DOI:
10.1039/D4RA00550C
(Paper)
RSC Adv., 2024,
14, 13905-13914
The crucial role of hydrogen bonding in shaping the structures of zinc-based coordination polymers using tridentate N, N, O donor reduced Schiff base ligands and bridging acetates†
Received
22nd January 2024
, Accepted 23rd April 2024
First published on 26th April 2024
Abstract
In this manuscript we report the synthesis and characterization of two new polynuclear zinc(II) complexes, [Zn2L1(μ-OAc)3]n·H2O (1) and [Zn2L2(μ-OAc)3]n (2) using two tridentate ligands, HL1 {4-chloro-2-(((2-(methylamino)ethyl)amino)methyl)phenol} and HL2 {2,4-dibromo-6-(((3-(methylamino)propyl)amino)methyl)phenol}. The structures were confirmed by single-crystal X-ray diffraction analysis. Both complexes form 1D chains. The energy of H-bonding interaction in the solid state structures of the complexes has been estimated by DFT calculation and the crucial role of hydrogen bonding in shaping their structures has been highlighted.
Introduction
Schiff base ligands, characterized by imine bonds, are of interest to inorganic chemists for their wide ranging complexing ability and stability under various oxidative and reductive conditions. Their structural versatility is associated with their diverse applications since long.1–6 They have been used to prepare numerous transition and non-transition metal complexes, many of which have interesting applications in materials science.7–11 Many such complexes found application in biochemistry,12 catalysis,13 medical imaging,14 non-linear optics,15,16 and opto-electronics.17,18 The application of these complexes in magetochemistry is also noteworthy.19–23 Among various Schiff bases, salen type N2O2 donor ligands, prepared by the 1
:
2 condensation of ethylenediamine and salicylaldehyde, are probably most popular.24–32 Use of N-substituted diamine may produce tridentate N2O donor Schiff bases and may also be used for forming varieties of complexes.33–38 These ligands could be reduced with mild reducing agents (e.g. sodium borohydride) to form more flexible ‘reduced Schiff bases’, which may form complexes with various transition and non-transition metals, which have also been shown to have different interesting applications in catalysis, sending and magnetism.39–44
Formation of different supramolecular assemblies in the solid state structures of different transition and non-transition metal complexes may be tactfully controlled by exploiting various non-covalent interactions.45–47 The application of these supramolecular structures in host–guest chemistry, in sensing, and in catalysis is well documented in literature.48–50 Therefore, a good command over several branches of supramolecular chemistry may be achieved by the systematic study of these non-covalent interactions. The most frequently used methodology to design supramolecular structure in complexes is to employ hydrogen bonds.51–53 A hydrogen bond is nothing but an interaction of two electronegative atoms with an H atom. The energy of interaction associated with the formation of a hydrogen bond falls approximately in range of 0.25–40 kcal mol−1.54 It is well known since its discovery that the increasing electronegativity of the interacting atoms increases the energy of H-bonding interaction.55 Owing to the directional nature and strength hydrogen bonding interaction, crystal engineers frequently used it for the association of discrete molecules into well-organized supramolecules.56–70
In the present work, two structurally similar reduced Schiff base ligands have been synthesized and used to form zinc(II) complexes. Supramolecular interactions in the solid state structures of both complexes were studied energetically using theoretical DFT calculations to understand clearly the importance of hydrogen bonding in shaping the structures. These interactions have also been analyzed using several computational tools, including Bader's “atoms-inmolecules” (AIM).
Experimental
All starting materials and solvents were commercially available, reagent grade, and used as purchased from Sigma-Aldrich without further purification.
Synthesis of the ligands
The ligands have been prepared following the literature methods71,72 and therefore the synthesis of the ligands is briefly given in the ESI.†
Synthesis of complexes
[Zn2L1(μ-OAc)3]n·H2O (1). A methanol solution (5 mL) of ∼2 mmol zinc(II) acetate dihydrate (439 mg) was added to a methanol solution (10 mL) of the reduced ‘Schiff base’ ligand HL1 (∼1 mmol) with constant stirring. Colourless crystals of the complex 1 were obtained after ten days of slow evaporation in an open atmosphere.Yield: 435.44 mg (∼81%) based on zinc. Anal. Calc. for C16H23ClN2O8Zn2 (FW: 537.59): C, 35.75; H, 4.31; N, 5.21. Found: C, 35.72; H, 4.33; N, 5.24%, FT-IR (KBr, cm−1): 3304–3241 (νN–H), 2924–2864 (νC–H), 1607–1567 (νCOO−), 1434–1390 (νCOO−). UV-vis, λmax (nm), [εmax (L mol−1 cm−1)] (acetonitrile): 292 (2.817 × 103).
[Zn2L2(μ-OAc)3]n (2). Complex 2 was synthesized in a similar procedure to that of complex 1, except that HL2 was used instead of HL1.Yield: 487.63 mg (∼74%) based on zinc. Anal. Calc. for C17H24Br2N2O7Zn2 (FW: 658.96): C, 30.99; H, 3.67; N, 4.25. Found: C, 31.10; H, 3.71; N, 4.32%, FT-IR (KBr, cm−1): 3261–3220 (νN–H), 2960–2863 (νC–H), 1605–1562 (νCOO−), 1405 (νCOO−). UV-vis, λmax (nm), [εmax (L mol−1 cm−1)] (acetonitrile): 309 (5.18 × 103).
Physical measurement
Elemental analysis (carbon, hydrogen and nitrogen) was performed using a PerkinElmer 240C elemental analyzer. IR spectra in KBr (4500–500 cm−1) were recorded RX-1 FTIR spectrophotometer. Absorption spectra in acetonitrile solvent were recorded on UV-1900i Shimadzu absorbance spectrophotometer. Emission spectra were recorded in acetonitrile solvent on Duetta-1621 fluorescence spectrophotometer.
