prog. Solid State Chem., 2020, 57, 100267 CrossRef CAS.
- J. Yang, P. He, Y. Xie, Q. Chen, Q. Dong, W. Nie, F. Yang, W. Wang, F. Du, J. Peng and X. Ye, J. Alloys Compd., 2022, 903, 163815 CrossRef CAS.
- X. Feng, F. Long, B. Wang, Z. Weng, X. Wang, Y. Li and H. Ju, Inorg. Chem. Commun., 2022, 137, 109180 CrossRef CAS.
- M. Runowski, A. Shyichuk, A. Tymiński, T. Grzyb, V. Lavín and S. Lis, ACS Appl. Mater. Interfaces, 2018, 10, 17269–17279 CrossRef CAS.
- K. Soler-Carracedo, I. R. Martín, F. Lahoz, H. C. Vasconcelos, A. D. Lozano-Gorrín, L. L. Martín and F. Paz-Buclatin, J. Alloys Compd., 2020, 847, 156541 CrossRef CAS.
- S. Ryszczyńska, K. Trejgis, Ł. Marciniak and T. Grzyb, ACS Appl. Nano Mater., 2021, 4, 10438–10448 CrossRef.
- K. Annapoorani, N. Suriya Murthy, T. R. Ravindran and K. Marimuthu, J. Lumin., 2016, 171, 19–26 CrossRef CAS.
- X. Wang, X. Li, L. Cheng, S. Xu, J. Sun, J. Zhang, X. Zhang, X. Yang and B. Chen, RSC Adv., 2017, 7, 23751–23758 RSC.
- D. L. Silva, R. S. Pugina and J. M. Almeida Caiut, J. Lumin., 2022, 243, 118639 CrossRef CAS.
- C. Zhao, Y. Gao, T. Song, J. Wang and J. Qiu, J. Phys. Chem. Lett., 2023, 14, 9011–9018 CrossRef CAS.
- A. Kumar and J. Manam, Opt. Mater., 2019, 96, 109373 CrossRef CAS.
- B. Dong, B. Cao, Y. He, Z. Liu, Z. Li and Z. Feng, Adv. Mater., 2012, 24, 1987–1993 CrossRef CAS PubMed.
- H. Suo, C. Guo, J. Zheng, B. Zhou, C. Ma, X. Zhao, T. Li, P. Guo and E. M. Goldys, ACS Appl. Mater. Interfaces, 2016, 8, 30312–30319 CrossRef CAS.
- D. Chen, Z. Wang, Y. Zhou, P. Huang and Z. Ji, J. Alloys Compd., 2015, 646, 339–344 CrossRef CAS.
- C. D. S. Brites, P. P. Lima, N. J. O. Silva, A. Millán, V. S. Amaral, F. Palacio and L. D. Carlos, Nanoscale, 2012, 4, 4799–4829 RSC.
- L. Mukhopadhyay, V. K. Rai, R. Bokolia and K. Sreenivas, J. Lumin., 2017, 187, 368–377 CrossRef CAS.
- P. Du, L. Luo and J. Su Yu, RSC Adv., 2016, 6, 94539–94546 RSC.
- R. Dey and V. K. Rai, Dalton Trans., 2014, 43, 111–118 RSC.
- W. Xu, X. Gao, L. Zheng, P. Wang, Z. Zhang and W. Cao, Appl. Phys. Express, 2012, 5, 072201 CrossRef.
- A. Pandey, V. K. Rai, V. Kumar, V. Kumar and H. C. Swart, Sens. Actuators, B, 2015, 209, 352–358 CrossRef CAS.
- H. Zhang, D. Peng, W. Wang, L. Dong and C. Pan, J. Phys. Chem. C, 2015, 119, 28136–28142 CrossRef CAS.
- P. Du and J. S. Yu, Chem. Eng. J., 2017, 327, 109–119 CrossRef CAS.
- H. Suo, C. Guo and T. Li, J. Phys. Chem. C, 2016, 120, 2914–2924 CrossRef CAS.
- N. Vijaya, P. Babu, V. Venkatramu, C. K. Jayasankar, S. F. León-Luis, U. R. Rodríguez-Mendoza, I. R. Martín and V. Lavín, Sens. Actuators, B, 2013, 186, 156–164 CrossRef CAS.
- L. Li, C. Guo, S. Jiang, D. K. Agrawal and T. Li, RSC Adv., 2014, 4, 6391 RSC.
- K. Saidi, W. Chaabani and M. Dammak, RSC Adv., 2021, 11, 30926–30936 RSC.
- J. Zhu, T. Yang, H. Li, Y. Xiang, R. Song, H. Zhang and B. Wang, Chem. Eng. J., 2023, 471, 144550 CrossRef CAS.
- R. Dey, A. Pandey and V. K. Rai, Sens. Actuators, B, 2014, 190, 512–515 CrossRef CAS.
- K. Nigoghossian, S. Ouellet, J. Plain, Y. Messaddeq, D. Boudreau and S. J. L. Ribeiro, J. Mater. Chem. B, 2017, 5, 7109–7117 RSC.
- S. Rohani, M. Quintanilla, S. Tuccio, F. De Angelis, E. Cantelar, A. O. Govorov, L. Razzari and F. Vetrone, Adv. Opt. Mater., 2015, 3, 1606–1613 CrossRef CAS.
- Q. Qiang and Y. Wang, Front. Chem., 2019, 7, 425 CrossRef CAS.
- S. Xiang, B. Chen, J. Zhang, X. Li, J. Sun, H. Zheng, Z. Wu, H. Zhong, H. Yu and H. Xia, Opt. Mater. Express, 2014, 4, 1966–1980 CrossRef CAS.
|
This journal is © The Royal Society of Chemistry 2024 |