X-ray crystallography
A ‘Bruker D8 QUEST area detector’ diffractometer equipped with graphite-monochromated Mo Kα radiation (λ = 0.71073 Å) has been used to collect (single crystal) XRD data using suitable crystals (of appropriate dimension) of the complexes. Direct method has been used to solve the structures and refinement was done by full-matrix least squares on F2 using the SHELXL-18/1 package.73 Non-hydrogen atoms were refined with anisotropic thermal parameters. The hydrogen atoms attached to nitrogen atoms were located by difference Fourier maps and were kept at fixed positions. All other hydrogen atoms were placed in their geometrically idealized positions and constrained to ride on their parent atoms. Multi-scan empirical absorption corrections were applied to the data using the program SADABS.74 The details of crystallographic data and refinements have been given in Table S1.† Important bond lengths and angles have been listed in Tables S2 and S3, respectively (ESI).†
Theoretical methods
The H-bonding interactions in this study were analyzed using Gaussian-16 software75 and the PBE0-D3/def2-TZVP level of theory.76–78 For evaluating these interactions in their solid-state form, we utilized crystallographic coordinates. The Bader's “Atoms in Molecules” theory (QTAIM)79,80 was employed for examining the hydrogen bonding interactions, utilizing the AIMAll calculation package for these analyses.81 The hydrogen bonding interaction has been estimated using the potential energy density (Vg) at the BCP and the equation proposed in the literature.82
Results and discussion
Synthesis
A methanol solution of N-methyl-1,2-diaminoethane or N-methyl-1,3-diaminopropane and 5-chlorosalicylaldehyde or 3,5-dibromosalicylaldehyde in 1
:
1 molar ratio were refluxed to produce tridentate N2O donor Schiff base ligands, following the literature method.83–90 NaBH4 was then used as a reducing agent to prepare the reduced analogues (HL1 and HL2) of the Schiff base ligands.71,72 These are essentially secondary amines, but may be considered as a ‘reduced Schiff base’ to highlight the method of its synthesis. HL1 and HL2 on reaction with zinc(II) acetate dihydrate in 1
:
2 molar ratio in presence of few drops of methanol to produce the complexes 1 and 2. Synthetic routes to complexes have been shown in Schemes 1 and 2.
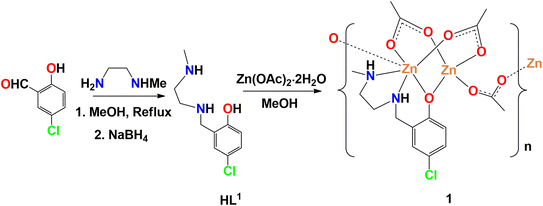 |
| Scheme 1 Synthetic route to complex 1. Lattice water is not shown for clarity. | |
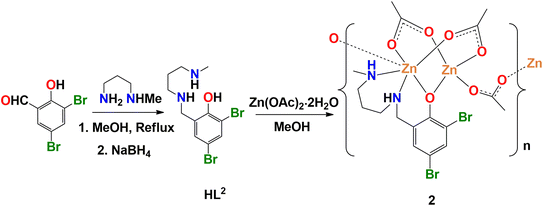 |
| Scheme 2 Synthetic route to complex 2. | |
Description of structures
[Zn2L1(μ-OAc)3]n·H2O (1). X-ray crystal structure determination reveals that the complex 1 crystalizes in orthorhombic space group, Fdd2 forming a chain structure, as shown in Fig. 1. The asymmetric unit (Fig. 2) of complex 1 contains two different types of zinc(II) centers, distorted octahedral, Zn(1) and distorted tetrahedral, Zn(2) along with a lattice water molecule. Zn(1), is equatorially coordinated by two amine nitrogen atoms, {N(1) and N(2)}, one phenoxo oxygen atom, O(1), of the N2O reduced Schiff base unit, (L1)− and one bridging acetate oxygen atom, O(4), and axially coordinated by two oxygen atoms, O(2) and O(7)i (i = symmetry transformation = −1/4 + x, 1.25 − y, −1/4 + z) of two bridging acetate units. Zn(2), On the other hand is coordinated by one phenoxo oxygen atom, O(1), of the reduced Schiff base unit (L1)− and three oxygen atoms, {O(3), O(5) and O(6)} of three bridging acetate units. The most common geometry of any tetra-coordinated complex is either square planar or tetrahedral. The exact geometry could be calculated from the τ4 index, which is defined as τ4 = {360−(a + b)}/141, where a and b are the two largest ligand–metal–ligand angles in the four coordinate species.91 For a perfect square planar system, τ4 index should be equal to 0. Similarly, for a regular tetrahedral geometry, τ4 index is very close to 1. The τ4 index of complex 1 is 0.885, which confirms the distorted tetrahedral geometry of Zn(2) center. The two largest ligand–metal–ligand angles are 120.6(2)° [O(6)–Zn(2)–O(3)] and 114.6(2)° [O(5)–Zn(2)–O(3)] around Zn(2) center. The saturated five member chelate ring [Zn(1)–N(1)–C(8)–C(9)–N(2)], represent a half-chair conformation (Fig. 3) with puckering parameters, q = 0.473(7) Å; ϕ = 263.5(6)°.92
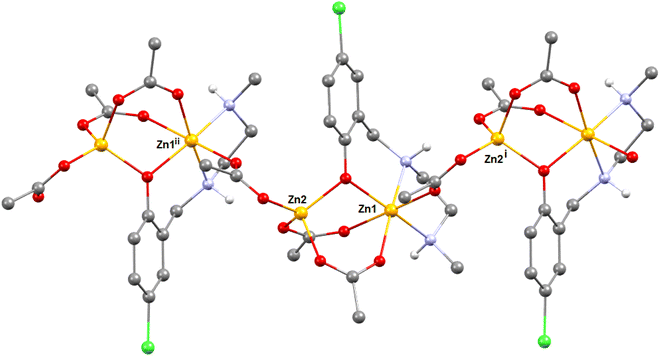 |
| Fig. 1 1D chain of complex 1. Only amine hydrogen atoms are shown for clarity. Symmetry transformation i = −1/4 + x, 1.25 − y, −1/4 + z and ii = 1/4 + x, 1.25 − y, 1/4 + z. We have maintained a colour codes in this figure: red for oxygen, grey for carbon, light blue for nitrogen, green for chlorine, light grey for hydrogen and orange for zinc centres. | |
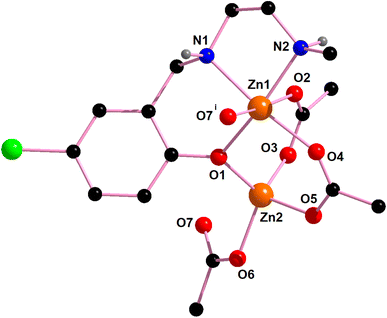 |
| Fig. 2 The asymmetric unit of complex 1. Lattice water molecule is not shown for clarity. Only the relevant hydrogen atoms are shown for clarity. Symmetry transformation i = −1/4 + x, 1.25 − y, −1/4 + z. | |
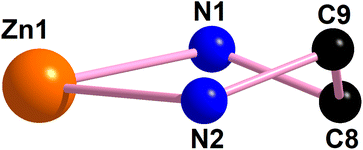 |
| Fig. 3 The perspective view of the half-chair conformation of the saturated five-member chelate ring [Zn(1)–N(1)–C(8)–C(9)–N(2)] of complex 1. | |
In complex 1, there are two N–H⋯O hydrogen bonding interactions involving the lattice water molecule and neighboring monomeric unit. The hydrogen atom, H(1), attached to the amine nitrogen atom, N(1), forms hydrogen bonds (Fig. 4) with the acetate oxygen atom, O(3)i of the neighboring monomeric unit (symmetry transformation i = −1/4 + x, 1.25 − y, 1/4 + z). The hydrogen atom, H(2) attached to the amine nitrogen atom, N(2), of the reduced Schiff base unit, (L1)− forms hydrogen bonds (Fig. 4) with the oxygen atom, O(8), of the lattice water molecule. The details of hydrogen bonding interactions are gathered in Table 1.
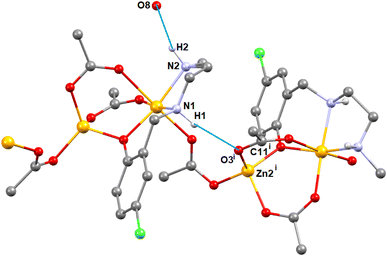 |
| Fig. 4 The N–H⋯O hydrogen bonding interactions in complex 1. Only the relevant hydrogen atoms are shown for clarity. Symmetry transformation i = −1/4 + x, 1.25 − y, −1/4 + z. We have maintained a colour codes in this Fig.: red for oxygen, grey for carbon, light blue for nitrogen, green for chlorine, light grey for hydrogen and orange for zinc centres. | |
Table 1 Hydrogen bond distances and angles in complexes 1–2
Complex |
N–H⋯O |
N–H (Å) |
H⋯O (Å) |
N–H⋯O (Å) |
∠N–H⋯ O (°) |
Symmetry transformation |
1 |
N(1)–H(1)⋯O(3)i |
0.980 |
2.183 |
3.153(7) |
170.2 |
i = −1/4 + x, 1.25 − y, −1/4 + z |
N(2)–H(2)⋯O(8) |
0.981 |
2.117 |
3.04(1) |
155.3 |
— |
2 |
N(1)–H(1)⋯O(5)iii |
0.980 |
2.070 |
3.03(1) |
166.7 |
iii = −0.5 + x, 1.5 − y, z |
N(2)–H(2)⋯O(3)iii |
0.980 |
2.312 |
3.26(1) |
162.7 |
[Zn2L2(μ-OAc)3]n (2). X-ray crystal structure determination reveals that the complex 2 crystalizes in orthorhombic space group, Pba2 forming a chain structure, as shown in Fig. 5. The asymmetric unit (Fig. 6) of complex 2 contains two different types of zinc(II) centers, distorted octahedral, Zn(1) and distorted tetrahedral, Zn(2). Zn(1), is equatorially coordinated by two amine nitrogen atoms, {N(1) and N(2)}, one phenoxo oxygen atom, O(1), of the N2O reduced Schiff base unit, (L2)− and one bridging acetate oxygen atom, O(4), and axially coordinated by two oxygen atoms, {O(2) and O(7)}of two bridging acetate units. Zn(2), On the other hand is coordinated by one phenoxo oxygen atom, O(1), of the reduced Schiff base unit (L2)− and three oxygen atoms, {O(3), O(5) and O(6)} of three bridging acetate units. The τ4 index of the complex 2 is 0.928, which confirms the distorted tetrahedral that the geometry of Zn(2) center. The two largest ligand–metal–ligand angles are 120.1(3)° [O(6)–Zn(2)–O(5)] and 109.1(4)° [O(5)–Zn(2)–O(3)] around Zn(2) center. The saturated six member chelate ring [Zn(1)–N(1)–C(8)–C(9)–C(10)–N(2)], represent a chair conformation (Fig. 7) with puckering parameters, q = 0.599(14) Å; θ = 171.5(12)°; ϕ = 341(9)°.92,93
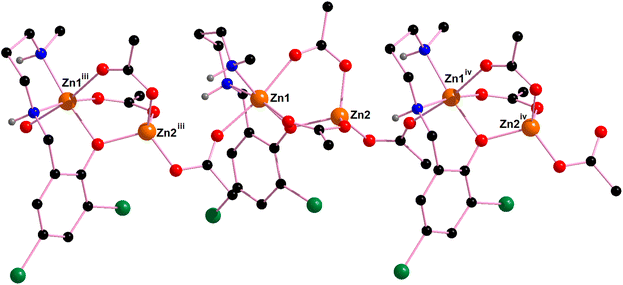 |
| Fig. 5 1D chain of the complex 2. Only amine hydrogen atoms are shown for clarity. Symmetry transformation iii = −0.5 + x, 1.5 − y, z and iv = 0.5 + x, 1.5 − y, z. We have maintained a colour codes in this figure: red for oxygen, black for carbon, blue for nitrogen, deep green for bromine, grey for hydrogen and orange for zinc centres. | |
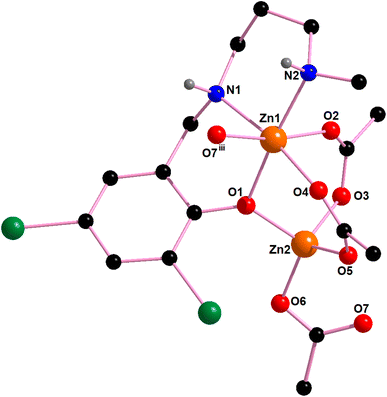 |
| Fig. 6 The asymmetric unit of complex 2. Only the relevant hydrogen atoms are shown for clarity. Symmetry transformation iii = −0.5 + x, 1.5 − y, z. | |
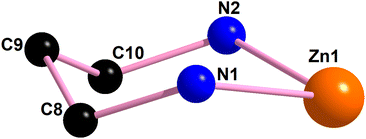 |
| Fig. 7 The perspective view of the chair conformation of the saturated six member chelate ring [Zn(1)–N(1)–C(8)–C(9)–C(10)–N(2)] of complex 2. | |
In complex 2, additionally, two N–H⋯O hydrogen bonding interaction exist between the adjacent monomeric units. The hydrogen atoms, H(1) and H(2), attached to the amine nitrogen atoms, N(1) and N(2) of the reduced Schiff base unit, (L2)− forms hydrogen bonds (Fig. 8) with the acetate oxygen atoms, O(5)iii and O(3)iii of the neighbouring monomeric unit (symmetry transformation = iii = −1/4 + x, 1.25 − y, 1/4 + z). The details of hydrogen bonding interactions are gathered in Table 1.
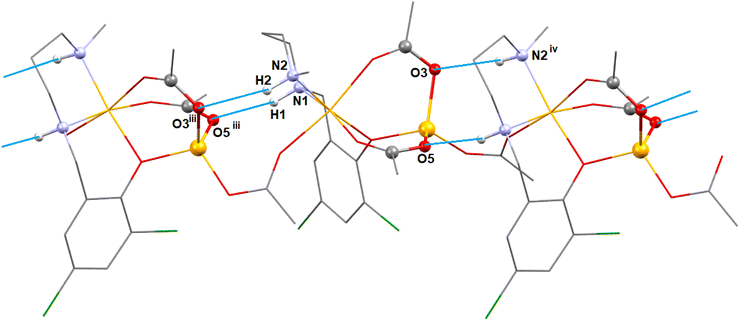 |
| Fig. 8 The N–H⋯O hydrogen bonding interactions in complex 2. Only the relevant hydrogen atoms are shown for clarity. | |
Theoretical study
Two novel coordination polymers based on Zn(II) have been successfully synthesized, as depicted in Fig. 9. These complexes can be described as dinuclear Zn(II) motifs with the formula [Zn2Ln(AcO)3], connected by bridging acetate ligands. In the case of complex 1, which includes a lattice water molecule within its composition, the NH amino groups of the reduced Schiff base ligand exhibit divergent orientations (as indicated by the yellow circle). One NH group points towards the water molecule, while the other NH group interacts with the oxygen atom of an acetate group from an adjacent [Zn2Ln(AcO)3] unit.
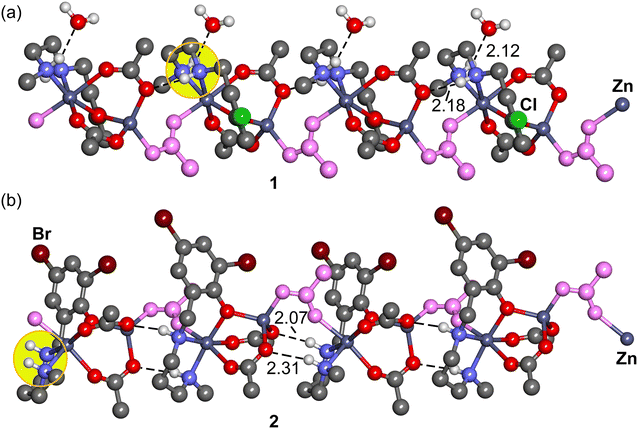 |
| Fig. 9 Representation of the 1D coordination polymers 1 (a) and 2 (b) with indication of the H-bonds. The H-bonded are omitted apart from those participating in the H-bonds. The distances are given in A. | |
In contrast, complex 2 displays a different arrangement, with both NH groups oriented in the same direction. These NH groups form hydrogen bonds with the acetate ligands of neighboring units. Consequently, NH⋯OAc hydrogen bonds play a significant role in both the formation and stabilization of the coordination polymers.
Theoretical Density Functional Theory (DFT) analysis is employed to assess the strength of these hydrogen bonds and to investigate the profound impact of the lattice water molecule on the orientation of the NH groups. This, in turn, influences the geometry and arrangement of the polymeric chain.
We conducted QTAIM (Quantum Theory of Atoms in Molecules) analysis on a dimeric fragment of the polymers, represented as tetranuclear models, to assess the strength of the hydrogen bonds. Given the polymeric nature of the structures, the hydrogen bonds were evaluated using the potential energy density at the bond critical points (CPs), as described in the theoretical methods. The QTAIM analysis confirms the presence of NH⋯OH2 and NH⋯OAc hydrogen bonds, which is evident from the existence of corresponding bond critical points (BCP) represented as purple spheres in Fig. 10, as well as bond paths (depicted as dashed bonds) connecting the hydrogen atoms to the oxygen atoms.
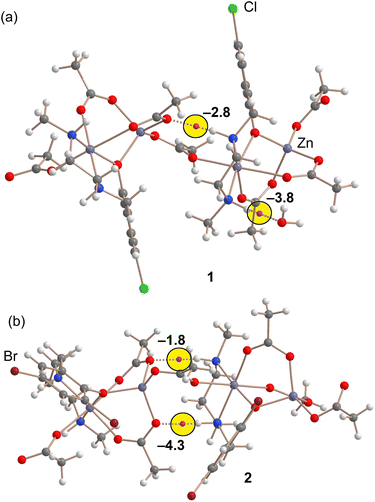 |
| Fig. 10 QTAIM analysis of dimeric models of complexes 1 (a) and 2 (b). Only BCPs (purple spheres) and bond paths (dashed bonds) corresponding to the NH⋯O H-bonds are represented for clarity. The H-bonding energies are indicated close to the BCPs. We have maintained a colour codes in this figure: red for oxygen, grey for carbon and zinc, blue for nitrogen, green for chlorine, purple for bromine and light grey for hydrogen atoms. | |
It is worth noting that in complex 2, where no water is present in the solid-state structure, the energy associated with both hydrogen bonds is −6.1 kcal mol−1. However, in complex 1, the cumulative energy of both hydrogen bonds is slightly more negative, measuring −6.6 kcal mol−1. This difference, albeit small (0.5 kcal mol−1), has a significant cumulative effect along the infinite polymeric chain. Consequently, it explains the distinct arrangement of the NH groups in these complexes.
Therefore, the pronounced influence of the lattice water molecule on the conformation of the polymeric chain can be attributed to the fact that the combination of NH⋯OAc and NH⋯OH2 hydrogen bonds is energetically stronger than the presence of two NH⋯OAc hydrogen bonds alone.
Hirshfeld surface analysis
Fig. S1 and S2 (ESI)† display the Hirshfeld surfaces of complexes 1 and 2, which are mapped over dnorm, curvedness, and shape index (range −0.1 Å to 1.5 Å). To enable visibility of the molecular moiety around which they are calculated, the surface is rendered as transparent. The dominant interactions are Br⋯H/H⋯Br and O⋯H/H⋯O of complex 2 can be seen in the Hirshfeld surface as red spots on the dnorm surface in Fig. S1.† Other visible spots in the Hirshfeld surfaces corresponds to H⋯H contacts. The small extent of area and light colour on the surface indicate weaker and longer contact other than hydrogen bonds. C⋯H/H⋯C interaction appear as distinct spikes in the 2D fingering plot (Fig. 11). Complementary regions are visible in the fingerprint plots where one molecule acts as a donar (de > di) and the other as an acceptor (de < di). The fingerprint plots can be decomposed to high-light contributions from different interactions types, which overlap in the full fingerprint.94
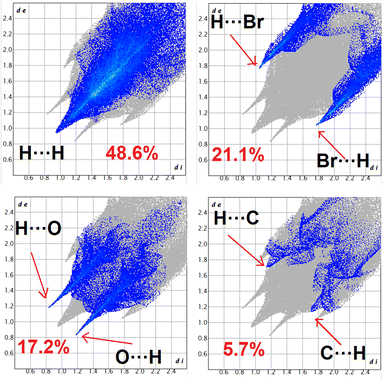 |
| Fig. 11 Fingerprint plot: different contacts contributed to the total Hirshfeld surface area of complex 2. | |
The proportions Br⋯H/H⋯Br, O⋯H/H⋯O and C⋯H/H⋯C, comprises 21.1, 17.2 and 5.7% of the Hirshfeld surface of the complex 2. Br⋯H/H⋯Br interaction appears as two distinct spike in the 2D fingerprint plots. The Br⋯H interaction is represented by lower spike (di = 1.78 and de = 1.03 Å) and the H⋯Br interaction is represented by the upper spike (di = 1.03 and de = 1.78 Å), which can be viewed as bright red spots on the dnorm surface. The O⋯H interaction is represented by lower spike (di = 1.18 and de = 0.83 Å) and the H⋯O interaction is represented by upper spike (di = 0.83 and de = 1.18 Å), which can be viewed as bright red spots on the dnorm surface. The C⋯H interaction is represented by lower spike (di = 1.70 and de = 1.15 Å) and the H⋯C interaction is represented by upper spike (di = 1.15 and de = 1.70 Å), which can be viewed as bright red spots on the dnorm surface.
IR spectral study
In the IR spectra of complexes 1 and 2, distinct bands in the region 3304–3152 are observed due to the presence of N–H stretching vibrations.95–97 Bands in the region 2924–2863 cm−1 may be assigned as C–H stretching vibrations.53,98,99 The details of the IR bands are gathered in Table S4 (ESI).†
Potential application of the complexes
The complexes may have applications in different fields. Some important potential applications of the complexes are outlined below. Many zinc complexes have been used as photo-catalyst to degrade several oreganic dyes, e.g. methylene blue.100,101 The present complexes may therefore have the potential to be used in the photo-catalytic degradation of different organic dyes and may therefore be used in textile industry for waste management. Literature shows that zinc complexes may mimic several hydrolytic enzymes e.g. phosphatase etc.42,102,103 Therefore, the present complexes may have interesting bio-mimetic catalytic application. Many group 12 metal complexes with similar ligands have been utilised to fabricate different opto-electronic devices, some of which have been found to show photo-sensitivity.17,104 It will therefore be interesting to explore the possibility of fabricating different opto-electronic devices using complexes 1 and 2. The strong fluorescence emission of many zinc complexes have been exploited for the detection of nitro-aromatic explosives by turn off fluorescence responses.39,105–107 The present complexes may also be used as sensor to detect different nitroaromatic explosives.
Conclusions
In summary, we have synthesized two zinc complexes. Both are forming 1D chains. We have estimated the energy of H-bonding interaction in the solid state structures of the complexes. Our analysis highlights the crucial role of hydrogen bonding in shaping the structures of Zn(II)-based coordination polymers. Complex 1, with a lattice water molecule, shows slightly stronger combined hydrogen bond energy than complex 2, lacking water. This modest energy difference significantly influences the orientation of NH groups and the polymer's arrangement. Although the application of the complexes were not explored in the present study, a literature survey on zinc complexes with similar Schiff bases or their reduced analogues indicates that the complexes may have the potential application in detecting nitroarmatic explosives, in the fabrication of opto-electronic devices, in the photo-catalytic degradation of organic dyes and also in mimicking different hydrolytic enzymes etc.
Conflicts of interest
There are no conflicts to declare.
Acknowledgements
P. Middya thanks the UGC, India, for awarding a Senior Research Fellowship (NFSC). This research was funded by MICIU/AEI of Spain (project PID2020-115637GB-I00 FEDER funds). We thank the “centre de technologies de la informació” (CTI) at the University of the Balearic Islands for computational facilities.
Notes and references
- R. Ziessel, Coord. Chem. Rev., 2001, 216, 195 CrossRef.
- P. G. Cozzi, Chem. Soc. Rev., 2004, 33, 410 RSC.
- K. C. Gupta and A. K. Sutar, Coord. Chem. Rev., 2008, 252, 1420 CrossRef CAS.
- J. P. Costes, S. Shova and W. Wernsdorfer, Dalton Trans., 2008, 1843 RSC.
- X. G. Ran, L. Y. Wang, Y. C. Lin, J. Hao and D. R. Cao, Appl. Organomet. Chem., 2010, 24, 741 CrossRef CAS.
- M. Orio, O. Jarjayes, H. Kanso, C. Philouze, F. Neese and F. Thomas, Angew. Chem., Int. Ed., 2010, 49, 4989 CrossRef CAS PubMed.
- X. Liu and J.-R. Hamon, Coord. Chem. Rev., 2019, 389, 94–118 CrossRef CAS.
- N. Cao, R. Jiang, L. Hao, L. Tian, R. Moc, Y. Fan, J. Zhao and L. Ren, Mater. Lett., 2019, 250, 182–185 CrossRef CAS.
- S.-H. Lee, S.-R. Shin and D.-S. Lee, Mater. Des., 2019, 172, 107774 CrossRef CAS.
- A. Panja and K. Ghosh, Mater. Chem. Front., 2018, 2, 1866–1875 RSC.
- S. Kr. Saha and P. Banerjee, Mater. Chem. Front., 2018, 2, 1674–1691 RSC.
- N. Ahmed, C. Das, S. Vaidya, A. K. Srivastava, S. K. Langley, K. S. Murray and M. Shanmugam, Dalton Trans., 2014, 43, 17375–17384 RSC.
- X. Yang, D. Schipper, R. A. Jones, L. A. Lytwak, B. J. Holliday and S. Huang, J. Am. Chem. Soc., 2013, 135, 8468–8471 CrossRef CAS PubMed.
- S. Sarkar and K. A. Dey, Spectrochim. Acta, Part A, 2010, 77, 740–748 CrossRef PubMed.
- A. Kösea, Ö. Güngör, J. N. Ballı and S. Erkan, J. Mol. Struct., 2022, 1268, 133750 CrossRef.
- S. Celedón, T. Roisnel, V. Artigas, M. Fuentealba, D. Carrillo, I. Ledoux-Rak, J.-R. Hamon and C. Manzur, New J. Chem., 2020, 44, 9190–9201 RSC.
- S. Roy, A. Dey, P. P. Ray, J. Ortega-Castro, A. Frontera and S. Chattopadhyay, Chem. Commun., 2015, 51, 12974–12976 RSC.
- S. Khan, S. Halder, P. P. Ray, S. Herrero, R. González-Prieto, M. G. B. Drew and S. Chattopadhyay, Cryst. Growth Des., 2018, 18, 651–659 CrossRef CAS.
- P. Bhowmik, H. P. Nayek, M. Corbella, N. Aliaga-Alcalde and S. Chattopadhyay, Dalton Trans., 2011, 40, 7916–7926 RSC.
- S. Naiya, S. Biswas, M. G. B. Drew, C. J. Gómez-García and A. Ghosh, Inorg. Chem., 2012, 51, 5332–5341 CrossRef CAS PubMed.
- P. Kar, P. M. Guha, M. G. B. Drew, T. Ishida and A. Ghosh, Eur. J. Inorg. Chem., 2011, 2075–2085 CrossRef CAS.
- B. Sarkar, M. S. Ray, Y.-Z. Li, Y. Song, A. Figuerola, E. Ruiz, J. Cirera, J. Cano and A. Ghosh, Chem.–Eur. J., 2007, 13, 9297–9309 CrossRef CAS PubMed.
- S. Chattopadhyay, M. G. B. Drew, C. Diaz and A. Ghosh, Dalton Trans., 2007, 2492–2494 RSC.
- S. Shaw and J. D. White, Chem. Rev., 2019, 119, 9381–9426 CrossRef CAS PubMed.
- B. Agrahari, S. Layek, R. Ganguly and D. D. Pathak, New J. Chem., 2018, 42, 13754–13762 RSC.
- A. Finelli, N. Hérault, A. Crochet and K. M. Fromm, Cryst. Growth Des., 2018, 18, 1215–1226 CrossRef CAS.
- S. I. Samponi, V. Zdorichenko, M. Danopoulou, M. C. Leech, K. Lam, A. Abdul-Sada, B. Cox, G. J. Tizzard, S. J. Coles, A. Tsipis and G. E. Kostakis, Dalton Trans., 2020, 49, 289–299 RSC.
- A. Singh, A. Maji, A. Mohanty and K. Ghosh, New J. Chem., 2020, 44, 18399–18418 RSC.
- A. Bhunia, P. W. Roesky, Y. Lan, G. E. Kostakis and A. K. Powell, Inorg. Chem., 2009, 48, 10483–10485 CrossRef CAS PubMed.
- N. Mahlooji, M. Behzad, H. A. Rudbari, G. Bruno and B. Ghanbari, Inorg. Chim. Acta, 2016, 445, 124–128 CrossRef CAS.
- L. S. Felices, E. C. Escudero-Adán, J. Benet-Buchholz and A. W. Kleij, Inorg. Chem., 2009, 48, 846–853 CrossRef PubMed.
- P. Asadollahi, R. Bikas, M. S. Krawczyk and T. Lis, Polyhedron, 2022, 211, 115537 CrossRef CAS.
- S. Roy, T. Dutta, M. G. B. Drew and S. Chattopadhyay, Polyhedron, 2020, 178, 114311 CrossRef CAS.
- P. Liu, X.-J. Feng and R. He, Tetrahedron, 2010, 66, 631–636 CrossRef CAS.
- M. J. Romero, R. Pedrido, A. M. González-Noya, M. Maneiro, M. I. Fernandez-García, G. Zaragoza and M. R. Bermejo, Dalton Trans., 2012, 41, 10832–10844 RSC.
- L. Rigamonti, A. Forni, M. Sironi, A. Ponti, A. M. Ferretti, C. Baschieri and A. Pasini, Polyhedron, 2018, 145, 22–34 CrossRef CAS.
- P. Bhowmik, S. Jana, P. Mahapatra, S. Giri, S. Chattopadhyay and A. Ghosh, Polyhedron, 2018, 145, 43–52 CrossRef CAS.
- P. Pandey, A. Verma, K. Bretosh, J.-P. Sutter and S. S. Sunkari, Polyhedron, 2019, 164, 80–89 CrossRef CAS.
- M. Karmakar, S. Roy and S. Chattopadhyay, New J. Chem., 2019, 43, 10093–10102 RSC.
- A. Biswas, M. G. B. Drew, C. J. Gomez-Garcia and A. Ghosh, Inorg. Chem., 2010, 49, 8155–8163 CrossRef CAS PubMed.
- A. Biswas, L. K. Das, M. G. B. Drew, C. Diaz and A. Ghosh, Inorg. Chem., 2012, 51, 10111–10121 CrossRef CAS PubMed.
- M. Karmakar, T. Basak and S. Chattopadhyay, New J. Chem., 2019, 43, 4432–4443 RSC.
- C.-T. Yang, M. Vetrichelvan, X. Yang, B. Moubaraki, K. S. Murray and J. J. Vittal, Dalton Trans., 2004, 113–121 RSC.
- A. Banerjee, S. Banerjee, C. J. Gomez Garc, S. Benmansour and S. Chattopadhyay, ACS Omega, 2019, 4, 20634–20643 CrossRef CAS PubMed.
- G. Consiglio, S. Failla, P. Finocchiaro, I. P. Oliveri, R. Purrello and S. D. Bella, Inorg. Chem., 2010, 49, 5134–5142 CrossRef CAS PubMed.
- S. Banerjee, P.-G. Lassahn, C. Janiak and A. Ghosh, Polyhedron, 2005, 24, 2963–2971 CrossRef CAS.
- P. J. Stang and B. Olenyuk, Acc. Chem. Res., 1997, 30, 502–518 CrossRef CAS.
- S. Horiuchi, R. Kumai and Y. Tokura, J. Am. Chem. Soc., 2005, 127, 5010–5011 CrossRef CAS PubMed.
- S. Roy, M. G. B. Drew, A. Frontera and S. Chattopadhyay, ChemistrySelect, 2017, 2, 7880–7887 CrossRef CAS.
- M. N. Ahamad, K. Iman, M. K. Raza, M. Kumar, A. Ansari, M. Ahmad and M. Shahid, Bioorg. Chem., 2020, 95, 103561 CrossRef PubMed.
- P. K. Bhaumik, A. Frontera and S. Chattopadhyay, Inorg. Chim. Acta, 2021, 515, 120023–120030 CrossRef CAS.
- S. Thakur, M. G. B. Drew, A. Franconetti, A. Frontera and S. Chattopadhyay, RSC Adv., 2019, 9, 35165–35175 RSC.
- M. Karmakar, A. Frontera and S. Chattopadhyay, CrystEngComm, 2021, 23, 1918–1928 RSC.
- G. R. Desiraju, Acc. Chem. Res., 2002, 35, 565–573 CrossRef CAS PubMed.
- L. Pauling, The Nature of the Chemical Bond, Cornell University Press, Ithaca, New York, 1939 Search PubMed.
- E. Andris, M. Straka, J. Vrána, A. Růžička, J. Roithová and L. Rulíšek, Chem.–Eur. J., 2023, 29, e202203769 CrossRef CAS PubMed.
- A. W. Schaefer, M. A. Ehudin, D. A. Quist, J. A. Tang, K. D. Karlin and E. I. Solomon, J. Am. Chem. Soc., 2019, 141, 4936–4951 CrossRef CAS PubMed.
- R. Gaur, S. Roy, P. Kallem and F. Banat, J. Mol. Struct., 2022, 1265, 133400 CrossRef CAS.
- M. A. Ehudin, A. W. Schaefer, S. M. Adam, D. A. Quist, D. E. Diaz, J. A. Tang, E. I. Solomon and K. D. Karlin, Chem. Sci., 2019, 10, 2893–2905 RSC.
- C. Bravo, F. Galego and V. André, CrystEngComm, 2019, 21, 7199–7203 RSC.
- D. Li and K. Kaneko, Chem. Phys. Lett., 2001, 335, 50–56 CrossRef CAS.
- J. E. Caton Jr and C. V. Banks, Inorg. Chem., 1967, 9, 1670–1675 CrossRef.
- S. D. Kurbah and N. S. Clovis, Inorg. Chim. Acta, 2020, 511, 119837 CrossRef CAS.
- S. Bosch, P. Comba, L. R. Gahan and G. Schenk, Inorg. Chem., 2014, 53, 9036–9051 CrossRef CAS PubMed.
- J. C. Mareque-Rivas, R. Prabaharan and R. T. M. de Rosales, Chem. Commun., 2004, 76–77 RSC.
- H. Duan, W. Dan and X. Fang, J. Solid State Chem., 2018, 260, 159–164 CrossRef CAS.
- M. Karmakar, W. Sk, R. M. Gomiila, M. G. B. Drew, A. Frontera and S. Chattopadhyay, RSC Adv., 2023, 13, 21211–21224 RSC.
- M. A. Kinzhalov, D. M. Ivanov, A. V. Shishkina, A. A. Melekhova, V. V. Suslonov, A. Frontera, V. Y. Kukushkin and N. A. Bokach, Inorg. Chem. Front., 2023, 10, 1522–1533 RSC.
- C. E. MacBeth, R. Gupta, K. R. Mitchell-Koch, V. G. Young Jr, G. H. Lushigtobn, W. H. Thompson, M. P. Hendrich and A. S Borovik, J. Am. Chem. Soc., 2004, 126, 2556–2567 CrossRef CAS PubMed.
- C. S. Hong, J. H. Yoon and Y. S. You, Inorg. Chim. Acta, 2005, 358, 3341–3346 CrossRef CAS.
- A. Mondal, A. Sarkar, A. Adhikary, D. Samanta and D. Das, Dalton Trans., 2020, 49, 15461–15472 RSC.
- J. M. Payne, M. Kamran, M. G. Davidson and M. D. Jones, ChemSusChem, 2022, 15, e202200225 Search PubMed.
- G. M. Sheldrick, Acta Crystallogr. Sect., 2015, 71, 3–8 CrossRef PubMed.
- G. M. Sheldrick, SADABS, V2014/5, Software for Empirical Absorption Correction, University of Göttingen, Gottingen, Germany, 1999 Search PubMed.
- M. J. Frisch, G. W. Trucks, H. B. Schlegel, G. E. Scuseria, M. A. Robb, J. R. Cheeseman, G. Scalmani, V. Barone, G. A. Petersson, H. Nakatsuji, X. Li, M. Caricato, A. V. Marenich, J. Bloino, B. G. Janesko, R. Gomperts, B. Mennucci, H. P. Hratchian, J. V. Ortiz, A. F. Izmaylov, J. L. Sonnenberg, D. Wil-liams-Young, F. Ding, F. Lipparini, F. Egidi, J. Goings, B. Peng, A. Petrone, T. Henderson, D. Ranasinghe, V. G. Zakrzewski, J. Gao, N. Rega, G. Zheng, W. Liang, M. Hada, M. Ehara, K. Toyota, R. Fukuda, J. Hasegawa, M. Ishida, T. Nakajima, Y. Honda, O. Kitao, H. Nakai, T. Vreven, K. Throssell, J. A. Montgomery Jr, J. E. Peralta, F. Ogliaro, M. J. Bearpark, J. J. Heyd, E. N. Brothers, K. N. Kudin, V. N. Staroverov, T. A. Keith, R. Kobayashi, J. Normand, K. Raghavachari, A. P. Rendell, J. C. Burant, S. S. Iyengar, J. Tomasi, M. Cossi, J. M. Millam, M. Klene, C. Adamo, R. Cammi, J. W. Ochterski, R. L. Martin, K. Morokuma, O. Farkas, J. B. Foresman, and D. J. Fox, Gaussian 16, Revision C.01, Gaussian, Inc., Wallingford CT, 2016 Search PubMed.
- C. Adamo and V. Barone, J. Chem. Phys., 1999, 110, 6158–6170 CrossRef CAS.
- S. Grimme, J. Antony, S. Ehrlich and H. Krieg, J. Chem. Phys., 2010, 132, 154104–154118 CrossRef PubMed.
- F. Weigend, Phys. Chem. Chem. Phys., 2006, 8, 1057–1065 RSC.
- R. F. W. Bader, Chem. Rev., 1991, 91(5), 893–928 CrossRef CAS.
- R. F. W. Bader, J. Phys. Chem. A, 1998, 102, 7314–7323 CrossRef CAS.
- T. A. Keith, TK Gristmill Software, AIMAll
(Version 19.10.12), Overland Park KS, USA, 2019, https://aim.tkgristmill.com/ Search PubMed.
- E. Espinosa, E. Molins and C. Lecomte, Chem. Phys. Lett., 1998, 285, 170–173 CrossRef CAS.
- A. Banerjee, A. Frontera and S. Chattopadhyay, Dalton Trans., 2019, 48, 11433–11447 RSC.
- S. Roy, A. Dey, M. G. B. Drew, P. P. Ray and S. Chattopadhyay, New J. Chem., 2019, 43, 5020–5031 RSC.
- M. Das, S. Chatterjee, K. Harm, T. K. Mondal and S. Chattopadhyay, Dalton Trans., 2014, 43, 2936–2947 RSC.
- N. Sarkar, M. G. B. Drew, K. Harms, A. Bauza, A. Frontera and S. Chattopadhyay, CrystEngComm, 2018, 20, 1077–1086 RSC.
- A. Bannerjee, D. Das, P. P. Ray, S. Banerjee and S. Chattopadhyay, Dalton Trans., 2021, 50, 1721 RSC.
- S. Thakur, S. Banerjee, S. Das and S. Chattopadhyay, New J. Chem., 2019, 43, 18747–18759 RSC.
- S. Khan, S. Sproules, L. S. Natrajan, K. Harms and S. Chattopadhyay, New J. Chem., 2018, 42, 1634–1641 RSC.
- I. Mondal, T. Basak, S. Banerjee and S. Chattopadhyay, CrystEngComm, 2020, 22, 3005–3019 RSC.
- L. Yang, D. R. Powell and R. P. Houser, Dalton Trans., 2007, 955–964 RSC.
- D. Cremer and J. A. Pople, J. Am. Chem. Soc., 1975, 97, 1354–1358 CrossRef CAS.
- D. Cremer, Acta Crystallogr., 1984, 40, 498–500 CrossRef.
- M. A. Spackman and P. G. Byrom, Chem. Phys. Lett., 1997, 267, 215–220 CrossRef CAS.
- S. Chattopadhyay, M. S. Ray, S. Chaudhuri, G. Mukhopadhyay, G. Bocelli, A. Cantoni and A. Ghosh, Inorg. Chim. Acta, 2006, 359, 1367–1375 CrossRef CAS.
- A. Hazari, R. M. Gomila, A. Frontera, M. G. B. Drew and A. Ghosh, CrystEngComm, 2021, 23, 4848–4856 RSC.
- D. Pellico, M. Gomez-Gallego, R. Escudero, P. Ramirez-Lopez, M. Olivan and M. A. Sierra, Dalton Trans., 2011, 40, 9145–9153 RSC.
- K. Ghosh, S. Roy, A. Ghosh, A. Banerjee, A. Bauzá, A. Frontera and S. Chattopadhyay, Polyhedron, 2006, 112, 6–17 CrossRef.
- A. K. Sharma, F. Lloret and R. Mukherjee, Inorg. Chem., 2013, 52, 4825–4833 CrossRef CAS PubMed.
- T. Basak, A. Bhattacharyya, K. Harms and S. Chattopadhyay, Polyhedron, 2019, 157, 449–457 CrossRef CAS.
- M. Karmakar and S. Chattopadhyay, Polyhedron, 2020, 184, 114527 CrossRef CAS.
- T. Basak, M. G. B. Drew and S. Chattopadhyay, Inorg. Chem. Commun., 2018, 98, 92–98 CrossRef CAS.
- T. Basak, A. Bhattacharyya, M. Das, K. Harms, A. Bauzá, A. Frontera and S. Chattopadhyay, ChemistrySelect, 2017, 2, 6286–6295 CrossRef CAS.
- S. Roy, A. Dey, R. M. Gomila, J. Ortega-Castro, A. Frontera, P. P. Ray and S. Chattopadhyay, Dalton Trans., 2022, 51, 5721–5734 RSC.
- T. Basak, S. Roy, S. Banerjee and S. Chattopadhyay, Inorg. Chim. Acta, 2022, 543, 121186 CrossRef CAS.
- I. Mondal, S. Chatterjee and S. Chattopadhyay, Polyhedron, 2020, 190, 114735 CrossRef CAS.
- M. Karmakar and S. Chattopadhyay, Polyhedron, 2020, 187, 114639 CrossRef CAS.
Footnote |
† Electronic supplementary information (ESI) available: Fig. S1–S7 and Table S1–S4. CCDC 2323492 and 2323493 for complexes 1 and 2 respectively. For ESI and crystallographic data in CIF or other electronic format see DOI: https://doi.org/10.1039/d4ra00550c |
|
This journal is © The Royal Society of Chemistry 2024 |